DOI:
10.1039/D2SC06436G
(Edge Article)
Chem. Sci., 2023,
14, 1138-1144
Regulating the assembly and expansion of the silver cluster from the Ag37 to Ag46 nanowheel driven by heteroanions†
Received
22nd November 2022
, Accepted 26th December 2022
First published on 26th December 2022
Abstract
Precise control over the shape and size of metal nanoclusters through anion template-driven self-assembly is one of the key scientific goals in the nanocluster community, however, it is still not understood comprehensively. In this work, we report the controllable synthesis and atomically precise structures of silver nanowheels Ag37 and Ag46, using homo (Cl− ions) and heteroanion (Cl− and CrO42− ions) template strategies, along with macrocyclic p-phenyl-thiacalix[4]arene and small iPrS− ligands. Structural analyses revealed that in Ag37, Cl− ions serve as both local and global templates, whereas CrO42− ions function as local and Cl− ions as global templates in Ag46, resulting in a pentagonal nanowheel (Ag37) and a hexagonal (Ag46) nanowheel. The larger ionic size and more negative charges of CrO42− ions than Cl− ions offer more coordination sites for the silver atoms and are believed to be the key factors that drive the nanowheel core to expand significantly. Also, by taking advantage of the deep cavity of thiacalix[4]arene with an extended phenyl group, Ag46 has been used as a host material for dye adsorption depending on the charge and size of organic dyes. The successful use of heteroanions to control the expansion of well-defined silver nanowheels fills the knowledge gap in understanding the directing role of heteroanions in dictating the shape and size of nanoclusters at the atomic level.
Introduction
Metal nanoclusters (NCs) are highly attractive synthetic targets in cluster chemistry due to their potential application as catalysts, sensors, and bioimaging agents, to name a few.1 Synthesizing NCs in a controlled manner is much more challenging than the trial-and-error approach because such processes always involve the intricate assembly of multiple components controlled by thermodynamic and kinetic factors.2 Furthermore, controlled synthesis offers the functionalization of novel metal NCs with customizable properties and applications that are unavailable to simpler systems.
After decades of effort, the anion-template method is well-established for the synthesis of silver NCs. It has been proven to be useful for adding complexity and functionality with a high probability of creating high-nuclearity NCs that are somewhat predictable.3 As a subclass, heteroanion templates with different sizes, geometries, and charge states have received less attention in the synthesis of silver NCs than homoanion templates.4 Xie et al. used Cl− and [(O)2V2O6]4− ions to produce an Ag36 NC.5 Our group isolated a high-nuclearity [(SO4)2(W5O19)2@Ag90] NC, where the heteroanions of SO42− and W5O198− are both in situ generated.6 We are motivated to use the concept of assembling heteroanion templates in the early stage of the assembly, which may interfere with the formation of the core by aggregating silver atoms around them in different ways and thus is a feasible way to regulate the shape, size, and specific metal atom and ligand arrangement of silver NCs. A comparative study of template effects (homo and heteroanion templates) on the structure and figuring out the factors that lead to the core variation of the NCs and their structural details are really complex processes and are rarely investigated.
On the other hand, protecting ligands are also crucial for controlling the physicochemical properties, core size, and structural stability of silver NCs.7 Besides using common thiolate,8 phosphine,9 and alkynyl ligands,10 or combinations of these,11 the unusual macrocyclic ligands (e.g., thiacalix[4]arene) hold promising potential for passivating silver NCs, such as Ag18,12 Ag21,13 Ag34,14 Ag35,15 Ag42,16 Ag88,17 and Ag155.18 In addition, thiacalix[4]arene or its derivative represents a fascinating cavity that could be potentially used to encapsulate guest molecules.19 As such, it would be of great interest to further develop silver NCs protected by thiacalix[4]arene, especially those comprising different anion templates, which will give us an opportunity to better understand the controlled synthesis, assembly, and structural evolution mechanism.
Here, we present two silver nanowheels [Cl7@Ag37Na(PTC4A)5(iPrS)10(DMF)4(CH3CN)2]·Cl·2CH2Cl2 (Ag37) and [(CrO4)2Cl6@Ag46(PTC4A)6(iPrS)12(CH3CN)6] (Ag46) that manifest the core size expansion relying on the heteroanion template strategy in the presence of macrocyclic p-phenyl-thiacalix[4]arene (H4PTC4A) as a protecting ligand. More specifically, with the Cl− ions, an Ag37 NC is obtained, exhibiting a pentagonal waterwheel-like entity, while with a heteroanion combination (Cl− and CrO42−), the metal core expands from Ag37 to Ag46, adopting a hexagonal waterwheel-like entity. Furthermore, the facile and scalable synthesis of Ag46 has been demonstrated for the selective adsorption and separation of cationic dyes methylene blue (MB) and rhodamine B (RhB) in water through host–guest interactions in the extended cavity of the PTC4A4− ligand. To the best of our knowledge, this is the first example of atomically precise silver NCs being used for dye adsorption studies. These observations will inspire the use of heteroanion templates to design NC assemblies with various geometries and functional properties.
