DOI:
10.1039/D3SC02604C
(Edge Article)
Chem. Sci., 2023,
14, 10795-10799
Rare-earth hydroxide/MXene hybrid: a promising agent for near-infrared photothermy and magnetic resonance imaging†
Received
24th May 2023
, Accepted 19th September 2023
First published on 20th September 2023
Abstract
Layered gadolinium hydroxide (LGdH) and Ti3C2 monolayers were assembled into a LGdH/Ti3C2 (GTC) hybrid. The hybrid demonstrated enhanced near-infrared (NIR) light absorption properties and superior photothermal performance. Moreover, the GTC hybrid achieved an excellent T1-weighted magnetic resonance imaging (MRI) effect.
Introduction
Titanium carbide (Ti3C2), the first reported MXene, is synthesized by selective etching of the parent MAX phase of layered Ti3AlC2, which has attracted tremendous attention for various applications including energy storage and conversion, drug delivery and environmental remediation.1–6 Recent reports show that MXenes with excellent biocompatibility and low toxicity are promising materials in biochemistry fields, such as immunomodulation and tissue reconstruction,7,8 3D bioprinting,9,10 bioimaging,11 biosensing,11 and photothermal therapy.12–14 The etching process involves the surface modification of MXenes with functional groups –OH, –F,
O, or –Al(OH)4, resulting in enhanced photothermal properties.15,16 But the photothermal performance of Ti3C2 in the NIR window is still limited and further improvement is required.17,18 Therefore, it is of great significance to modify Ti3C2 MXene with other functional materials, such as 2D nanosheets,19,20 to elevate its photothermal performance as well as expand its multi-functionality.
Layered rare-earth hydroxides (LREHs), a new important family of anion-exchangeable layered compounds, show promising applications in the fields of magnetism21–23 and photoluminescence24,25 due to special 4f electronic structures. In recent years, more and more research interest has been invested in exploring the biochemical applications of rare earth-containing materials.26–28 However, biochemical research is mainly focused on rare-earth element-doped nanomaterials or quantum dots.27,29,30 Exploration and hybridization of 2D LREHs with other nanosheets are still blank. Recently, the successful exfoliation of LREHs into monolayer nanosheets has greatly expanded their application prospects as building blocks in nanofilms and nanodevices.31,32 For example, through layer-by-layer assembly, superlattice structured films constructed from LREH nanosheets and semiconducting oxide nanosheets exhibited enhanced photoluminescence through efficient energy transfer across the nanointerface.32,33 Among rare-earth elements, gadolinium and gadolinium-based compounds are widely used as photoluminescent hosts or matrices due to low phonon energy, suitability for coupling with other rare-earth ions, and low cost. Moreover, gadolinium compounds with the half-filled 4f orbitals of Gd3+, exhibit excellent magnetism properties making them ideal for use as magnetic resonance imaging (MRI) and computed tomography (CT) contrast agents.34–37 Therefore, a layered gadolinium hydroxide (LGdH) nanosheet is selected to pair with a Ti3C2 nanosheet for enhanced photothermal and MRI properties.
In this work, unilaminar LGdH and Ti3C2 nanosheets were face-to-face restacked into a LGdH/Ti3C2 (GTC) hybrid, which exhibited enhanced NIR light absorption and MRI performance. This approach not only broadens the application of both MXenes and LREHs nanosheets toward biotherapy, but also provides a general strategy to design photothermal therapy and MRI contrast agent materials based on 2D nanomaterials.
