DOI:
10.1039/D2SC06400F
(Edge Article)
Chem. Sci., 2023,
14, 1912-1918
Synthesis of vinyl-substituted alcohols using acetylene as a C2 building block†
Received
21st November 2022
, Accepted 22nd December 2022
First published on 23rd December 2022
Abstract
Vinyl-substituted alcohols represent a highly useful class of molecular skeletons. The current method typically requires either stoichiometric metallic reagents or preformed precursors. Herein, we report a nickel catalysis-enabled synthesis of vinyl-substituted alcohols via a 5-membered oxa-metallacycle. In this protocol, acetylene, the simplest alkyne and abundant feedstock, is employed as an ideal C2 synthon. The reaction features mild conditions, good functional group tolerance and broad substrate scope. Mechanistic exploration implies that the oxa-metallacycle originated from the cyclometallation of aldehyde and acetylene is the key intermediate for this transformation, which is then terminated by a silane-mediated σ-bond metathesis and subsequent reductive elimination.
As a class of versatile motifs in organic synthesis, vinyl-substituted alcohols (abbreviated as vinyl alcohols) permit a myriad of opportunities for the manipulation and introduction of functional groups as well as construction of complex molecular architectures.1 Therefore, numerous methods have been established to prepare such significant synthetic building blocks (Scheme 1A).2 Among these approaches, alkenylation of aldehydes with vinyl metal is one of the most common strategies to deliver vinyl alcohols (Scheme 1A, path a).3 Despite widespread utilization of this method in organic synthesis, sensitive and stoichiometric organometallic reagents are often required under harsh conditions with poor functional group tolerance. In addition to the alkenylation of aldehydes, direct 1,2-addition of acrolein with organometallic compounds could give the desired vinyl alcohols as well (path b).4 However, the competition between 1,2- and 1,4-addition5 makes this method useless from a practical point of view. Apart from nucleophilic addition of metallic reagents, transition metal-catalyzed allylic substitution with O-nucleophiles represents another important route to obtain vinyl alcohols (path c),6 while it is often plagued with the control of regioselectivities and requirement of pre-synthesized allylic substrates. Taken together, syntheses of vinyl-substituted alcohols via these strategies are limited to either sensitive metallic reagents or allylic precursors. Therefore, developing alternative efficient approaches with easily available reagents to acquire vinyl-substituted alcohols and their derivatives under mild conditions is highly desirable.
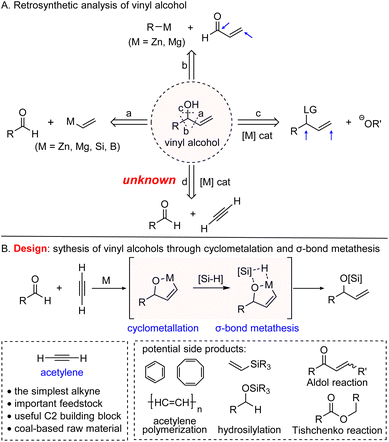 |
| Scheme 1 Synthetic strategies of vinyl alcohols. | |
As the simplest alkyne, acetylene is an inexpensive and abundant feedstock, which has been used as a raw material for bulk vinyl-containing monomer production in the chemical industry.7 While in the fine chemical field, catalytic transformation of this unique C2 unit into high value-added chemicals is underdeveloped.7e,8,9 Despite great efforts being devoted to the development of reductive coupling of substituted alkynes with aldehydes,10 incorporation of acetylene with aldehydes still remains unknown (path d). This may be attributed to the unique properties of acetylene.11 Moreover, the gaseous nature and flammable properties of acetylene make chemists stay away from it.12
Inspired by the fact that substituted allylic alcohols could be provided by reductive coupling of aldehydes with substituted alkynes via an oxa-metallacycle intermediate,10a,h,j we investigated whether it is feasible to employ acetylene and aldehyde through cyclometallation and subsequent silane-mediated σ-bond metathesis to construct silyl-protected vinyl alcohols (Scheme 1B). However, the cyclometallation of aldehyde with acetylene in the presence of silane to obtain key intermediate oxa-metallacycle is a really big challenge due to the following potential competitions: (1) self-polymerization of acetylene,11b,13 (2) direct hydrosilation of aldehyde10c or acetylene and (3) condensation or dimerization of aldehyde (Tischenko reaction and14 Aldol reaction15). More importantly, safety issues concerning explosive and flammable acetylene should not be ignored as well.12b,16
Herein, we report the synthesis of vinyl-substituted alcohols enabled by a nickel-catalyzed reductive coupling of aldehyde with acetylene by utilizing hydrosilane as an efficient terminating reductant. Compared with the previously reported reductive coupling method requiring addition of an alkyne utilizing syringe pump, this protocol only needs slow addition of premixed solution of acetylene and aldehyde dropwise. Both aryl and aliphatic aldehydes with diverse functional groups react smoothly under mild conditions. The robustness of this strategy has also been exhibited by gram-scale reactions and further transformations of the resulting vinyl alcohols into diversely functionalized molecules. The mechanistic studies demonstrate that hydrogen comes from silane, which supports the postulation that the oxa-nickelacycle originated from cyclometallation is terminated by silane-mediated σ-bond metathesis.
