DOI:
10.1039/D3SC00877K
(Edge Article)
Chem. Sci., 2023,
14, 4605-4611
Formation and fine-tuning of metal–organic frameworks with carboxylic pincers for the recognition of a C2H2 tetramer and highly selective separation of C2H2/C2H4†
Received
16th February 2023
, Accepted 2nd April 2023
First published on 3rd April 2023
Abstract
Highly efficient ethylene (C2H4) and acetylene (C2H2) separation is a great challenge and an important process in current industries. Herein, we finely tune a new family of 6-c metal–organic frameworks (MOFs) with crab-like carboxylic pincers for the recognition of a C2H2 tetramer and afford NTU-72 with high adsorption C2H2/C2H4 selectivity (56–441, 298 K) as well as unprecedented recovery of both highly pure C2H4 (99.95%) and C2H2 (99.36%). Furthermore, the effective binding of a C2H2 tetramer by NTU-72's carboxylic pincers has been revealed by gas-loaded crystallography and Raman spectral studies. Our work provides a novel approach for the selective binding of a small molecular cluster for designing high-performance MOFs.
Introduction
As components of cracked gas, C2H4 and C2H2 are important raw materials for the synthesis of various organic compounds such as polyethylene, α-ethynyl alcohols, and acrylic acid derivatives.1–3 The preparation of these value-added products generally requires a high-purity feed gas. In industry, pure C2H4 is realized by solvent absorption or selective catalytic hydrogenation from cracked gas, which are energy consuming processes.4,5 Meanwhile, the recovery of high-purity C2H2 is generally ignored in this process.
Adsorptive separation using porous materials is more environmentally friendly and energy efficient, and is regarded as a promising future separation technology.6–11 As emerging porous materials, metal–organic frameworks (MOFs), derived from the self-assembly of organic ligands and inorganic nodes, demonstrate great opportunities to distinguish these two kinds of gas molecules by dedicated framework regulations, despite them having nearly identical molecular dimensions and physical properties.12–15 For example, the MOF materials SIFSIX-Cu-i7 and NKMOF-1-Ni16 exhibit minimal trade-off between adsorption capacity and selectivity, while the flexible UTSA-300 (ref. 17) demonstrates gating separation of these mixtures. However, they all produce lower purity C2H2 during desorption, even for the sieving materials, as both C2H2 and C2H4 molecules can interact with strong binding sites, or the more or less flexibility of the sieving frameworks allows a certain amount of co-adsorption.18,19
MOFs with free carboxylic acids might be potential light hydrocarbon separation materials due to their remarkable characteristics of being a hydrogen bonding acceptor (O–H) and donor (C
O).20,21 However, these MOFs have never been systematically investigated or intentionally designed for high-performance gas separation,22–24 due to the significant coordination activity of carboxylic acids in MOF construction. We are interested in finely tuning MOFs to achieve efficient gas separations.25–28 Herein, evolved from the interpenetrated dia topology,29 a new family of 6-c MOFs (termed NTU-71 to NTU-73) with carboxylic pincers were designed based upon a C2v symmetric ligand of 3,5-di(1H-imidazol-1-yl)benzoic acid (HL) and the hybrid ions with different radii (SiF62− (dSi–F: 1.690 Å), TiF62− (dTi–F: 1.886 Å) and ZrF62− (dZr–F: 1.983 Å)). Among them, the distance of their carboxylic pincers has been finely tailored, and thus, they may approach those found in crab-like systems (Scheme 1). Very interestingly, NTU-72 exhibits a high C2H2/C2H4 selectivity and great ability for recovery of both highly pure C2H4 (99.95%) and C2H2 (99.36%). This excellent separation performance is due to the stabilization of a C2H2 tetramer by a couple of carboxylic pincers in the optimized pore geometry and size in NTU-72.
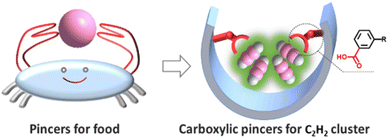 |
| Scheme 1 Crab-inspired MOF design incorporating carboxylic pincers for selective recognition of a C2H2 cluster. | |
Experimental section
General procedures of the experiment and simulation are available in the ESI.†
Synthesis of NTU-70 to NTU-73
Synthesis of NTU-71.