Results and discussion
Under the solvothermal conditions, the reaction of polymeric [iPrSAg]n, PhCOOAg, H4PTC4A, and NaCl as a chloride source produced yellow fusiform crystals of Ag37 in a 5% yield (1.3 mg; based on H4PTC4A), whereas NaCl and (NH4)2Cr2O7 (sources of Cl− and CrO42−, respectively) produced black-red cubic crystals of Ag46 in a 50% yield (14 mg; based on H4PTC4A) (Scheme 1 and Fig. S1†). Of note, from the perspective of scientific research, the feasibility of scale-up synthesis is important for property exploration and large-scale applications.20 However, metal NCs are known to be sensitive to synthesis conditions, and it is extremely difficult to achieve high-yield synthesis. Fortunately, we managed to achieve a tenfold scale-up for the synthesis of Ag46, which produced 140 mg of the final product from a one pot reaction. The synthetic details and a series of characterization studies, including single-crystal X-ray diffraction (SCXRD), powder X-ray diffraction (PXRD), UV-vis spectroscopy, and infrared (IR) spectroscopy, are provided in the ESI.†
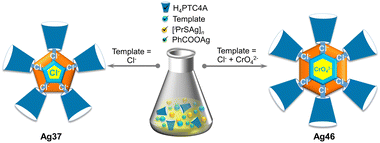 |
| Scheme 1 Synthetic routes for Ag37 and Ag46. | |
X-ray structures of Ag37 and Ag46
SCXRD analyses reveal that Ag37 crystallizes in the triclinic system with the space group of P
, whereas Ag46 crystallizes in the monoclinic P21/n space group (Table S1†). The asymmetric units of both the nanowheels comprise an entire molecule. As shown in Fig. 1, Ag37 possesses a waterwheel-like structure with a pentagonal metal core that is 1.3 nm × 0.4 nm (excluding the organic ligands). The structure of Ag37 is composed of 37 Ag+ cations and 1 Na+ cation, 7 Cl− templates, 5 PTC4A4− and 10 iPrS− ligands, and coordinated solvent molecules of 2 CH3CN and 4 DMF (Fig. 1a and b). The metallic skeleton of 37 silver atoms is a Ag2 (core)@Ag35 (shell) configuration, which can be described as three parts: in the centre, two silver atoms act as the innermost core, and on the surface, two eclipsed Ag5 pentagons above and below the equatorial plane and a bracelet-like Ag25 ring form the Ag35 shell (Fig. S2†). The Ag⋯Ag distances fall in the range of 2.76–3.37 Å. The Ag35 shell is covered by external organic ligands and solvent molecules. All the PTC4A4− ligands are deprotonated and adopt a distorted cone conformation, coordinating with five Ag atoms using a μ5-κo3:κo3:κo3:κo3:κs1:κs1:κs1:κs1 mode to produce a shuttlecock-like Ag5@PTC4A unit (Fig. 1c). Among the Ag5@PTC4A units, four Ag atoms are symmetrically arranged, each of which is ligated by two phenolic O atoms and one bridging S atom. There is one more Ag atom at the centre of the pentagonal metal core (Fig. 1c; blue ball), approximately 1.4–1.7 Å offset above the center of the Ag4 quadrangle formed by four silver atoms at the bottom, connected by four phenolic O atoms, and is further stabilized by either DMF or CH3CN (Fig. S3†). The units are interconnected with each other by argentophilic interactions to form bracelet-like Ag25@(PTC4A)5 (Fig. 1d). The Ag–O and Ag–S bonds linked to PTC4A4− ligands are within the limits of 2.23–2.63 Å and 2.52–2.63 Å, respectively. All the iPrS− ligands are distributed uniformly on both sides of the nanowheel, connecting the Ag25@(PTC4A)5 unit and two Ag5 pentagons above and below the equatorial plane in μ4-κ1:κ1:κ1:κ1 mode (Ag–S distances, 2.35–2.54 Å; Fig. S4†).