Results and discussion
Scanning electron microscopy (SEM) images of Ti3AlC2 before and after etching are shown in Fig. S1a and b.† Raw Ti3AlC2 swelled and split obviously after etching. According to inductively coupled plasma-optical emission spectrometry (ICP-OES) analysis, the Al/Ti atomic ratio was estimated to be 0.007
:
3 after etching, verifying an almost complete removal of the Al atoms. Fig. S1c† shows the X-ray diffraction (XRD) profile of the raw Ti3AlC2 particles. The pattern matched well with the standard JCPDS card of the hexagonal Ti3AlC2 phase (No. 52-0875), indicating a high crystallinity and phase purity of the pristine crystals. After etching, the 00l peaks slightly shifted to a lower degree, corresponding to an expanded interlayer distance from 1.84 nm to 2.05 nm. In addition, the broader full width at half maximum (FWHM) implied a decreased layer stacking order.
Fig. 1a and b show the atomic force microscopy (AFM) image and thickness profile of exfoliated Ti3C2 nanosheets, respectively. They were characterized by a typical thickness of approximately 1.5 nm, which is larger than a crystallographic unilamellar thickness of 0.75 nm,16 likely due to the adsorption of functional groups/ions (such as –OH and –F) and water molecules on the surface. Similarly, Fig. 1c and d show the AFM image and typical thickness of LGdH nanosheets, separately. The thickness was estimated to be approximately 1.0 nm, which is consistent with our previous report,31 indicating a monolayer feature. The lateral dimensions of both nanosheets fall in a range from several hundred nanometres to a few micrometres. The inset photographs of the suspensions in Fig. 1a and c display a homogeneous and stable dispersion state of the exfoliated nanosheets.
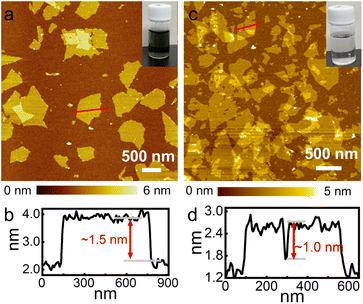 |
| Fig. 1 AFM images of (a) Ti3C2 and (c) LGdH nanosheets. Corresponding height profiles of (b) Ti3C2 and (d) LGdH nanosheets along the marked lines. Insets of (a) and (c) show the photographs of the nanosheet suspension. | |
A black flocculated product was induced immediately with the dropwise addition of Ti3C2 nanosheets into the LGdH nanosheet suspension. As the Ti3C2 nanosheets held a negative charge with a zeta potential of −34.6 mV while their LGdH counterparts were positively charged with a zeta potential of +57.3 mV, they underwent spontaneous restacking driven by the electrostatic attraction (see Fig. S2† for details). Fig. S3a† depicts the XRD pattern of the flocculated product GTC. Both the characteristic in-plane diffraction peaks of LGdH and Ti3C2 were observed, confirming the intact feature of two oppositely charged nanosheets during the hybridizing process. Fig. 2a shows the transmission electron microscopy (TEM) image of the resultant GTC hybrid, in which lamellar morphology was observed. The rings in the selected area electron diffraction (SAED) pattern are indexed to be in-plane reflections of Ti3C2 nanosheets and LGdH nanosheets, respectively (Fig. 2b). The elemental mapping in Fig. 2c indicates a uniform distribution and intimate mixing of the two nanosheets in the GTC hybrid. The size of the GTC hybrid ranged from 50 to 500 nm according to the dynamic light scattering (DLS) results in Fig. S3b.† The smaller size of the flocculated GTC is due to the bending and wrinkles of the nanosheets during the flocculation process. It is noteworthy that flocculated GTC hybrid can be well re-dispersed in deionized water, as evidenced by the inset of Fig. 2a. The small size and uniform dispersion features of the flocculated product made it possible to be used in biomedicine fields such as MRI and photothermal therapy.