HL (4 mg, 0.015 mmol), CuSiF6 (10 mg, 0.04 mmol) and H2SiF6 (100 μL) were mixed in DMA(dimethylacetamide)/H2O/EtOH (1
:
1
:
1, 3 mL) in a 10 mL glass container and tightly capped with a Teflon vial and heated at 95 °C for 48 h. After cooling to room temperature, the resulting blue-green crystals were harvested and washed with fresh DMA three times. Yield is ∼49% (based on the ligand).
Synthesis of NTU-72.
HL (4 mg, 0.015 mmol), Cu(BF4)2 (80 mg, 0.29 mmol), (NH4)2TiF6(10 mg, 0.05 mmol) and H2TiF6 (100 μL) were mixed in DMA/H2O/EtOH (1
:
1.5
:
1, 3.5 mL) in a 10 mL glass container and tightly capped with a Teflon vial and heated at 95 °C for 48 h. After cooling to room temperature, the resulting blue-green crystals were harvested and washed with fresh DMA three times. Yield is ∼53% (based on the ligand).
Synthesis of NTU-73.
HL (4 mg, 0.015 mmol), Cu(BF4)2 (80 mg, 0.29 mmol), (NH4)2ZrF6(10 mg, 0.04 mmol) and H2ZrF6 (100 μL) were mixed in DMA/H2O/EtOH (1
:
1
:
1, 3 mL) in a 10 mL glass container and tightly capped with a Teflon vial and heated at 90 °C for 48 h. After cooling to room temperature, the resulting blue-green crystals were harvested and washed with fresh DMA three times. Yield is ∼32% (based on ligand).
Results and discussion
Synthesis and structure characterization
A reaction of copper(II) fluorosilicate with HL afforded polyhedron-shaped crystals (NTU-71). It crystallizes in a tetragonal I4122 space group with the formula of [Cu(HL)2SiF6]·xguest (Table S1†). Its asymmetric unit includes one ligand, half a Cu2+ ion and half a SiF62− ion (Fig. S1†). The charge of all the Cu2+ ions in NTU-71 is balanced by SiF62− ions, and thus, all the HL ligands are neutral. The single Cu node here is coordinated by four imidazole nitrogen atoms from four independent HL and two F atoms from two SiF62− anions. Meanwhile, the three general branching points of the ligand take part in the coordination with the Cu nodes by two imidazole N atoms, while the carboxylate sites are free. As a third component of this framework, two F atoms from the SiF62− anion join the coordination of the adjacent Cu node, yielding two helical chains in this 3D framework (Fig. S2†). Obviously, two channels with densely incorporated carboxylic groups can be observed along the b- and c-axis (Fig. 1a and S3 and S4†). Like crab pincers, these carboxylate groups are packed in pairs throughout the whole structure, and thus form a defined nanospace of about 4.5 × 5.2 Å2 (Fig. 1b and c). In addition, these carboxylic pincers are also available in another semiregular channel with an aperture size of 3.9 × 5.6 Å2 (Fig. 1d). To understand this structure better, topology analysis was performed. Cu(HL)2 is an interpenetrated framework, of which each net can be simplified as a classical dia topology. Interestingly, these interpenetrated dia nets are then bridged by a 2-connected SiF62− anion, yielding a new 6-c topology with a point symbol of {4^8.6^7} (Fig. S5†).
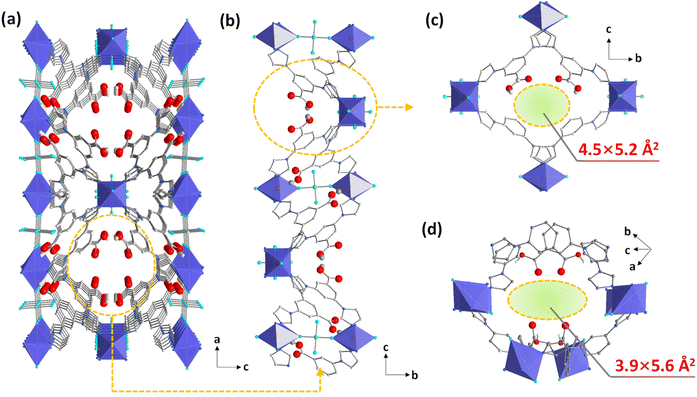 |
| Fig. 1 Structure of NTU-71: view of the 1D channel that features carboxylic pincers along the b-axis (a); view of the pincers arranged alternately along the a-axis (b); local view of the nanospace defined by carboxylic pincers (c and d). Color codes: C, grey; N, blue; F, cyan; Si, yellow; O, red. The blue red polyhedron represents an octahedral coordinated Cu atom. | |
Nanospace tuning
Encouraged by this structure and reticular chemistry,30 the fine pore size engineering of the carboxylic-functionalized MOFs was performed, in which the balancing SiF62− ions were replaced by TiF62− and ZrF62−, respectively. With a general formula of [Cu(HL)2MF6], the other two iso-reticular MOFs (M = Ti2+ and Zr2+, termed NTU-72 and NTU-73) were obtained (Fig. S6–S8†). Compared with that of NTU-71 (dO⋯O: 5.021 Å), the pincer distance increased to 5.583 Å and 5.911 Å in NTU-72 and NTU-73, respectively (Fig. 2). These fine changes may offer a fitted nanospace for carboxylic pincers to recognize C2H2 clusters. The phase purity of these MOFs was examined by powder X-ray diffraction (PXRD). The diffraction peaks of the as-synthesized NTU-series are in good agreement with the simulated patterns, as well as the activated phases (Fig. S9–S11†).