Results and discussion
To start our investigation, 4-biphenylcarboxaldehyde 1a and triethylsilane were chosen as model substrates along with acetylene as a vinyl synthon (Table 1). After optimization, we were pleased to find that the use of Ni(cod)2 (20 mol%) and IPr·HCl (40 mol%) as precatalysts, KOtBu (44 mol%) as the base, and triethylsilane (4 equiv.) as the terminating reductant successfully facilitated the formation of desired product 2a in 74% yield at 35 °C (entry 1). No consumption of aldehyde 1a was observed when utilizing Ni(0) generated from reduction of Ni(II) with Zn dust (entry 2). Among several Ni(0) precatalysts, Ni(cod)2 was found to be the most efficient (entry 3, see the ESI for details†). The employment of the 20 mol% Ni(0) precatalyst might be attributed to the potential deactivation of Ni(0) species. The relatively unstable Ni(0) particles might agglomerate to form Ni black during the reaction.17 Ni black is devoid of catalytic activity which leads to the loss of catalytically active Ni(0) species.17a When acetylene was charged in a balloon to conduct the reaction, low yield was afforded with vast amounts of insoluble black residue observed (entry 4), which might be attributed to the competing polymerization of acetylene. Most phosphine- and nitrogen-based ligands were ineffective for this process, while N-heterocyclic carbene (NHC) ligands proved to be effective (see the ESI for details†). Screening of various N-heterocyclic carbene (NHC) ligands showed that IPr provided the best result. It was supposed that the steric effect played a significant role in this reaction because both less sterically hindered ligand IMes and more crowded ligand IAd gave inferior results (entries 5 and 6). As for the base, KOtBu was the best choice for this protocol (entry 7). Changing the solvent to DCE and toluene led to diminished yields (entries 8 and 9). The yield could not be further improved by varying other reaction parameters including the substrate ratio, catalyst loading and temperature (entries 10–13).
Table 1 Optimization of reaction conditionsa

|
Entry |
Deviation from the standard conditions |
Yield (%) |
Reaction conditions: 1a (0.3 mmol), saturated solution of acetylene in THF (0.6 mmol), Et3SiH (1.2 mmol), Ni(cod)2 (20 mol%), IPr·HCl (40 mol%), KOtBu (44 mol%), THF (1.5 mL), 35 °C. The yield was determined by 1H NMR spectroscopy with 4-nitrotoluene as the internal standard.
Isolated yield.
|
1 |
None |
74 (75)b |
2 |
Ni(acac)2/Zn instead of Ni(cod)2 |
0 |
3 |
Ni(cod)DQ instead of Ni(cod)2 |
13 |
4 |
A gaseous acetylene balloon instead of acetylene saturated solution in THF |
18 |
5 |
IMes·HCl instead of IPr·HCl |
17 |
6 |
IAd·HBF4 instead of IPr·HCl |
40 |
7 |
NaOtBu instead of KOtBu |
39 |
8 |
DCE instead of THF |
21 |
9 |
Toluene instead of THF |
32 |
10 |
10 mol% Ni(cod)2 |
34 |
11 |
1 equiv. acetylene |
53 |
12 |
2 equiv. Et3SiH |
49 |
13 |
Room temperature instead of 35 °C |
56 |
With the optimized reaction conditions in hand, the generality of this three-component reaction was then explored (Scheme 2). The reaction demonstrated broad substrate scope and excellent functional group tolerance. For example, in addition to 4-biphenylcarboxaldehyde 1a, a variety of different aryl aldehydes were able to give the desired vinyl alcohols in 36–89% yields (2a–2ab). All the para-, meso-, and ortho-substituted phenyl aldehydes were converted into the corresponding products 2a–c in 75–87% yields. Moderate yields could be obtained when the hydrogen was replaced by OBn or OPh groups (2d–e). The parent benzaldehyde was also a good substrate, providing the desired product 2h in 69% yield. Aryl aldehydes bearing electron-withdrawing groups such as halogens, trifluoromethyl, and trifluoromethoxy were all well tolerated, giving the corresponding products in 46–71% yields (2f, 2i–n). The decreased yields obtained from 2k–l may be ascribed to the competing