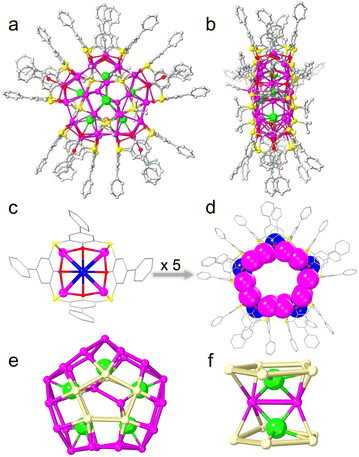 |
| Fig. 1 (a) and (b) Total X-ray crystal structure of Ag37 viewed along two orthogonal directions. (c) Coordination mode of the PTC4A4− ligand towards Ag atoms. (d) The pentagonal arrangement of PTC4A4− ligands on the Ag25 bracelet. The silver atoms are shown in a space-filling pattern. (e) Five Cl− ions held between the Ag2 core and the Ag35 shell. (f) The other two Cl− ions capping on the inner Ag2 core. Colour code: Ag, purple, blue, and pale yellow; Na, brown; O, red; S, yellow; Cl, green; C, gray; N, pale blue. | |
It is worth mentioning that each Ag5@PTC4A unit is subsequently connected with a Cl− template, which fills the inner space of the silver shell around the Ag2 core in a pentagonal distribution (Fig. 1e). Two Ag5 pentagons on the surface of the silver shell are arranged in a face-to-face manner above and below the Cl− ion layer. These five Cl− ions (2 μ5 and 3 μ4) play a critical role in the global template effect of connecting the outer Ag35 shell and the inner Ag2 core. Interestingly, the other two Cl− ions are enclosed in the center of the silver shell, covering the Ag2 core at both ends, up and down. These two Cl− ions exert the local template effect to ligate to the innermost two Ag atoms and three Ag atoms of the Ag5 pentagon in the same μ5 mode (Fig. 1f). Ag37 has a wavy ABAB pattern along the [100] direction, and one of the phenyl groups of the PTC4A4− ligand penetrates into the cavity of the PTC4A4− ligand of the nanowheel in the adjacent layer (Fig. S5†).
On the other hand, Ag46 is a hexagonal waterwheel-like structure with an enlarged radial diameter of 1.70 nm and nearly the same axial thickness of 0.4 nm as that of Ag37. In detail, the structure has a fascinating hexagonal core–shell skeleton with an inner Ag4 core in the center with up to six Cl− and two CrO42− as anion templates, enveloped by a larger Ag42 shell, which is further covered and stabilized by 12 iPrS− and 6 PTCTA4− ligands together with 6 CH3CN solvent molecules (Fig. 2a). Unlike Ag37, the outer shell of Ag46 is anchored by six PTC4A4− ligands, adopting two different coordination modes to form the [Ag5@(PTC4A)]6 unit: two μ5-κo3:κo3:κo3:κo3:κs1:κs1:κs1:κs1 and four μ5-κo3:κo3:κo2:κo2:κs1:κs1:κs1:κs1. And each of the central Ag atoms (Fig. 2b; blue balls) in the bowl of Ag5@(PTC4A) units is ligated to one CH3CN molecule (Fig. S6†). Six such units are linked together by argentophilic interactions to form larger bracelet-like Ag30@(PTC4A)6 with a hexagonal arrangement (Fig. 2b). The iPrS− ligands link Ag30@(PTC4A)6 and two Ag6 hexagons above and below the equatorial plane through sulfur atoms, adopting μ4-κ1:κ1:κ1:κ1 ligation mode (Fig. S7†). Within this structure, six Cl− ions (1 μ3, 2 μ4, 2 μ6, and 1 μ7) act as global templates and are arranged in a hexagonal ring that connects the outer Ag42 shell and the innermost Ag4 core, providing reasonable stability to the entire nanowheel (Fig. 2c). In addition, the two middle Ag6 hexagons on the surface of the silver shell and the innermost Ag4 tetragon are interiorly supported by two CrO42− ions via μ8-κ3:κ3:κ1:κ1 and μ8-κ4:κ2:κ1:κ1 modes (Fig. 2d and e). Crystal packing of Ag46 shows a layered structure along the [010] direction, and the nanowheels in each layer are horizontally arranged side by side (Fig. S8†).
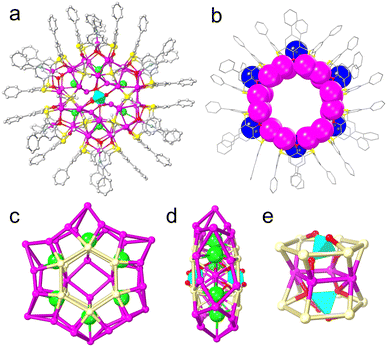 |
| Fig. 2 (a) Total X-ray crystal structure of Ag46. (b) The hexagonal arrangement of PTC4A4− ligands on the Ag30 bracelet. The silver atoms are shown in a space-filling pattern. (c) Six Cl− ions anchored between the Ag4 core and the Ag42 shell. (d) The structure of Ag4@(CrO4)2Cl6@Ag42. (e) Two CrO42− anions capping on the inner Ag4 core. The cyan polyhedra represent CrO42−. Colour code: Ag, purple, blue, and pale yellow; O, red; S, yellow; Cl, green; C, gray; N, pale blue. | |
The most intriguing aspects of Ag37 and Ag46 are the central Cl− and CrO42− templates. In the case of Ag37, two singly charged Cl− ions as local anion templates passivated a linear Ag2 core in the center. And another set of five Cl− ions with a pentagonal distribution serves as a global template in the interior. Not only that, the number of silver atoms in each part of the outer shell (2 Ag5 pentagons above and below the equatorial plane and 1 Ag25 bracelet) and ligands (5 PTC4A4− and 10 iPrS−) is either 5 or a multiple of 5, which makes the configuration of the overall structure appear to be a pentagonal arrangement. However, in Ag46, the larger CrO42− with two negative charges acts as a local anion template, giving rise to the larger Ag4 core. Meanwhile, the number of Cl− ions (serving as a global template interiorly), silver atoms (two Ag6 hexagons above and below the equatorial plane and another Ag30 bracelet) or the ligands (six PTC4A4− and twelve iPrS−) on the outer shell correspondingly enlarged from 5 or a multiple of 5 (in Ag37) to 6 or a multiple of 6, resulting in the expansion of the silver nanowheel structure into a hexagonal arrangement. The enclosed CrO42− provides more coordination sites, increases the metal coordination tendency, and is supposed to be the critical feature that allows the nanowheel core to expand significantly.