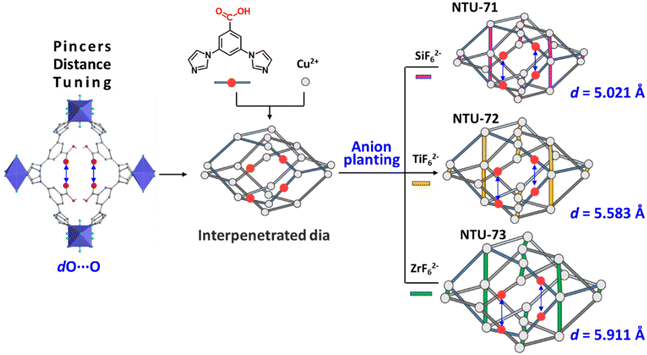 |
| Fig. 2 Fine distance tuning in NTU-seriesvia the anion planting approach, where dO⋯O increases at the sub-angstrom level. | |
Pore evaluation
Permanent porosity of NTU-71 to NTU-73 was further explored by N2 (77 K) and CO2 (195 K and 298 K) adsorption measurements. They all display negligible N2 uptakes, but rapid and revisable type-I CO2 adsorptions, indicating typical microporous characteristics. Their maximum CO2 uptakes are 77, 82 and 71 cm3 cm−3, respectively (Fig. 3a). Their calculated BET surface areas (based on CO2, 195 K) reach 242, 315 and 225 m2 g−1, respectively, while the analysis of pore size distributions illustrate the systematic change of the nanospace (inset of Fig. 3a).
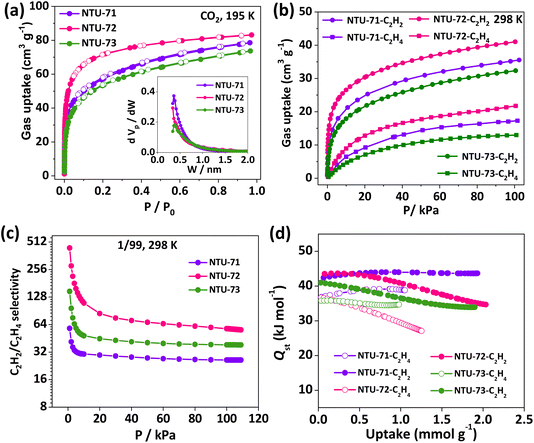 |
| Fig. 3 Pore evaluation of the NTU-series: CO2 adsorption isotherms (a), inset is the pore size distribution; C2H2 and C2H4 isotherms (b); IAST selectivity (c); isosteric heats (d). | |
Static adsorption selectivity and isosteric heats
Single-component adsorption isotherms of C2H2 and C2H4 were collected (Fig. 3b and S16–S21†), respectively. At 298 K, they all show relatively higher C2H2 uptakes compared with C2H4. Interestingly, the C2H2 uptakes (10.6 cm3 g−1 and 8.2 cm3 g−1) at 1 kPa are almost 13–14 times higher than that of C2H4 (0.8 cm3 g−1 and 0.6 cm3 g−1) in NTU-71 and NTU-73, and this ratio (15.8 cm3 g−1vs. 1.0 cm3 g−1) increases to 16 in NTU-72 (Fig. S22†). These higher uptake ratios reflect preferred affinity of the three frameworks toward C2H2 over C2H4. The adsorption selectivity of C2H2/C2H4 (v/v, 1/99) in these three MOFs was predicted by ideal adsorbed solution theory (IAST) (Fig. 3c and S23–S35†).31,32 The low-pressure C2H2 adsorption selectivity of NTU-72 (∼441) is much higher than that of both NTU-71 (∼58) and NTU-73 (∼147), and is also higher than that of ZUL-200 (60),33 NTU-69 (49),34 SIFSIX-2-Cu-i (45),7 and JCM-1 (9).35 In addition, the C2H2 adsorption selectivities remain as high as 26, 56 and 38 at 1 bar. Particularly, the selectivity of NTU-72 is only surpassed by the benchmark UTSA-200 (6320),36 ZU-33 (1100),37 and ZUL-series (175 and 114),33 and is higher than most of the well known MOFs.7,38
To explore the reason for such high selectivities, isosteric heats of adsorption (Qst) of C2H2 and C2H4 were calculated (Fig. 3d and S36–S41†). The Qst values of C2H4 at low pressure (36.8, 36.7 and 35.7 kJ mol−1) are all lower than that of C2H2 (42.4, 43.5, and 40.9 kJ mol−1) for the NTU-series. Similar to the higher C2H2 uptake, the adsorption enthalpy difference of NTU-72 (6.7 kJ mol−1) is higher than that of NTU-71 (5.6 kJ mol−1) and NTU-73 (5.2 kJ mol−1). This may be due to the fine fitting of the pore geometry and size in NTU-72 to the C2H2 molecule, while the relatively larger or smaller nanospace in NTU-71 or NTU-73 provides a small interaction difference.