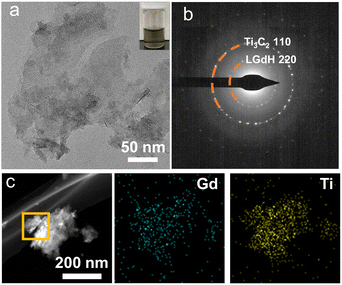 |
| Fig. 2 (a) TEM, (b) SAED pattern, and (c) elemental mapping images of the GTC hybrid. The inset of (a) shows the corresponding suspension. | |
Fig. 3 compares UV-vis absorbance of Ti3C2 nanosheets and the GTC hybrid at various concentrations. For the convenience of comparison, all the concentrations were calibrated using the quantity of Ti3C2 in the suspensions. The concentrations of all samples in Fig. 3 are tabulated in Table S1.† As shown in Fig. 3a, for pure Ti3C2 nanosheet suspensions, the strong and broad absorption band ranging from 230 to 500 nm was attributed to the band-gap energy of the oxidized MXene.38 In addition, the weak broad absorption band centred at 715 nm in the NIR range from 600 to 830 nm was due to the surface plasmon resonance (SPR) caused by the inter-band transitions in MXene nanosheets.39 As an important parameter to evaluate the efficiency of light absorption, the mass extinction coefficient ε at 808 nm can be calculated according to the Lambert–Beer law:
where
A is the absorbance,
ε represents the absorption coefficient,
c (g L
−1) stands for the concentration of the samples and
l (cm) is the absorption path length. Based on the plot shown in
Fig. 3b, the
ε of Ti
3C
2 nanosheets was calculated to be 38.9 L g⁻
1 cm⁻
1. This value was higher than those reported in the previous literature (Table S2
†).
16,40 In the current work, the Al atomic layer in the Ti
3AlC
2 parent compound was selectively etched using LiF/HCl solvent. Some residual Li
+ ions adsorbed on the surface of the Ti
3C
2 nanosheets,
41 which was confirmed by the ICP result yielding a molar ratio of Ti
![[thin space (1/6-em)]](https://www.rsc.org/images/entities/char_2009.gif)
:
![[thin space (1/6-em)]](https://www.rsc.org/images/entities/char_2009.gif)
Al
![[thin space (1/6-em)]](https://www.rsc.org/images/entities/char_2009.gif)
:
![[thin space (1/6-em)]](https://www.rsc.org/images/entities/char_2009.gif)
Li at 94.28
![[thin space (1/6-em)]](https://www.rsc.org/images/entities/char_2009.gif)
:
![[thin space (1/6-em)]](https://www.rsc.org/images/entities/char_2009.gif)
0.23
![[thin space (1/6-em)]](https://www.rsc.org/images/entities/char_2009.gif)
:
![[thin space (1/6-em)]](https://www.rsc.org/images/entities/char_2009.gif)
5.49. Similar to Al or Au ion modified Ti
3C
2 MXene, the adsorption of Li
+ ions may enhance the local surface plasmon resonance (LSPR) effect,
18,40 resulting in an enhancement in NIR light absorption properties. As shown in
Fig. 3c, for the GTC hybrid, the broad band in the NIR region red-shifted to 780 nm and the absorption was extended to a wider wavelength range from 600 to 1000 nm. Compared to 38.9 L g⁻
1 cm⁻
1 for Ti
3C
2 nanosheets, the
ε value of the GTC hybrid increased to 50.0 L g⁻
1 cm⁻
1, the highest value among those of the reported NIR photothermal materials (Table S2
†). In contrast, the same amount of pure LGdH nanosheets showed negligible absorption in the NIR region (Fig. S4
†). The red-shift absorption and enhanced mass extinction coefficient of the GTC hybrid are believed to be a synergistic effect of hybridizing LGdH and Ti
3C
2 nanosheets on a molecular scale. The outstanding NIR absorption properties of the GTC hybrid promise their high photothermal performance.