oxidative addition of the C–X bond (X = Br, I). The reaction offered excellent functional group tolerance; a broad range of synthetically valuable functional groups typically sensitive to the Grignard reagent could be well-tolerated, including ester, nitro, cyano, ketone, amide, and enoate furnishing the target products with functional groups that remained untouched in 35–89% yields (2g, 2o–s, 2u). Sulfone substituted arylaldehyde was also capable of participating in this reaction and gave the expected product 2t as well. The strong affinity of nitrogen to Ni(0) may be responsible for the modest yields obtained from nitrogen-containing aldehydes. It is noteworthy that p-pinacol boronate-substituted benzaldehyde could furnish the product 2v in 71% yield. As pinacol boronate is a highly useful synthetic handle, it might permit access to diverse aryl vinyl alcohol derivatives. Interestingly, when terephthalaldehyde was employed in the reaction, bi-functionalization occurred smoothly to afford the expected di-substituted vinyl alcohol 2w in 53% yield. Benzaldehyde substituted with imidazole was also a suitable substrate, giving the target product 2x in 50% yield. Additionally, the fused aromatic aldehydes could be successfully applied in this transformation as well, leading to the corresponding products 2y–2ab in 35–71% yields. Aliphatic aldehyde, which represents a type of challenging substrate under basic conditions because of the potential self-aldol condensation, could also be converted to aryl vinyl alcohol derivatives successfully. Both primary and secondary aldehydes participated well in this allylation reaction, providing the corresponding products 2ba–bh in 50–77% yields. To further demonstrate the feasibility of this protocol, late-stage modifications using some important aliphatic aldehydes applied for flavor and fragrance,18 such as cyclamen aldehyde,18b lilial,18c floramelon,18d VANDOR B,18e myrac aldehyde,18f ligustrum aldehyde18g and citronellal18h were also successfully accomplished (2ca–cg). The coordination of the C
C bond in the substrates with the Ni center might reduce the catalytic activity which led to relatively low yields. Notably, as a common food additive,18i laurine with a free hydroxyl could be tolerated as well, leading to the corresponding product 2ch in 77% yield.
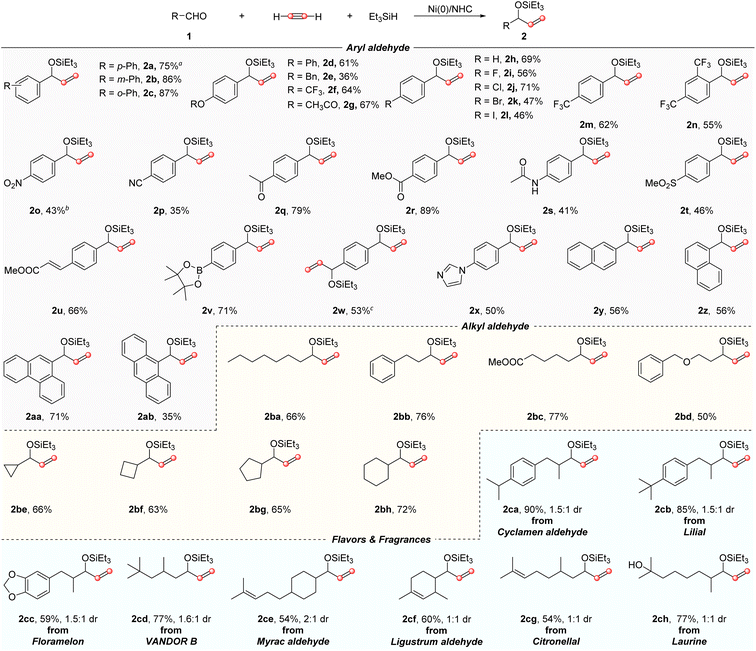 |
| Scheme 2 Substrate scope. Reaction conditions: 1a (0.2 mmol), acetylene (0.4 mmol), Et3SiH (0.8 mmol), Ni(cod)2 (20 mol%), IPr·HCl (40 mol%), KOtBu (44 mol%), THF (1.0 mL), 35 °C. All yields shown are of isolated products. aOn a 0.3 mmol scale. bOn a 0.1 mmol scale. c40 mol% precatalyst, on a 0.1 mmol scale. | |
To showcase the robustness of this method, gram-scale preparation of the vinyl-substituted alcohols was then carried out. As shown in Scheme 3, both aryl vinyl alcohol 3q and aliphatic vinyl alcohol 3ca could be easily synthesized on a 10 mmol scale under slightly modified conditions followed by desilication with TBAF.6a With vinyl alcohols 3q and 3ca in hand, several further transformations were also performed. For example, ketone 4 and epoxide 5 could be produced in 84% and 90% yields through the conventional Heck and epoxidation reactions, respectively. The dehydrative Tsuji–Trost reaction of 3q with TsOH delivered allylic sulfone 6 in 79% yield. Palladium-catalyzed allylation of 3q with 4-tolylboronic acid afforded the coupling product 7. Furthermore, oxidation of the vinyl alcohol 3ca with DMP generated enone 8 in 81% yield which could then be a useful building block to assemble furan 9 in 67% yield via a tandem olefin cross metathesis/acid catalyzed isomerization/cyclization cascade reaction. It should be noticed that the four carbon atoms of furan all come from acetylene. Moreover, ester and boronate groups could also be introduced, furnishing E-homoallylic ester 10 and borate 11 in 70% and 75% yields, respectively.