Breakthrough experiments were performed on NTU-72, in which the binary mixtures of C2H2/C2H4 (v/v, 1/99) were introduced into the system. Pure C2H4 passed through the sample bed as the first component (4.2 min g−1), while C2H2 is retained in the column (Fig. 4a). The mixtures of C2H2 and C2H4 were detected at about 71.1 min g−1. This result shows that highly pure C2H4 (99.95%) can be directly collected in 66.9 min g−1 at the adsorption stage. According to the sample weight and gas velocity, NTU-72 could produce 331.1 cm3 (STP) per g of pure C2H4 from this mixture. To confirm the selective adsorption, the saturated adsorbents were then swept by helium at 298 K until no signal was detected. Subsequently, C2H2 was desorbed by rapid heating (up to 323 K), and its purity is approximately 99.36% (Fig. 4b and S42 and S43†). Therefore, as an emerging example, NTU-72 can deliver both highly pure C2H4 and C2H2 in one adsorption/desorption cycle, although the recovery of poly-grade C2H4 has been widely reported (Table S2†). Structural stability was then confirmed by cycling breakthrough experiments, where nearly the same separation curves were displayed (Fig. 4c). Further experiments with varied gas velocity (2 and 10 cm3 min−1) also showed obvious separation, and the C2H4 productivity (319.6 and 323.8 cm3 (STP) per g) has hardly suffered any impact (Fig. 4d and S44†).
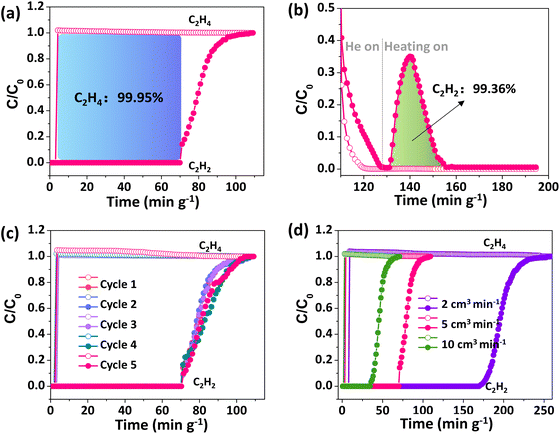 |
| Fig. 4 Breakthrough curves of NTU-72 for a C2H2/C2H4 mixture (1/99, v/v) at 298 K, 1 bar, velocity is 5 cm3 min−1 (a); desorption curves (b); cycle breakthrough tests (c); (d) breakthrough curves at different velocities. | |
To gain better understanding of the separation performance, C2H2- and C2H4-loaded NTU-72 crystals were investigated at 298 K.39 As expected, both gases interact with the carboxylic sites (Fig. S45 and S46†). For NTU-72⊃C2H2, the occupancy of C2H2 was refined to be 1, which corresponds to 30.5 cm3 g−1, slightly lower than the amount in the C2H2 adsorption experiment (41 cm3 g−1) at 1 bar. This difference may be caused by two reasons: (1) the C2H2 molecules at other free space are strongly disordered and hard to be accurately modelled; (2) part of the C2H2 escaped during the sealing of the glass tube by using a hot candle. Despite this, the position and geometry of the adsorbed C2H2 molecules can be clearly identified in this confined nanospace. The C2H2 molecules are confined in the channel through three types of hydrogen bonds in different binding geometries (Fig. 5a and b). Acting as an acceptor and a donor, the OCOOH and OHCOOH moieties connect the terminal hydrogen and carbon atoms of a C2H2 molecule. In addition, the OHCOOH moiety also forms a hydrogen bond with another C2H2 molecule, resulting in close packing of the two gas molecules with intermolecular hydrogen