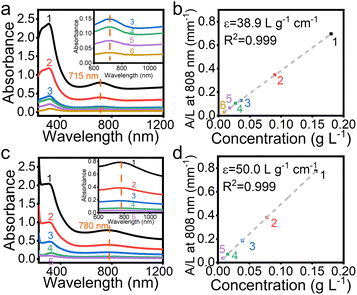 |
| Fig. 3 UV-vis absorption of (a) Ti3C2 nanosheet suspensions and (c) GTC hybrid suspensions. The Lambert–Beer law absorbance plot for absorption at 808 nm of (b) Ti3C2 nanosheet suspensions and (d) GTC hybrid suspensions. The numbers 1–6 stand for different concentrations of each suspension. | |
The photothermal properties were evaluated under NIR laser exposure with a laser intensity of 2 W cm⁻2. The concentration of a pure Ti3C2 suspension and LGdH suspension was 15.6 mg mL⁻1. While for the GTC suspension, the contained Ti3C2 component was 15.6 mg mL⁻1. As indicated by the IR thermographic images in Fig. S5,† the temperature of the GTC hybrid increased with an increasing concentration. Under 2 W cm⁻2 irradiation for 10 min, the temperature surpassed 60.4 °C when the concentration was higher than 7.8 mg mL⁻1. For instance, for an elevated concentration of 15.6 mg mL⁻1, the temperature of GTC drastically rose to 61.2 °C after a short exposure of only 3 min, and finally reached 67.2 °C after 10 min. In comparison, the temperature reached only 57.1 °C and 27.7 °C for pure Ti3C2 and LGdH nanosheets, respectively, as presented in Fig. 4. The result was well consistent with that of the UV-vis absorption analysis, in which the ε value of the GTC hybrid was notably higher than that of Ti3C2 nanosheets, while the LGdH nanosheet exhibited negligible NIR absorption. The significant temperature growth induced by the GTC hybrid indicated a higher photothermal capability than that by using sole Ti3C2 or LGdH nanosheets.
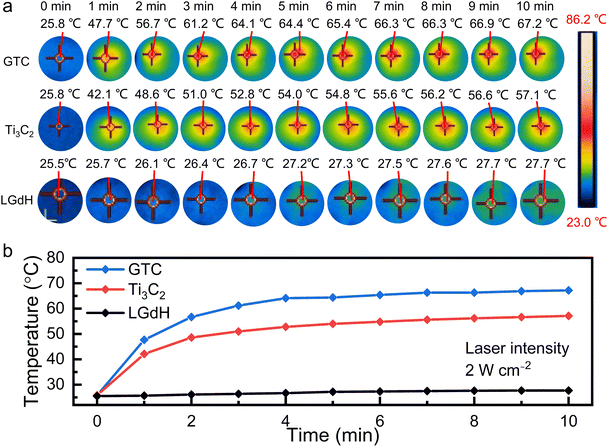 |
| Fig. 4 Photothermal properties. (a) IR thermographic images and (b) heating curves of the GTC hybrid, Ti3C2 nanosheets and LGdH nanosheets at a calibrated concentration of Ti3C2 of 15.6 mg mL⁻1 under NIR laser irradiation (808 nm, 2 W cm⁻2). | |
The performance of the GTC hybrid as a T1-weighted MRI contrast agent was evaluated by an in vitro assay experiment. As shown in Fig. 5a and b, the contrast effect was enhanced with the increasing concentration of the GTC hybrid, while no significant enhancement for individual Ti3C2 nanosheet and LGdH nanosheet suspensions was observed when compared with the control saline group. When the concentration reached 1.25 mg mL⁻1, the T1-weighted signal exhibited obvious brightness compared with that of the control group, comparable to the frontier contrast agents such as clinical gadoteric acid meglumine salt injection.42Fig. 5c depicts the in vivo contrast effect of both the GTC suspension (white arrow) and the control saline (grey arrow), from which a T1-weighted signal image was clearly presented. The significant enhancement in both in vitro and in vivo tests implied a promising application of the GTC hybrid as an MRI agent.