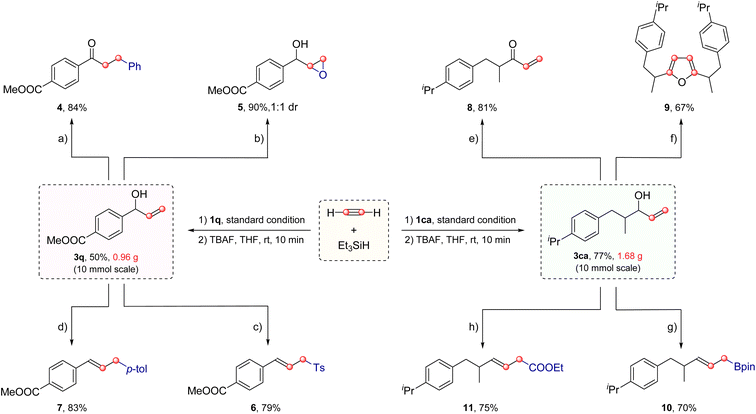 |
| Scheme 3 Gram-scale experiments and synthetic applications of products.19 | |
To better understand this reaction, a series of control experiments were then performed. First, we wondered whether this nickel-catalyzed system was feasible for the substituted alkynes. As shown in Scheme 4a, when terminal aliphatic alkyne, 1-octyne was subjected to the standard conditions, the desired three-component reactions occurred smoothly but gave a mixture of regioisomers 14a and 14a′ in 53% and 24% yield, respectively (eqn (1)). The attempt to assemble phenylacetylene turned out to be a failure and no desired three-component product 14b or 14b′ was obtained (eqn (2)). In addition to the terminal alkynes, internal alkynes were also tested. Similarly, aliphatic 4-octyne was a suitable substrate for this catalytic system, leading to 14c in 65% yield (eqn (3)), while the aromatic diphenylacetylene did not proceed in this catalytic system either (eqn (4)). These outcomes led us to conclude that this nickel-catalyzed three-component reaction seems to be only confined to acetylene or aliphatic alkyne, as both terminal and internal aromatic alkynes did not undergo the transformation. Furthermore, the competition reaction of different alkynes in one flask under standard conditions also suggested that the reaction of acetylene was faster than that of both internal and terminally substituted alkynes because the predominant product was 2a, though 4-octyne-involved product 14c was detected in 10% yield as well (Scheme 4b). To further elucidate the reaction mechanism, deuterium-labeling experiments were conducted (Scheme 4c). Initially, the deuterium (D)-labeled aldehyde d-1a was prepared and submitted to the reaction, and the desired deuterated product d-2a without observable loss of deuterium was isolated in 28% yield (eqn (7)). When deuterated triethylsilane (Et3SiD) was employed in the model reaction, a deuterated product d-2a′ was produced in 25% yield, with 96% D-incorporation at the terminal carbon of the C
C bond in cis-configuration (eqn (8)), which indicated that the silane was the hydrogen donor and validated our hypothesis on silane-mediated σ-bond metathesis.
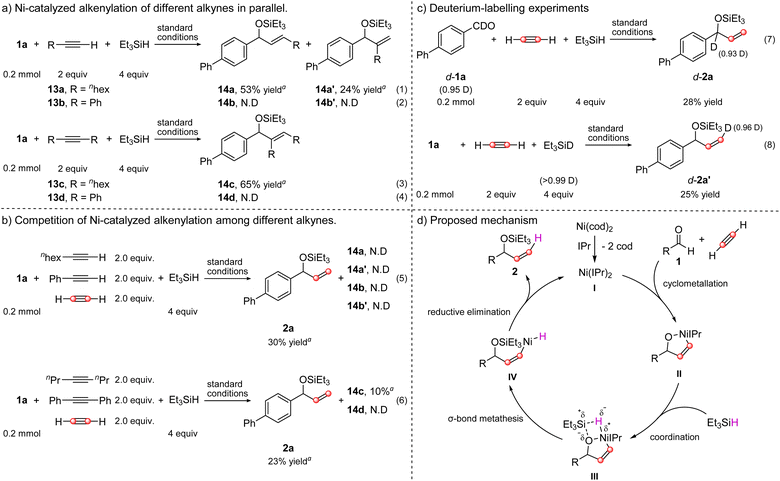 |
| Scheme 4 Control experiments and proposed mechanism. aDetermined by 1H NMR with 4-nitrotoluene as the internal standard. | |
Based on the above control experiments and previous reports,10a,c–i,k–m a plausible mechanism for this protocol is proposed in Scheme 4d. Initial ligand exchange of precatalyst Ni(cod)2 with IPr generates a stable low-valent bis-NHC–Ni species I, which then undergoes cyclometallation of aldehyde with acetylene, resulting in the formation of oxa-nickelacycle II. Subsequent coordination of triethylsilane with oxa-nickelacycle II delivers a transient four-center intermediate III. Cleavage of the Ni–O bond of III occurs via σ-bond metathesis, furnishing the alkenyl nickel intermediate IV. The following reductive elimination of IV produces the desired vinyl-substituted alcohol 2 and regenerates the active nickel species.