bonds. Meanwhile, the terminal HC2H2 forms hydrogen bonds with CC2H2 from its symmetrically equivalent molecule, so that a C2H2 tetramer with C2h symmetry is formed periodically in NTU-72 (Fig. S47–S49†), which is different from the S4 symmetric C2H2 tetramer in SIFSIX-Cu-i.7 Although the C2H2 clusters have been investigated,20,21 a compact C2H2 tetramer with C2h symmetry being stabilized by chelating carboxylic pincers is found for the first time. Sharply in contrast, two types of hydrogen bonds are observed in NTU-72⊃C2H4 (Fig. 5c and d and S50–S52†). Particularly, dCC2H4⋯H–OCOOH (2.705 Å) is much longer than that of dCC2H2⋯H–OCOOH (1.871 and 2.588 Å), as well as the longer dOCOOH⋯H–OC2H4, reflecting relative weak carboxylic–C2H4 interaction. Furthermore, no intermolecular interaction is found between the two C2H4 molecules, as their distance is far. Therefore, the carboxylic sites contribute mainly to the recognition of the C2H2 tetramer, and meanwhile, the finely engineered pore geometry and nano-size confined this cluster to be released in the pure form. Further Raman spectra of gas loaded NTU-72 show that the peak at 1950 cm−1 belonging to the tensile vibration of C2H2 was found in NTU-72, and in contrast, no helium peak was found (Fig. 5e).40,41 In addition, the corresponding peak that belongs to C2H4 (theoretical position: 1689 cm−1) can't be observed. Therefore, it is rational to conclude that the C2H2 clusters assembled in a periodic configuration in NTU-72, agreeing well with the crystallographic studies.
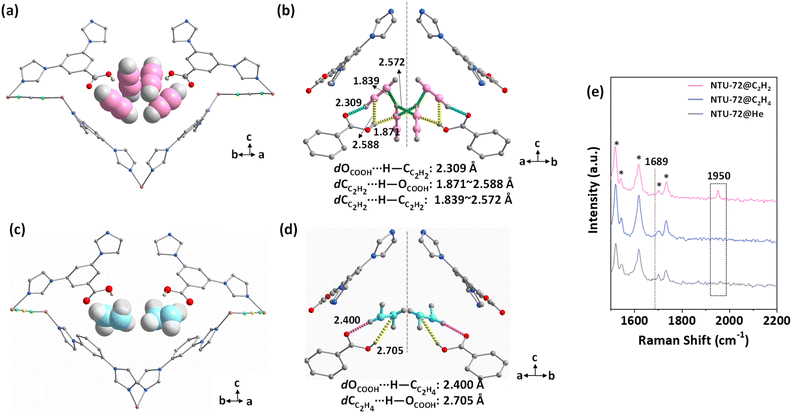 |
| Fig. 5 Structure of C2H2- (a and b) and C2H4- (c and d) loaded NTU-72. Raman spectra of gas-loaded NTU-72 (e). | |
Stability
The water/air stability of NTU-72 was then evaluated at room temperature. As shown in Fig. S53,†NTU-72 retained a polyhedral shape after air treatment, but some changes occurred in the shape after water treatment. Single crystal X-ray analysis showed that the water treated crystal (NTU-72⊃H2O, for 72 h) retains the framework of the as-synthesized phase (Table S1†), confirmed further by PXRD analysis. Additionally, adsorption measurements revealed that the CO2 capacity of all treated samples is consistent with that of fresh samples, except for a slight decrease in the air treated sample for 72 h. These results confirm that NTU-72 has good water and moisture robustness (Fig. 6a and b). Considering the structure, the maintained crystallinity of NTU-72 is due to the relatively strong Cu–N bond, similar to that of NTU-67, having a highly robust framework with a Cu–N coordination sphere also.39 In addition, the crystal structure of NTU-72⊃H2O shows that a couple of carboxylic pincers bind a tetra-water cluster via short hydrogen bonds (dO⋯O: 2.586–2.662 Å), which may provide a blocking effect for other water molecules to reach the coordination bonds (Fig. 6c).