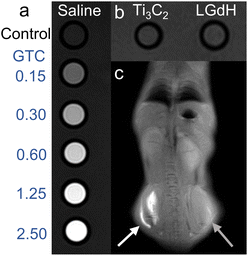 |
| Fig. 5
In vitro T1-weighted MRI effect of (a) the GTC hybrid at various concentrations (mg mL⁻1), and (b) individual Ti3C2 nanosheet and LGdH nanosheet suspensions at a concentration of 1.25 mg mL⁻1. (c) In vivo MRI of a nude mouse with injection of the GTC suspension (white arrow) and the control saline (grey arrow). | |
Conclusions
In conclusion, a GTC hybrid was fabricated by the assembly of oppositely charged Ti3C2 nanosheets and their LGdH counterparts. The hybrid showed a significantly improved mass absorption coefficient ε of 50.0 L g⁻1 cm⁻1 at 808 nm compared with 38.9 L g⁻1 cm⁻1 for Ti3C2 nanosheets. Under NIR laser (808 nm, 2 W cm⁻2) irradiation for 10 min, the temperature of the GTC hybrid could reach as high as 67.2 °C, demonstrating a higher photothermal capability than sole Ti3C2 and LGdH nanosheets. Moreover, the GTC hybrid showed an excellent T1-weighted MRI effect. This work may hold great potential for the design and development of high-performance photothermal therapy materials as well as MRI contrast agents through the molecular-level hybridization of fancy nanosheet building blocks.
Author contributions
M. B. and R. M. conceived and coordinated the project. X. L., H. W. and R. M. supervised the project. M. B. performed the experiments and analysed the data. L. S. assisted with the photothermal property measurement and analysis. J. D. assisted with the MRI measurement and data analysis. The manuscript was written through the contributions of all authors. All authors have given approval to the final version of the manuscript.
Conflicts of interest
The authors declare no competing financial interest.
Acknowledgements
The authors acknowledge the financial support from the National Natural Science Foundation of China (U20A20123) and the Innovative Research Group of the Hunan Provincial Natural Science Foundation of China (2019JJ10006). M. B. is thankful for the support from the Chongqing University of Technology (2023ZDZ026) and the Science and Technology Research Program of the Chongqing Municipal Education Commission (KJQN202301134). R. M. acknowledges the support from JSPS KAKENNHI (22H01916 and 22K18956). Prof. Guoping Chen from the National Institute for Materials Science, Tsukuba, Japan is gratefully acknowledged.
Notes and references
- M. Naguib, M. Kurtoglu, V. Presser, J. Lu, J. Niu, M. Heon, L. Hultman, Y. Gogotsi and M. W. Barsoum, Adv. Mater., 2011, 23, 4248–4253 CrossRef CAS PubMed.
- A. Levitt, J. Zhang, G. Dion, Y. Gogotsi and J. M. Razal, Adv. Funct. Mater., 2020, 30, 2000739 CrossRef CAS.
- L. Yu, Z. Fan, Y. Shao, Z. Tian, J. Sun and Z. Liu, Adv. Energy Mater., 2019, 9, 1901839 CrossRef.
- Q. Liu, A. Zhao, X. He, Q. Li, J. Sun, Z. Lei and Z. H. Liu, Adv. Funct. Mater., 2021, 31, 2010944 CrossRef CAS.
- J. Lei, F. Yu, H. Xie and J. Ma, Chem. Sci., 2023, 14, 3610–3621 RSC.
- A. E. Allah, J. Wang, Y. V. Kaneti, T. Li, A. A. Farghali, M. H. Khedr, A. K. Nanjundan, B. Ding, H. Dou and X. Zhang, Nano Energy, 2019, 65, 103991 CrossRef CAS.
- S. Pan, J. Yin, L. Yu, C. Zhang, Y. Zhu, Y. Gao and Y. Chen, Adv. Sci., 2020, 7, 1901511 CrossRef CAS PubMed.