ESI-MS studies of Ag37 and Ag46
Apart from crystallographic data, electrospray ionization mass spectrometry (ESI-MS) is an alternative characterization method reaching atomic accuracy, which has been widely used to study the solution behavior of metal NCs.21 We characterized Ag37 and Ag46 with a Bruker impact II high definition mass spectrometer using CH3OH/CH2Cl2 as the solvent. As shown in Fig. 3, the most prominent signal containing eight peaks was observed only in the positive mode for Ag37, while three peaks were observed in the negative mode for Ag46 in the m/z range of 3000–12000. The signals at m/z = 4383.535 (1b), 4412.498 (1d), 4441.486 (1f), and 4470.961 (1h) correspond to [NaCl7@Ag37(PTC4A)5(iPrS)x(H2O)(OH)y]2+ (x = 6 ∼ 9, y = 3 ∼ 0; calcd. m/z = 4383.434, 4412.446, 4441.458, and 4470.970), which represents an exchange between iPrS− and OH−. The observed isotopic distribution patterns align with the simulated ones (inset of Fig. 3a). Among them, the species 1h corresponds well with the parent ion peak of Ag37 with the loss of one iPrS− ligand followed by adding one H2O. The remaining peaks were assigned as a series of 36-nuclei silver species due to slight coordination disassociation. The summary of the formulae of the assigned peaks is detailed in Table S2.† Discriminatively, the ESI-MS of Ag46 consists of three predominant peaks carrying two negative charges (2a–2c) as shown in Fig. 3b. After identification, we found that all three peaks belonged to the 44-nuclei silver species but with the loss of the CrO42− anion (Table S3†). This may be due to the fact that the bulky CrO42− is not completely encapsulated in the silver shell and is easily lost after dissolution or during electrospray. These results indicate that Ag37 and Ag46 show a slight dissociation of the surface ligand and Ag atom in solution, but both maintain the basic integrity of the skeleton.
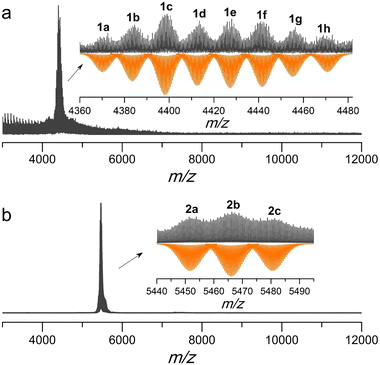 |
| Fig. 3 (a) Positive-ion mode ESI-MS of Ag37 and (b) negative-ion mode ESI-MS of Ag46. Insets: zoom-in experimental (black line) and simulated (orange line) isotope patterns of each labeled species. | |
Dye adsorption performance of Ag46
The problem of water pollution, initiated by the long-term use of dyes, has become a severe threat to human health. Therefore, the efficient removal of dyes from effluents is critical and urgent.22 Adsorption is considered to be one of the most promising methods due to its low cost and high efficiency.23 Because of their hydrophobic and π-electron-rich cavities, calixarenes have recently been introduced as the backbones for constructing metallic and non-metallic containers, successfully inducing these compounds to recognize various guest molecules, such as organic dyes and iodine, as excellent absorbents.24 Studies suggest that the small size of the cavity in calixarenes makes it hard for dyes to bind. On the other hand, calixarene derivatives with extended aromatic groups could bind dyes more efficiently because of the bigger cavity size and also the possibility of π⋯π interactions between aromatic groups and dyes.19,25 From the crystal structures, it is clear that the PTC4A4− ligands adopt a cone conformation and possess a deeper cavity defined by the phenyl group on the upper rim. The average distance between the opposite phenyl groups is 14.7 and 9.9 Å, respectively, and the depth of the cavity is 7.4 Å (Fig. S9 and Table S4†). These cavities are large enough to accommodate suitable guest molecules for inclusion. We envisioned that the PTC4A4−-supported silver NC could fully exert the host–guest interaction of the PTC4A4− ligands on its surface with foreign organic dye molecules. Then, Ag46 is subjected to dye adsorption studies because of its high synthetic yield (50%, at the gram level) and more free interacting sites on the surface available for dye adsorption.