Conclusions
In conclusion, we have developed an efficient silane-mediated σ-bond metathesis-enabled synthesis of vinyl-substituted alcohols by nickel catalysis with readily available substrates and abundant acetylene. In this catalytic system, acetylene is incorporated into the vinyl alcohol as a useful C2 building block. This mild synthetic method features good functional tolerance and broad substrate scope, which can be achieved on a gram-scale. The resulting vinyl alcohols are important and versatile scaffolds in organic synthesis, which can be easily transformed into diversely functionalized molecules. Competition experiments between acetylene and other substituted alkynes exhibit the superior reactivity of acetylene. The deuterium-labelling experiments show that the cis-hydrogen at the terminal carbon of the C
C bond is created via silane-mediated σ-bond metathesis and subsequent reductive elimination. It is expected that the application of acetylene in this efficient catalytic transformation might spur interest in the development of novel methodologies employing acetylene as a C2 building block to assemble diverse high value-added molecules.
Data availability
All the data including experimental procedures, NMR, IR, and HRMS, are recorded in the ESI.†
Author contributions
Conceptualization, funding acquisition, resources and supervision were performed or provided by S. Zhu; project administration; investigation and formal analysis were performed by Z. Lin and B. L; validation and data curation were performed by Z. Lin, Y. Wang and S. Li.; the conception of the experiments, discussion of the results and preparation of the manuscript were performed by Z. Lin, B. Liu. and S. Zhu.
Conflicts of interest
There are no conflicts to declare.
Acknowledgements
We appreciate financial support from the National Natural Science Foundation of China (21871096, 22071062 and 22271096).
Notes and references
- For selected reviews, see:
(a) R. Uma, C. Crévisy and R. Grée, Chem. Rev., 2003, 103, 27–52 CrossRef CAS;
(b) M. Bandini, Angew. Chem., Int. Ed., 2011, 50, 994–995 CrossRef CAS;
(c) B. Sundararaju, M. Achard and C. Bruneau, Chem. Soc. Rev., 2012, 41, 4467 RSC;
(d) A. Baeza and C. Nájera, Synthesis, 2013, 46, 25–34 CrossRef;
(e) J. Qu and G. Helmchen, Acc. Chem. Res., 2017, 50, 2539–2555 CrossRef ; For selected examples, see:;
(f) Y. Masuyama, J. P. Takahara and Y. Kurusu, J. Am. Chem. Soc., 1988, 110, 4473–4474 CrossRef;
(g) M. Roggen and E. M. Carreira, J. Am. Chem. Soc., 2010, 132, 11917–11919 CrossRef PubMed;
(h) D. Banerjee, R. V. Jagadeesh, K. Junge, H. Junge and M. Beller, Angew. Chem., Int. Ed., 2012, 51, 11556–11560 CrossRef;
(i) M. Vellakkaran, M. M. S. Andappan and N. Kommu, Green Chem., 2014, 16, 2788–2797 RSC;
(j) S. F. Musolino, O. S. Ojo, N. J. Westwood, J. E. Taylor and A. D. Smith, Chem.–Eur. J., 2016, 22, 18916–18922 CrossRef;
(k) C.-Y. Meng, X. Liang, K. Wei and Y.-R. Yang, Org. Lett., 2019, 21, 840–843 CrossRef;
(l) Y. Gan, H. Hu and Y. Liu, Org. Lett., 2020, 22, 4418–4423 CrossRef;
(m) S. Tang, P. Zhang, C. Wang, Y. Shao and J. Sun, Chem. Commun., 2021, 57, 11080–11083 RSC;
(n) X.-Y. He and Z.-X. Wang, Chem. Commun., 2021, 57, 11988–11991 RSC;
(o) Z.-H. Wu, H.-Y. Wang, H.-L. Yang, L.-H. Wei, T. Hayashi and W.-L. Duan, Angew. Chem., Int. Ed., 2022, e202213904 Search PubMed;
(p) P. Yang, R. X. Wang, Y. Z. Cheng, C. Zheng and S. L. You, Angew. Chem., Int. Ed., 2022, e202213520 Search PubMed;
(q) B. Li, M. Liu, S. U. Rehman and C. Li, J. Am. Chem. Soc., 2022, 144, 2893–2898 CrossRef PubMed.