- A. Rafieerad, W. Yan, G. L. Sequiera, N. Sareen, E. Abu-El-Rub, M. Moudgil and S. Dhingra, Adv. Healthcare Mater., 2019, 8, 1900569 CrossRef PubMed.
- H. Rastin, B. Zhang, A. Mazinani, K. Hassan, J. Bi, T. T. Tung and D. Losic, Nanoscale, 2020, 12, 16069–16080 RSC.
- M. Das, R. S. Ambekar, S. K. Panda, S. Chakraborty and C. S. Tiwary, J. Mater. Res., 2021, 36, 4024–4050 CrossRef CAS.
- M. Soleymaniha, M. A. Shahbazi, A. R. Rafieerad, A. Maleki and A. Amiri, Adv. Healthcare Mater., 2019, 8, 1801137 CrossRef PubMed.
- H. Lin, X. Wang, L. Yu, Y. Chen and J. Shi, Nano Lett., 2017, 17, 384–391 CrossRef CAS PubMed.
- R. Liang, Y. Li, M. Huo, H. Lin and Y. Chen, ACS Appl. Mater. Interfaces, 2019, 11, 42917–42931 CrossRef CAS PubMed.
- X. Fan, Y. Ding, Y. Liu, J. Liang and Y. Chen, ACS Nano, 2019, 13, 8124–8134 CrossRef CAS PubMed.
- G. Li, B. C. Wyatt, F. Song, C. Yu, Z. Wu, X. Xie, B. Anasori and N. Zhang, Adv. Funct. Mater., 2021, 21, 2105043 CrossRef.
- J. Xuan, Z. Wang, Y. Chen, D. Liang, L. Cheng, X. Yang, Z. Liu, R. Ma, T. Sasaki and F. Geng, Angew. Chem., Int. Ed., 2016, 55, 14569–14574 CrossRef CAS PubMed.
- D. Xu, Z. Li, L. Li and J. Wang, Adv. Funct. Mater., 2020, 30, 2000712 CrossRef CAS.
- Z. Yu, L. Jiang, R. Liu, W. Zhao, Z. Yang, J. Zhang and S. Jin, Chem. Eng. J., 2021, 426, 131914 CrossRef CAS.
- M. Eguchi, A. S. Nugraha, A. E. Rowan, J. Shapter and Y. Yamauchi, Adv. Sci., 2021, 8, 2100539 CrossRef CAS PubMed.
- W. Liu, Z. Wang, Y. Su, Q. Li, Z. Zhao and F. Geng, Adv. Energy Mater., 2017, 7, 1602834 CrossRef.
- Z. Yang, H. Zhang, M. Bai, W. Li, S. Huang, S. Ruan and Y. J. Zeng, J. Mater. Chem. C, 2020, 8, 11866–11873 RSC.
- L. Caretta, E. Rosenberg, F. Büttner, T. Fakhrul, P. Gargiani, M. Valvidares, Z. Chen, P. Reddy, D. A. Muller, C. A. Ross and G. S. D. Beach, Nat. Commun., 2020, 11, 1090 CrossRef CAS PubMed.
- H. Wang, J. Chen, T. Liu, J. Zhang, K. Baumgaertl, C. Guo, Y. Li, C. Liu, P. Che, S. Tu, S. Liu, P. Gao, X. Han, D. Yu, M. Wu, D. Grundler and H. Yu, Phys. Rev. Lett., 2020, 124, 027203 CrossRef CAS PubMed.
- Y. Liu, X. Rong, M. Li, M. S. Molokeev, J. Zhao and Z. Xia, Angew. Chem., Int. Ed., 2020, 59, 11634–11640 CrossRef CAS PubMed.
- Z. Zeng, Y. Xu, Z. Zhang, Z. Gao, M. Luo, Z. Yin, C. Zhang, J. Xu, B. Huang and F. Luo, Chem. Soc. Rev., 2020, 49, 1109–1143 RSC.