In order to evaluate the adsorption performance of Ag46, three typical organic dyes with different charges and sizes were chosen for measurement at room temperature in the dark: methyl orange (MO), methylene blue (MB), and rhodamine B (RhB) (Table S4†). Among them, both MB and RhB are positively charged, but the size of RhB is larger; MB and MO are similar in size, but MO is negatively charged. Time-dependent UV/vis spectra were collected after adding the freshly prepared crystals of Ag46 (15 mg) to aqueous solutions of MB, RhB, and MO (2.5 mg L−1), respectively (Fig. 4a–c). As evidenced by the changes in the characteristic absorption maximum of the dye aqueous solutions, only the cationic dyes were absorbed by Ag46. This phenomenon was possibly attributed to the electrostatic interaction between the positively charged dyes and the electronegative inner wall of thiacalix[4]arene-based coordination containers (Fig. S10†). Here, the adsorption efficiency for each dye was calculated based on the change in the UV/vis absorbance intensity (100% for MB; 89% for RhB; and 7% for MO within 35 min) (Fig. 4d). Based on selective adsorption of dyes with different charges, Ag46 may have the potential to separate them in bicomponent solutions. Then, the samples of Ag46 were added to the mixed dyes MO/MB, respectively. Obviously, only the absorbance (λmax) of MB centered at 665 nm gradually disappeared, indicating that almost all MB was absorbed from the aqueous solution, with the solution colors varying from green to yellow and the sample of Ag46 changing from brownish red to green-yellow (Fig. 4e). A similar phenomenon was observed, with RhB being selectively separated from the MO/RhB mixture (Fig. 4f).
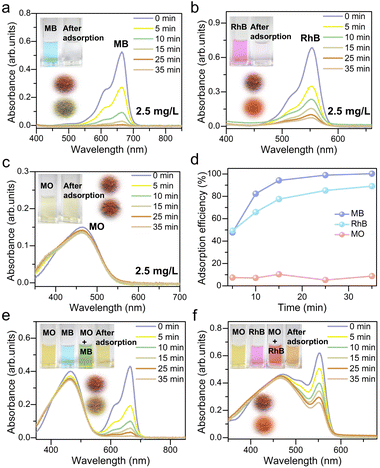 |
| Fig. 4 Temporal evolution of UV-vis absorption spectra of (a) MB, (b) RhB, and (c) MO. (d) The adsorption efficiency of MB, RhB, and MO at the same concentrations. (e) Temporal evolution of UV-vis absorption spectra of MO/MB (concentration ratio of MO and MB = 3 : 1; the concentration of MO = 2.5 mg L−1) and (f) MO/RhB (concentration ratio of MO and RhB = 3 : 1; the concentration of RhB = 2.5 mg L−1). The inset shows the color changes of the aqueous dye solutions and the crystals of Ag46 before (top) and after adsorption (bottom), respectively. | |
The results indicate that Ag46 has highly selective adsorption and separation efficiency for the cationic dyes (MB and RhB) from aqueous solution through the host–guest interaction of the surface cavity, which also inspired us to use Ag46 as the stationary phase of a chromatographic column. MB, RhB, mixed solutions of MO/MB, and MO/RhB were injected into the chromatographic column, respectively. As shown in Fig. 5, MB and RhB are quickly adsorbed onto Ag46, while MO is transported through the column. This successfully separated two kinds of dyes, which could be easily seen with the naked eye (Fig. 5c and d). The above experiments suggest that Ag46 can serve as a column-chromatographic filter for the separation of dyes and provide a basis for further research on the adsorption performance of thiacalix[4]arene-supported silver NCs.
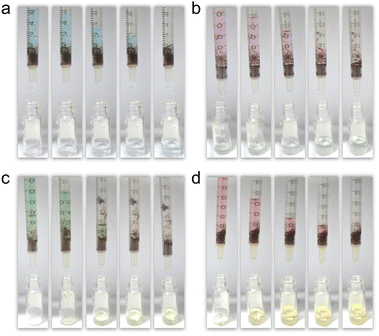 |
| Fig. 5 Photographs of the Ag46-filled column-chromatographic separation process for (a) MB, (b) RhB, (c) MO/MB, and (d) MO/RhB. | |
Molecular docking was conducted to understand and identify the interaction sites between Ag46 and the dyes (Fig. S11 and S12).† These studies support our hypothesis of the inclusion of dye molecules in the cavity of thiacalix[4]arene via noncovalent interactions. Ag46 binds primarily through π⋯π interactions with the MB dye (Fig. 6a and Table S5†), whereas RhB shows π⋯π and C-Halkyl⋯π interactions (Fig. 6b and Table S5†). To the best of our knowledge, this is the first report of metal NCs being utilized for dye-adsorption and exhibiting excellent selectivity for cationic dyes in water.