- For the book on the synthesis of allylic alcohols, see:
(a)
D. M. Hodgson and P. G. Humphreys, in Science of Synthesis: Category 5, Compounds with One Saturated Carbon Heteroatom Bond, ed. J. Clayden, Thieme Chemistry, Stuttgart, 1st edn, 2008, vol. 36, pp. 583–665; For selected reviews on the synthesis of allylic alcohols, see: Search PubMed;
(b) A. Lumbroso, M. L. Cooke and B. Breit, Angew. Chem., Int. Ed., 2013, 52, 1890–1932 CrossRef;
(c) J. Li, X. Zhang, Y. Yao, Y. Gao, W. Yang and W. Zhao, J. Org. Chem., 2022, 87, 6951–6959 CrossRef PubMed.
-
(a) D. Tomita, R. Wada, M. Kanai and M. Shibasaki, J. Am. Chem. Soc., 2005, 127, 4138–4139 CrossRef PubMed;
(b) D. Tomita, M. Kanai and M. Shibasaki, Chem.–Asian J., 2006, 1, 161–166 CrossRef PubMed;
(c) A. M. Deberardinis, M. Turlington and L. Pu, Angew. Chem., Int. Ed., 2011, 50, 2368–2370 CrossRef;
(d) A. Ambrosi, L. Pignataro, C. Zanato and C. Gennari, Eur. J. Org. Chem., 2012, 2012, 144–153 CrossRef.
- C. Elgindy, J. S. Ward and M. S. Sherburn, Angew. Chem., Int. Ed., 2019, 58, 14573–14577 CrossRef PubMed.
-
R. Brückner, in Science of Synthesis: Category 4, Compounds with Two Carbon Heteroatom Bonds, ed. J. Mulzer, Thieme Chemistry, Stuttgart, 1st edn, 2007, vol. 25, pp. 369–404 Search PubMed.
-
(a) I. Lyothier, C. Defieber and E. M. Carreira, Angew. Chem., Int. Ed., 2006, 45, 6204–6207 CrossRef PubMed;
(b) S. Ueno and J. F. Hartwig, Angew. Chem., Int. Ed., 2008, 47, 1928–1931 CrossRef PubMed;
(c) M. Roggen and E. M. Carreira, Angew. Chem., Int. Ed., 2011, 50, 5568–5571 CrossRef.
- For selected reviews, see:
(a) A. B. Trofimov, Curr. Org. Chem., 2002, 6, 1121–1162 CrossRef;
(b) J. Zhang, N. Liu, W. Li and B. Dai, Front. Chem. Sci. Eng., 2011, 5, 514–520 CrossRef;
(c) H. Schobert, Chem. Rev., 2014, 114, 1743–1760 CrossRef PubMed;
(d) I.-T. Trotuş, T. Zimmermann and F. Schüth, Chem. Rev., 2014, 114, 1761–1782 CrossRef;
(e) V. V. Voronin, M. S. Ledovskaya, A. S. Bogachenkov, K. S. Rodygin and V. P. Ananikov, Molecules, 2018, 23, 2442 CrossRef ; For selected examples, see:;
(f) E. Y. Shmidt, N. I. Protsuk, A. M. Vasil'Tsov, A. V. Ivanov, A. I. Mikhaleva and B. A. Trofimov, Chem. Heterocycl. Compd., 2013, 49, 404–407 CrossRef;
(g) T. J. Lin, X. Meng and L. Shi, Appl. Catal., A, 2014, 485, 163–171 CrossRef;
(h) J. Li, H. Zhang, L. Li, M. Cai, Y. Li, D. Xie and J. Zhang, ACS Sustainable Chem. Eng., 2020, 8, 10173–10184 CrossRef;
(i) S. Lü, Z. Wang and S. Zhu, Nat. Commun., 2022, 13, 5001 CrossRef.
-
(a) V. P. Ananikov, M. S. Ledovskaya, V. V. Voronin and K. S. Rodygin, Synthesis, 2022, 54, 999–1042 CrossRef;
(b) D. Scharnagel, I. Escofet, H. Armengol-Relats, M. E. Orbe, J. N. Korber and A. M. Echavarren, Angew. Chem., Int. Ed., 2020, 59, 4888–4891 CrossRef CAS PubMed;
(c) N. A. Sitte, M. Menche, P. Tužina, F. Bienewald, A. Schäfer, P. Comba, F. Rominger, A. S. K. Hashmi and T. Schaub, J. Org. Chem., 2021, 86, 13041–13055 CrossRef CAS PubMed;
(d) B. Yang, S. Lu, Y. Wang and S. Zhu, Nat. Commun., 2022, 13, 1858 CrossRef CAS PubMed;
(e) T. Cheng, B. Liu, R. Wu and S. Zhu, Chem. Sci., 2022, 7604–7609 RSC.