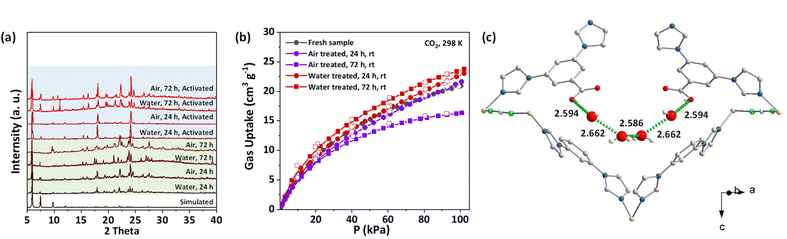 |
| Fig. 6 PXRD (a) and CO2 adsorption isotherms (b) of water and moisture treated NTU-72; local view of the structure of NTU-72⊃H2O (c), and the unit of O⋯O distance is Å. | |
Conclusions
In conclusion, as needed for the pure C2 hydrocarbons, we have finely tuned a new family of carboxylic-functionalized MOFs and afforded NTU-72 with high adsorption C2H2/C2H4 selectivity as well as unprecedented recovery of highly pure C2H4 and C2H2. The carboxylic pincers contribute to the recognition of a C2H2 tetramer, and the fitted pore geometry/size enforces synergistic binding and restricts this cluster to be released in a pure form. In addition, this material is stable in water and moisture environments. The foregoing results not only present an excellent MOF for energy-saving C2 hydrocarbon purification, but also provide a novel approach for the selective binding of gas clusters for designing advanced MOFs.
Data availability
All data can be found in the main text and ESI.†
Author contributions
J. D. conceived the idea of this work. Y. D. and Y. H. carried out the experiments and analyzed the results. J. D. and J. B. wrote the paper. C. W. performed the repeated adsorption experiments. H. W. prepared the ligands. All authors gave valuable discussion and suggestion for the final draft.
Conflicts of interest
The authors declare no competing interests.
Acknowledgements
We are thankful for the financial support of the National Natural Science Foundation of China (22171135 and 22271150), the Young and Middle-aged Academic Leader of Jiangsu Provincial Blue Project, the State Key Laboratory of Materials-Oriented Chemical Engineering (ZK201803) and Open Foundation of Hunan Provincial Key Laboratory of Controllable Preparation and Functional Application of Fine Polymers (E22306).
References
- D. S. Sholl and R. P. Lively, Nature, 2016, 532, 435–437 CrossRef PubMed.
- R. Matsuda, R. Kitaura, S. Kitagawa, Y. Kubota, R. V. Belosludov, T. C. Kobayashi, H. Sakamoto, T. Chiba, M. Takata, Y. Kawazoe and Y. Mita, Nature, 2005, 436, 238–241 CrossRef CAS PubMed.
- Y. Chai, X. Han, W. Li, S. Liu, S. Yao, C. Wang, W. Shi, I. da-Silva, P. Manuel, Y. Cheng, L. D. Daemen, A. J. Ramirez-Cuesta, C. C. Tang, L. Jiang, S. Yang, N. Guan and L. Li, Science, 2020, 368, 1002–1006 CrossRef CAS PubMed.
- K. J. Chen, D. G. Madden, S. Mukherjee, T. Pham, K. A. Forrest, A. Kumar, B. Space, J. Kong, Q. Y. Zhang and M. J. Zaworotko, Science, 2019, 366, 241–246 CrossRef CAS PubMed.
- F. Studt, F. Abild-Pedersen, T. Bligaard, R. Z. Sorensen, C. H. Christensen and J. K. Norskov, Science, 2008, 320, 1320–1322 CrossRef CAS PubMed.
- E. D. Bloch, W. L. Queen, R. Krishna, J. M. Zadrozny, C. M. Brown and J. R. Long, Science, 2012, 335, 1606–1610 CrossRef CAS PubMed.
- X. L. Cui, K. J. Chen, H. B. Xing, Q. W. Yang, R. Krishna, Z. B. Bao, H. Wu, W. Zhou, X. L. Dong, Y. Han, B. Li, Q. L. Ren, M. J. Zaworotko and B. L. Chen, Science, 2016, 353, 141–144 CrossRef CAS PubMed.