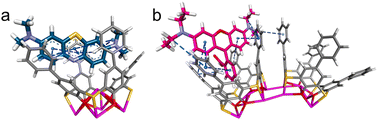 |
| Fig. 6 Various interactions of Ag46 with MB (a) or RhB (b). Colour code: Ag, purple; O, red; S, yellow; C, gray, sea blue, and dark pink; N, blue; H, white. The noncovalent interactions are shown with a dashed light blue line. | |
Conclusions
To summarize, we have presented a systematic strategy for the self-assembly of heteroanions that controls the shape and expansion of the silver nanowheels from Ag37 to Ag46 with the assistance of the macrocyclic PTC4A4− ligand. Structural determination revealed that when using chloride ions as a template, self-assembly resulted in the construction of Ag37, which indicated that chloride ions act as local and global anion templates and are crucial for the organization of a pentagonal silver shell. In contrast in Ag46, when chromate ions along with chloride ions were used as templates, the chromate ions served as the local template, and the chloride ions served as the global template to build a unique hexagonal silver shell. The chromate ions are bulkier and carry more negative charges, which increases their coordination ability with silver atoms, and it is believed to trigger the structural expansion of the silver core and silver shell. Interestingly, the hydrophobic cavity of the PTC4A4− ligand on the surface of Ag46 selectively captures cationic dyes (MB and RhB) through host–guest interaction. Our present work expands the scope of controlled synthesis, expansion, and organization of high-nuclearity silver NCs regulated by a heteroanion template and provides a feasible way of designing NC assemblies with various geometries and functional properties.
Data availability
All experimental procedures, characterization data, and experimental spectra that support the findings of this study are available in the main text and ESI.†
Author contributions
D. S. supervised the project; R. K. G. and L. L. performed the experiments; R. K. G., L. L., Z. W., B. L. H., L. F., Z. Y. G., C. H. T., and D. S. analyzed the data, prepared the figures, and provided conceptual contributions; R. K. G., L. L., and D. S. wrote the manuscript through contributions from all authors. All authors have given their approval to the final version of the manuscript.
Conflicts of interest
There are no conflicts to declare.
Acknowledgements
This work was financially supported by the National Natural Science Foundation of China (Grant No. 52261135637, 22201159, 22171164, 91961105, and 22150410333), the Natural Science Foundation of Shandong Province (No. ZR2022QB008, ZR2020ZD35 and ZR2019ZD45), the Taishan Scholar Project of Shandong Province of China (No. tsqn201812003 and ts20190908), the National Postdoctoral Innovative Talents Support Program (No. BX2021171) and China Postdoctoral Science Foundation (No. 2021M700081).
Notes and references
-
(a) J. P. Wilcoxon and B. L. Abrams, Chem. Soc. Rev., 2006, 35, 1162–1194 RSC;
(b) S. Yamazoe, K. Koyasu and T. Tsukuda, Acc. Chem. Res., 2014, 47, 816–824 CrossRef CAS PubMed;
(c) Y. P. Xie, J. L. Jin, G. X. Duan, X. Lu and T. C. W. Mak, Coord. Chem. Rev., 2017, 331, 54–72 CrossRef CAS;
(d) R. C. Jin, C. J. Zeng, M. Zhou and Y. X. Chen, Chem. Rev., 2016, 116, 10346–10413 CrossRef CAS;
(e) J. F. Corrigan, O. Fuhr and D. Fenske, Adv. Mater., 2009, 21, 1867–1871 CrossRef CAS;
(f) A. Fernando, K. L. D. M. Weerawardene, N. V. Karimova and C. M. Aikens, Chem. Rev., 2015, 115, 6112–6216 CrossRef CAS;
(g) M. Ueda, Z. L. Goo, K. Minami, N. Yoshinari and T. Konno, Angew. Chem., Int. Ed., 2019, 58, 14673–14678 CrossRef.
-
(a) C. J. Zeng, Y. X. Chen, K. Kirschbaum, K. J. Lambright and R. C. Jin, Science, 2016, 354, 1580–1584 CrossRef;
(b) Y. Jin, C. Zhang, X. Y. Dong, S. Q. Zang and T. C. W. Mak, Chem. Soc. Rev., 2021, 50, 2297–2319 RSC;
(c) C. J. Zeng, Y. X. Chen, K. Kirschbaum, K. Appavoo, M. Y. Sfeir and R. C. Jin, Sci. Adv., 2015, 1, e1500045 CrossRef;
(d) Y. Y. Liu, B. K. Najafabadi, M. A. Fard and J. F. Corrigan, Angew. Chem., Int. Ed., 2015, 54, 4832–4835 CrossRef;
(e) K. Yonesato, H. Ito, H. Itakura, D. Yokogawa, T. Kikuchi, N. Mizuno, K. Yamaguchi and K. Suzuki, J. Am. Chem. Soc., 2019, 141, 19550–19554 CrossRef PubMed.