- B. Liu, Z. Lin, Y. Wang, T. Cheng, T. Cao and S. Zhu, CCS Chem., 2022 DOI:10.31635/ccschem.022.202202579.
- For books on the reductive coupling of aldehydes and substituted alkynes, see:
(a)
J. Montgomery and G. J. Sormunen, in Metal Catalyzed Reductive C–C Bond Formation: A Departure from Preformed Organometallic Reagents, ed. M. J. Krische, Springer Berlin Heidelberg, Berlin, Heidelberg, 2007, vol. 279, pp. 1–23 Search PubMed;
(b)
J. Montgomery, in Organometallics in Synthesis: Fourth Manual, ed. B. H. Lipshut, John Wiley & Sons, Inc, 2013, ch. 3, pp. 319–428; For selected reviews on the reductive coupling of aldehydes and substituted alkynes, see: Search PubMed;
(c) Y. Hoshimoto, M. Ohashi and S. Ogoshi, Acc. Chem. Res., 2015, 48, 1746–1755 CrossRef CAS ; For selected examples on the reductive coupling of aldehydes and substituted alkynes, see:;
(d) W.-S. Huang, J. Chan and T. F. Jamison, Org. Lett., 2000, 2, 4221–4223 CrossRef CAS;
(e) K. M. Miller, W.-S. Huang and T. F. Jamison, J. Am. Chem. Soc., 2003, 125, 3442–3443 CrossRef CAS;
(f) G. M. Mahandru, G. Liu and J. Montgomery, J. Am. Chem. Soc., 2004, 126, 3698–3699 CrossRef CAS PubMed;
(g) M. R. Chaulagain, G. J. Sormunen and J. Montgomery, J. Am. Chem. Soc., 2007, 129, 9568–9569 CrossRef CAS PubMed;
(h) S. Ogoshi, T. Arai, M. Ohashi and H. Kurosawa, Chem. Commun., 2008, 1347 RSC;
(i) H. A. Malik, G. J. Sormunen and J. Montgomery, J. Am. Chem. Soc., 2010, 132, 6304–6305 CrossRef CAS PubMed;
(j) P. Liu, P. McCarren, P. H.-Y. Cheong, T. F. Jamison and K. N. Houk, J. Am. Chem. Soc., 2010, 132, 2050–2057 CrossRef CAS;
(k) Y. Cai, J.-W. Zhang, F. Li, J.-M. Liu and S.-L. Shi, ACS Catal., 2019, 9, 1–6 CrossRef;
(l) Y. L. Zheng and M. Ye, Chin. J. Chem., 2020, 38, 489–493 CrossRef CAS;
(m) W.-B. Zhang, G. Chen and S.-L. Shi, J. Am. Chem. Soc., 2022, 144, 130–136 CrossRef CAS PubMed.
-
(a) I. V. Alabugin and B. Gold, J. Org. Chem., 2013, 78, 7777–7784 CrossRef;
(b) E. G. Gordeev, E. O. Pentsak and V. P. Ananikov, J. Am. Chem. Soc., 2020, 142, 3784–3796 CrossRef PubMed.
-
(a) K. S. Erokhin, E. G. Gordeev, D. E. Samoylenko, K. S. Rodygin and V. P. Ananikov, Int. J. Mol. Sci., 2021, 22, 9919 CrossRef PubMed;
(b) A. Williams and D. B. Smith, Chem. Rev., 1970, 70, 267–293 CrossRef.
-
(a) W. Reppe, O. Schlichting, K. Klager and T. Toepel, Justus Liebigs Ann. Chem., 1948, 560, 1–92 CrossRef;
(b) M. Zarshenas, K. Moshkunov, B. Czerwinski, T. Leyssens and A. Delcorte, J. Phys. Chem. C, 2018, 122, 15252–15263 CrossRef.
-
(a) S. Ogoshi, Y. Hoshimoto and M. Ohashi, Chem. Commun., 2010, 46, 3354 RSC;
(b) Y. Hoshimoto, M. Ohashi and S. Ogoshi, J. Am. Chem. Soc., 2011, 133, 4668–4671 CrossRef CAS PubMed;
(c)
R. Mahrwald, in Modern Aldol Reactions, ed. R. Mahrwald, 2004, ch. 15, pp. 327–344 CrossRef.
-
M. Braun, in Modern Aldol Reactions, ed. R. Mahrwald, 2004, ch. 1, pp. 1–61 Search PubMed.
- R. B. Getman, Y.-S. Bae, C. E. Wilmer and R. Q. Snurr, Chem. Rev., 2012, 112, 703–723 CrossRef CAS.