- B. Li, X. Cui, D. O'Nolan, H. M. Wen, M. Jiang, R. Krishna, H. Wu, R. B. Lin, Y. S. Chen, D. Yuan, H. Xing, W. Zhou, Q. Ren, G. Qian, M. J. Zaworotko and B. Chen, Adv. Mater., 2017, 29, 1704210–1704217 CrossRef PubMed.
- H. Zeng, M. Xie, T. Wang, R. J. Wei, X. J. Xie, Y. F. Zhao, W. G. Lu and D. Li, Nature, 2021, 595, 542–548 CrossRef CAS PubMed.
- A. Cadiau, K. Adil, P. M. Bhatt, Y. Belmabkhout and M. Eddaoudi, Science, 2016, 353, 137–140 CrossRef CAS PubMed.
- Q. Dong, Y. Huang, J. Wan, Z. Lu, Z. Wang, C. Gu, J. Duan and J. Bai, J. Am. Chem. Soc., 2023 DOI:10.1021/jacs.3c00515.
- H. Li, M. Eddaoudi, M. O'Keeffe and O. M. Yaghi, Nature, 1999, 402, 276–279 CrossRef CAS.
- S. Krause, V. Bon, I. Senkovska, U. Stoeck, D. Wallacher, D. M. Tobbens, S. Zander, R. S. Pillai, G. Maurin, F. X. Coudert and S. Kaskel, Nature, 2016, 532, 348–352 CrossRef CAS PubMed.
- S. H. Lo, L. Feng, K. Tan, Z. H. Huang, S. Yuan, K. Y. Wang, B. H. Li, W. L. Liu, G. S. Day, S. S. Tao, C. C. Yang, T. T. Luo, C. H. Lin, S. L. Wang, S. J. L. Billinge, K. L. Lu, Y. J. Chabal, X. D. Zou and H. C. Zhou, Nat. Chem., 2020, 12, 90–97 CrossRef PubMed.
- H. Wang, X. L. Dong, V. Colombo, Q. N. Wang, Y. Y. Liu, W. Liu, X. L. Wang, X. Y. Huang, D. M. Proserpio, A. Sironi, Y. Han and J. Li, Adv. Mater., 2018, 30, 1805088–1805096 CrossRef PubMed.
- Y. L. Peng, T. Pham, P. Li, T. Wang, Y. Chen, K. J. Chen, K. A. Forrest, B. Space, P. Cheng, M. J. Zaworotko and Z. Zhang, Angew. Chem., Int. Ed., 2018, 57, 10971–10975 CrossRef CAS PubMed.
- R. B. Lin, L. B. Li, H. Wu, H. Arman, B. Li, R. G. Lin, W. Zhou and B. L. Chen, J. Am. Chem. Soc., 2017, 139, 8022–8028 CrossRef CAS PubMed.
- J. P. Zhang, P. Q. Liao, H. L. Zhou, R. B. Lin and X. M. Chen, Chem. Soc. Rev., 2014, 43, 5789–5814 RSC.
- N. Behera, J. Duan, W. Jin and S. Kitagawa, EnergyChem, 2021, 3, 100067–100124 CrossRef CAS.
- K. Shuler and C. E. Dykstra, J. Phys. Chem. A, 2000, 104, 11522–11530 CrossRef CAS.
- J. G. Yu, S. J. Su and J. E. Bloor, J. Phys. Chem., 1990, 94, 5589–5592 CrossRef CAS.
- R. Custelcean and M. G. Gorbunova, J. Am. Chem. Soc., 2005, 127, 16362–16363 CrossRef CAS PubMed.
- X. Duan, R. J. Song, J. C. Yu, H. L. Wang, Y. J. Cui, Y. Yang, B. L. Chen and G. D. Qian, RSC Adv., 2014, 4, 36419–36424 RSC.
- F. Ragon, B. Campo, Q. Yang, C. Martineau, A. D. Wiersum, A. Lago, V. Guillerm, C. Hemsley, J. F. Eubank, M. Vishnuvarthan, F. Taulelle, P. Horcajada, A. Vimont, P. L. Llewellyn, M. Daturi, S. Devautour-Vinot, G. Maurin, C. Serre, T. Devic and G. Clet, J. Mater. Chem. A, 2015, 3, 3294–3309 RSC.
- J. Duan, M. Higuchi, J. Zheng, S. I. Noro, I. Y. Chang, K. Hyeon-Deuk, S. Mathew, S. Kusaka, E. Sivaniah, R. Matsuda, S. Sakaki and S. Kitagawa, J. Am. Chem. Soc., 2017, 139, 11576–11583 CrossRef CAS PubMed.