-
(a) Q. M. Wang, Y. M. Lin and K. G. Liu, Acc. Chem. Res., 2015, 48, 1570–1579 CrossRef CAS PubMed;
(b) R. Ge, X. X. Li and S. T. Zheng, Coord. Chem. Rev., 2021, 435, 213787 CrossRef CAS;
(c) D. Rais, J. Yau, D. M. P. Mingos, R. Vilar, A. J. P. White and D. J. Williams, Angew. Chem., Int. Ed., 2001, 40, 3464–3467 CrossRef CAS;
(d) Z. Wang, R. K. Gupta, G. Luo and D. Sun, Chem. Rec., 2020, 20, 389–402 CrossRef CAS PubMed.
-
(a) Z. H. Pan, C. L. Deng, Z. Wang, J. Q. Lin, G. G. Luo and Di Sun, CrystEngComm, 2020, 22, 3736–3748 RSC;
(b) Y. L. Shen, P. Zhao, J. L. Jin, J. Han, C. Liu, Z. Liu, M. Ehara, Y. P. Xie and X. Lu, Dalton Trans., 2021, 50, 10561–10566 RSC.
- Y. P. Xie and T. C. W. Mak, Angew. Chem., Int. Ed., 2012, 51, 8783–8786 CrossRef CAS PubMed.
- J. W. Liu, H. F. Su, Z. Wang, Y. A. Li, Q. Q. Zhao, X. P. Wang, C. H. Tung, D. Sun and L. S. Zheng, Chem. Commun., 2018, 54, 4461–4464 RSC.
-
(a) J. Z. Yan, B. K. Teo and N. F. Zheng, Acc. Chem. Res., 2018, 51, 3084–3093 CrossRef CAS;
(b) J. Yang and R. C. Jin, ACS Mater. Lett., 2019, 1, 482–489 CrossRef CAS;
(c) Q. F. Yao, T. K. Chen, X. Yuan and J. P. Xie, Acc. Chem. Res., 2018, 51, 1338–1348 CrossRef CAS.
-
(a) A. Desireddy, B. E. Conn, J. Guo, B. Yoon, R. N. Barnett, B. M. Monahan, K. Kirschbaum, W. P. Griffith, R. L. Whetten, U. Landman and T. P. Bigioni, Nature, 2013, 501, 399–402 CrossRef CAS;
(b) H. Y. Yang, Y. Wang, H. Q. Huang, L. Gell, L. Lehtovaara, S. Malola, H. Hakkinen and N. F. Zheng, Nat. Commun., 2013, 4, 2422 CrossRef;
(c) C. P. Joshi, M. S. Bootharaju, M. J. Alhilaly and O. M. Bakr, J. Am. Chem. Soc., 2015, 137, 11578–11581 CrossRef CAS PubMed.
-
(a) G. X. Duan, J. Han, B. Z. Yang, Y. P. Xie and X. Lu, Nanoscale, 2020, 12, 1617–1622 RSC;
(b) M. Diecke, C. Schrenk and A. Schnepf, Angew. Chem., Int. Ed., 2020, 59, 14418–14422 CrossRef CAS PubMed.
-
(a) M. M. Zhang, X. Y. Dong, Y. J. Wang, S. Q. Zang and T. C. W. Mak, Coord. Chem. Rev., 2022, 453, 214315 CrossRef;
(b) M. Qu, H. Li, L. H. Xie, S. T. Yan, J. R. Li, J. H. Wang, C. Y. Wei, Y. W. Wu and X. M. Zhang, J. Am. Chem. Soc., 2017, 139, 12346–12349 CrossRef PubMed.
-
(a) W. J. Du, S. Jin, L. Xiong, M. Chen, J. Zhang, X. J. Zou, Y. Pei, S. X. Wang and M. Z. Zhu, J. Am. Chem. Soc., 2017, 139, 1618–1624 CrossRef PubMed;
(b) H. Y. Yang, J. Z. Yan, Y. Wang, H. F. Su, L. Gell, X. J. Zhao, C. F. Xu, B. K. Teo, H. Hakkinen and N. F. Zheng, J. Am. Chem. Soc., 2017, 139, 31–34 CrossRef;
(c) M. J. Alhilaly, M. S. Bootharaju, C. P. Joshi, T. M. Besong, A. H. Emwas, R. Juarez-Mosqueda, S. Kaappa, S. Malola, K. Adil, A. Shkurenko, H. Hakkinen, M. Eddaoudi and O. M. Bakr, J. Am. Chem. Soc., 2016, 138, 14727–14732 CrossRef;
(d) Y. T. Chen, I. S. Krytchankou, A. J. Karttunen, E. V. Grachova, S. P. Tunik, P. T. Chou and I. O. Koshevoy, Organometallics, 2017, 36, 480–489 CrossRef;
(e) H. Shen, K. Kubo, S. Kume, L. M. Zhang and T. Mizuta, Dalton Trans., 2017, 46, 16199–16204 RSC.
- Z. Wang, L. Li, L. Feng, Z. Y. Gao, C. H. Tung, L. S. Zheng and D. Sun, Angew. Chem., Int. Ed., 2022, 61, e202200823 CAS.