- Z. Liu, F. Ren, H. Zhang, Q. Yuan, Z. Jiang, H. Liu, Q. Sun and Z. Li, Biomaterials, 2019, 219, 119364 CrossRef CAS PubMed.
- L. Ruiyi, L. Zaijun, S. Xiulan, J. Jan, L. Lin, G. Zhiguo and W. Guangli, Chem. Eng. J., 2020, 382, 122992 CrossRef CAS.
- X. Shan, Q. Chen, X. Yin, C. Jiang, T. Li, S. Wei, X. Zhang, G. Sun, J. Liu and L. Lu, J. Mater. Chem. B, 2020, 8, 426–437 RSC.
- D.-K. Ji, G. Reina, H. Liang, D. Zhang, S. Guo, B. Ballesteros, C. Ménard-Moyon, J. Li and A. Bianco, ACS Appl. Nano Mater., 2021, 4, 1467–1477 CrossRef CAS.
- H. Li, R. Wei, G.-H. Yan, J. Sun, C. Li, H. Wang, L. Shi, J. A. Capobianco and L. Sun, ACS Appl. Mater. Interfaces, 2018, 10, 4910–4920 CrossRef CAS PubMed.
- M. Bai, X. Liu, N. Sakai, Y. Ebina, L. Jia, D. Tang, T. Sasaki and R. Ma, J. Phys. Chem. Lett., 2021, 12, 10135–10143 CrossRef CAS PubMed.
- M. Bai, X. Liu, T. Sasaki and R. Ma, Nanoscale, 2021, 13, 4551–4561 RSC.
- S. Ida, Y. Sonoda, K. Ikeue and Y. Matsumoto, Chem. Commun., 2010, 46, 877–879 RSC.
- X. Yu, X. Liu, W. Wu, K. Yang, R. Mao, F. Ahmad, X. Chen and W. Li, Angew. Chem., Int. Ed., 2019, 58, 2017–2022 CrossRef CAS PubMed.
- N. Rammohan, K. W. MacRenaris, L. K. Moore, G. Parigi, D. J. Mastarone, L. M. Manus, L. M. Lilley, A. T. Preslar, E. A. Waters, A. Filicko, C. Luchinat, D. Ho and T. J. Meade, Nano Lett., 2016, 16, 7551–7564 CrossRef CAS PubMed.
- L. M. Manus, D. J. Mastarone, E. A. Waters, X. Q. Zhang, E. A. Schultz Sikma, K. W. MacRenaris, D. Ho and T. J. Meade, Nano Lett., 2010, 10, 484–489 CrossRef CAS PubMed.
- H. Wan, L. Hu, X. Liu, Y. Zhang, G. Chen, N. Zhang and R. Ma, Chem. Sci., 2023, 14, 2776–2798 RSC.
- E. Satheeshkumar, T. Makaryan, A. Melikyan, H. Minassian, Y. Gogotsi and M. Yoshimura, Sci. Rep., 2016, 6, 32049 CrossRef CAS PubMed.
- S. Elumalai, J. R. Lombardi and M. Yoshimura, Adv. Mater., 2020, 1, 146–152 RSC.
- G. Liu, J. Zou, Q. Tang, X. Yang, Y. Zhang, Q. Zhang, W. Huang, P. Chen, J. Shao and X. Dong, ACS Appl. Mater. Interfaces, 2017, 9, 40077–40086 CrossRef CAS PubMed.
- F. Liu, A. Zhou, J. Chen, J. Jia, W. Zhou, L. Wang and Q. Hu, Appl. Surf. Sci., 2017, 416, 781–789 CrossRef CAS.
- J. Li, J. Duan, Z. He, Y. Liao, X. Liu, P. Rong, G. Chen, H. Wan, Y. Huang and R. Ma, Adv. Opt. Mater., 2023, 11, 2203146 CrossRef CAS.
|
This journal is © The Royal Society of Chemistry 2023 |