- Z. Y. Lu, L. T. Du, R. Y. Guo, G. B. Zhang, J. G. Duan, J. F. Zhang, L. Han, J. F. Bai and J. T. Hupp, J. Am. Chem. Soc., 2021, 143, 17942–17946 CrossRef CAS PubMed.
- M. X. Zhang, W. Zhou, T. Pham, K. A. Forrest, W. L. Liu, Y. B. He, H. Wu, T. Yildirim, B. L. Chen, B. Space, Y. Pan, M. J. Zaworotko and J. F. Bai, Angew. Chem., Int. Ed., 2017, 56, 11426–11430 CrossRef CAS PubMed.
- L. Du, Z. Lu, K. Zheng, J. Wang, X. Zheng, Y. Pan, X. You and J. Bai, J. Am. Chem. Soc., 2013, 135, 562–565 CrossRef CAS PubMed.
- M. Li, D. Li, M. O'Keeffe and O. M. Yaghi, Chem. Rev., 2014, 114, 1343–1370 CrossRef CAS PubMed.
- N. W. Ockwig, O. Delgado-Friedrichs, M. O'Keeffe and O. M. Yaghi, Acc. Chem. Res., 2005, 38, 176–182 CrossRef CAS PubMed.
- J. G. Duan, M. Higuchi, S. Horike, M. L. Foo, K. P. Rao, Y. Inubushi, T. Fukushima and S. Kitagawa, Adv. Funct. Mater., 2013, 23, 3525–3530 CrossRef CAS.
- R. Krishna, RSC Adv., 2015, 5, 52269–52295 RSC.
- J. Shen, X. He, T. Ke, R. Krishna, J. M. van Baten, R. Chen, Z. Bao, H. Xing, M. Dinca, Z. Zhang, Q. Yang and Q. Ren, Nat. Commun., 2020, 11, 6259 CrossRef CAS PubMed.
- Y. Huang, Y. Xu, B. Zheng, Z. Wang, Q. Dong and J. Duan, Energy Fuels, 2020, 34, 11315–11321 CrossRef CAS.
- J. Lee, C. Y. Chuah, J. Kim, Y. Kim, N. Ko, Y. Seo, K. Kim, T. H. Bae and E. Lee, Angew. Chem., Int. Ed., 2018, 57, 7869–7873 CrossRef CAS PubMed.
- H. Li, L. Li, R. B. Lin, G. Ramirez, W. Zhou, R. Krishna, Z. Zhang, S. Xiang and B. Chen, ACS Sustainable Chem. Eng., 2019, 7, 4897–4902 CrossRef CAS PubMed.
- Z. Zhang, X. Cui, L. Yang, J. Cui, Z. Bao, Q. Yang and H. Xing, Ind. Eng. Chem. Res., 2018, 57, 7266–7274 CrossRef CAS.
- J. Wang, L. Li, L. Guo, Y. Zhao, D. Xie, Z. Zhang, Q. Yang, Y. Yang, Z. Bao and Q. Ren, Chem.–Eur. J., 2019, 25, 15516–15524 CrossRef CAS PubMed.
- Q. B. Dong, Y. H. Huang, K. Hyeon-Deuk, I. Y. Chang, J. M. Wan, C. L. Chen, J. G. Duan, W. N. Jin and S. Kitagawa, Adv. Funct. Mater., 2022, 32, 2203745–2203755 CrossRef CAS.
- H. Wang, Y. Duan, Y. Wang, Y. Huang, K. Ge, S. Wang, B. Zheng, Z. Wang, J. Bai and J. Duan, ACS Appl. Mater. Interfaces, 2022, 14, 13550–13559 CrossRef CAS PubMed.
- G. C. Guo, S. H. Lin, F. K. Zheng, Z. C. Dong and J. S. Huang, Spectrosc. Spectral Anal., 2000, 20, 830–832 CAS.
Footnotes |
† Electronic supplementary information (ESI) available: Synthesis and characterization of these three crystals, PXRD, TGA, IR, sorption isotherms, IAST, breakthrough experiments and fitting parameters. CCDC 2211444–2211448, 2251139. For ESI and crystallographic data in CIF or other electronic format see DOI: https://doi.org/10.1039/d3sc00877k |
‡ These authors contributed equally to this work. |
|
This journal is © The Royal Society of Chemistry 2023 |
Click here to see how this site uses Cookies. View our privacy policy here.