- Z. J. Guan, R. L. He, S. F. Yuan, J. J. Li, F. Hu, C. Y. Liu and Q. M. Wang, Angew. Chem., Int. Ed., 2022, 61, e202116965 CAS.
- Z. J. Guan, F. Hu, S. F. Yuan, Z. A. Nan, Y. M. Lin and Q. M. Wang, Chem. Sci., 2019, 10, 3360–3365 RSC.
- Z. J. Guan, J. L. Zeng, Z. A. Nan, X. K. Wan, Y. M. Lin and Q. M. Wang, Sci. Adv., 2016, 2, e1600323 CrossRef.
- Z. Wang, H. F. Su, L. P. Zhang, J. M. Dou, C. H. Tung, D. Sun and L. S. Zheng, ACS Nano, 2022, 16, 4500–4507 CrossRef CAS PubMed.
- Z. Wang, H. F. Su, Y. W. Gong, Q. P. Qu, Y. F. Bi, C. H. Tung, D. Sun and L. S. Zheng, Nat. Commun., 2020, 11, 308 CrossRef CAS PubMed.
- Z. Wang, F. Alkan, C. M. Aikens, M. Kurmoo, Z. Y. Zhang, K. P. Song, C. H. Tung and D. Sun, Angew. Chem., Int. Ed., 2022, 61, e202206742 CAS.
-
(a) R. Kumar, Y. O. Lee, V. Bhalla, M. Kumar and J. S. Kim, Chem. Soc. Rev., 2014, 43, 4824–4870 RSC;
(b) M. Zhao, J. Lv and D. S. Guo, RSC Adv., 2017, 7, 10021–10050 RSC;
(c) N. Frank, A. Dallmann, B. Braun-Cula, C. Herwig and C. Limberg, Angew. Chem., Int. Ed., 2020, 59, 6735–6739 CrossRef.
-
(a) M. Rubio-Martinez, C. Avci-Camur, A. W. Thornton, I. Imaz, D. Maspochbc and M. R. Hill, Chem. Soc. Rev., 2017, 46, 3453–3480 RSC;
(b) A. Chen, X. Kang, S. Jin, W. J. Du, S. X. Wang and M. Z. Zhu, J. Phys. Chem. Lett., 2019, 10, 6124–6128 CrossRef;
(c) B. E. Conn, A. Desireddy, A. Atnagulov, S. Wickramasinghe, B. Bhattarai, B. Yoon, R. N. Barnett, Y. Abdollahian, Y. W. Kim, W. P. Griffith, S. R. J. Oliver, U. Landman and T. P. Bigioni, J. Phys. Chem. C, 2015, 119, 11238–11249 CrossRef.
-
(a) T. K. Chen, Q. F. Yao, R. R. Nasaruddin and J. P. Xie, Angew. Chem., Int. Ed., 2019, 58, 11967–11977 CrossRef;
(b) A. Nag, P. Chakraborty, G. Paramasivam, M. Bodiuzzaman, G. Natarajan and T. Pradeep, J. Am. Chem. Soc., 2018, 140, 13590–13593 CrossRef PubMed;
(c) Y. Niihori, M. Matsuzaki, T. Pradeep and Y. Negishi, J. Am. Chem. Soc., 2013, 135, 4946–4949 CrossRef;
(d) Y. Shichibu, M. Zhang, Y. Kamei and K. Konishi, J. Am. Chem. Soc., 2014, 136, 12892–12895 CrossRef.
-
(a) J. B. Baruah, Coord. Chem. Rev., 2022, 470, 214694 CrossRef;
(b) M. Najafi, S. Abednatanzi, P. G. Derakhshandeh, F. Mollarasouli, S. Bahrani, E. S. Behbahani, P. V. D. Voort and M. Ghaedi, Coord. Chem. Rev., 2022, 454, 214332 CrossRef.
- Y. B. Zhou, J. Lu, Y. Zhou and Y. D. Liu, Environ. Pollut., 2019, 252, 352–365 CrossRef PubMed.
-
(a) C. Z. Sun, T. P. Sheng, F. R. Dai and Z. N. Chen, Cryst. Growth Des., 2019, 19, 1144–1148 CrossRef;
(b) A. J. Gosselin, C. A. Rowland and E. D. Bloch, Chem. Rev., 2020, 120, 8987–9014 CrossRef PubMed.
- P. Lhotak, T. Smejkal, I. Stibor, J. Havlicek, M. Tkadlecova and H. Petrickova, Tetrahedron Lett., 2003, 44, 8093–8097 CrossRef.
Footnote |
† Electronic supplementary information (ESI) available: Synthesis details, IR, UV-vis, and PXRD data, details of the data collection and structure refinements, and crystal data. CCDC 2206151 and 2206152 for Ag46 and Ag37. For ESI and crystallographic data in CIF or other electronic format see DOI: https://doi.org/10.1039/d2sc06436g |
|
This journal is © The Royal Society of Chemistry 2023 |