-
(a) L. Nattmann and J. Cornella, Organometallics, 2020, 39, 3295–3300 CrossRef CAS;
(b) I. Buslov, F. Song and X. Hu, Angew. Chem., Int. Ed., 2016, 55, 12295–12299 CrossRef CAS PubMed.
-
(a) D. Ribeaucourt, B. Bissaro, F. Lambert, M. Lafond and J.-G. Berrin, Biotechnol. Adv., 2022, 56, 107787 CrossRef CAS PubMed;
(b) A. M. Api, D. Belsito, S. Biserta, D. Botelho, M. Bruze, G. A. Burton, J. Buschmann, M. A. Cancellieri, M. L. Dagli, M. Date, W. Dekant, C. Deodhar, A. D. Fryer, S. Gadhia, L. Jones, K. Joshi, A. Lapczynski, M. Lavelle, D. C. Liebler, M. Na, D. O'Brien, A. Patel, T. M. Penning, G. Ritacco, F. Rodriguez-Ropero, J. Romine, N. Sadekar, D. Salvito, T. W. Schultz, F. Siddiqi, I. G. Sipes, G. Sullivan, Y. Thakkar, Y. Tokura and S. Tsang, Food Chem. Toxicol., 2020, 138, 111267 CrossRef CAS;
(c) R. Ramachanderan and B. Schaefer, ChemTexts, 2019, 5, 11 CrossRef;
(d) S. C. Rastogi, S. Heydorn, J. D. Johansen and D. A. Basketter, Contact Dermatitis, 2001, 45, 221–225 CrossRef CAS PubMed;
(e) M. Schroeder, M. Mathys, N. Ehrensperger and M. Büchel, Chem. Biodiversity, 2014, 11, 1651–1673 CrossRef CAS;
(f) A. M. Api, D. Belsito, D. Botelho, M. Bruze, G. A. Burton, J. Buschmann, M. A. Cancellieri, M. L. Dagli, M. Date, W. Dekant, C. Deodhar, A. D. Fryer, L. Jones, K. Joshi, M. Kumar, A. Lapczynski, M. Lavelle, I. Lee, D. C. Liebler, H. Moustakas, M. Na, T. M. Penning, G. Ritacco, J. Romine, N. Sadekar, T. W. Schultz, D. Selechnik, F. Siddiqi, I. G. Sipes, G. Sullivan, Y. Thakkar and Y. Tokura, Food Chem. Toxicol., 2022, 161, 112859 CrossRef CAS;
(g) L. Chen, N. Chen, Q. He, Q. Sun and W. C. Zeng, J. Food Sci., 2021, 86, 1114–1123 CrossRef CAS;
(h) L. Jiang and K. Kubota, J. Agric. Food Chem., 2004, 52, 4197–4203 CrossRef CAS PubMed;
(i) A. M. Api, F. Belmonte, D. Belsito, S. Biserta, D. Botelho, M. Bruze, G. A. Burton, J. Buschmann, M. A. Cancellieri, M. L. Dagli, M. Date, W. Dekant, C. Deodhar, A. D. Fryer, S. Gadhia, L. Jones, K. Joshi, A. Lapczynski, M. Lavelle, D. C. Liebler, M. Na, D. O'Brien, A. Patel, T. M. Penning, G. Ritacco, F. Rodriguez-Ropero, J. Romine, N. Sadekar, D. Salvito, T. W. Schultz, I. G. Sipes, G. Sullivan, Y. Thakkar, Y. Tokura and S. Tsang, Food Chem. Toxicol., 2019, 134, 111024 CrossRef CAS.
-
(a) J. Yu, X. Chang, R. Ma, Q. Zhou, M. Wei, X. Cao and X. Ma, Eur. J. Org. Chem., 2020, 2020, 7238–7242 CrossRef CAS;
(b) P. Sjo and A. J. Aasen, Acta Chem. Scand., 1993, 47, 486 CrossRef CAS;
(c) W. Su, T.-T. Wang, X. Tian, J.-R. Han, X.-L. Zhen, S.-M. Fan, Y.-X. You, Y.-K. Zhang, R.-X. Qiao, Q. Cheng and S. Liu, Org. Lett., 2021, 23, 9094–9099 CrossRef CAS;
(d) T. J. Donohoe and J. F. Bower, Proc. Natl. Acad. Sci. U. S. A., 2010, 107, 3373–3376 CrossRef CAS;
(e) H. Tsukamoto, T. Uchiyama, T. Suzuki and Y. Kondo, Org. Biomol. Chem., 2008, 6, 3005 RSC;
(f) S. Guo, D. I. Abusalim and S. P. Cook, J. Am. Chem. Soc., 2018, 140, 12378–12382 CrossRef CAS;
(g) W. Wei, L. Liao, T. Qin and X. Zhao, Org. Lett., 2019, 21, 7846–7850 CrossRef CAS PubMed.
|
This journal is © The Royal Society of Chemistry 2023 |