DOI:
10.1039/D2SC05222A
(Edge Article)
Chem. Sci., 2023,
14, 5099-5105
An Fe complex for 19F magnetic resonance-based reversible redox sensing and multicolor imaging†
Received
19th September 2022
, Accepted 14th April 2023
First published on 14th April 2023
Abstract
We report a first-in-class responsive, pentafluorosulfanyl (–SF5)-tagged 19F MRI agent capable of reversibly detecting reducing environments via an FeII/III redox couple. In the FeIII form, the agent displays no 19F MR signal due to paramagnetic relaxation enhancement-induced signal broadening; however, upon rapid reduction to FeII with one equivalent of cysteine, the agent displays a robust 19F signal. Successive oxidation and reduction studies validate the reversibility of the agent. The –SF5 tag in this agent enables ‘multicolor imaging’ in conjunction with sensors containing alternative fluorinated tags and this was demonstrated via simultaneous monitoring of the 19F MR signal of this –SF5 agent and a hypoxia-responsive agent containing a –CF3 group.
Introduction
Antioxidants, or reducing agents, play a pivotal role in maintaining biological redox balance and counteracting oxidative stress. In a biological setting, common antioxidants include ascorbic acid, which reduces nitrite ions to nitric oxide in the stomach and protects agains reactive oxygen species (ROS); vitamin E, which scavenges free radicals formed during metabolism; and thiol-containing molecules. These thiols, including cysteine and glutathione, help maintain redox balance through their ability to be reversibly oxidized to form disulfides. Reductive stress is a condition in which the cellular redox balance is shifted to a more reducing state. For example, reducing environments are associated with cellular hypoxia, impair signalling functions, promote mitochondrial dysfunction, increase apoptosis, and decrease cell survival.1,2 Reductive stress is associated with a number of pathologies, including muscular dystrophy, cancer, Alzheimer's disease, rheumatoid arthritis, and alcohol abuse.3,4
Many techniques currently exist to detect biological reductants and reducing environments, including positron emission tomography (PET)5,6 and fluorescence imaging.7,8 While these are powerful techniques, PET requires the use of ionizing radiation and fluorescence imaging has limited depth penetration for in vivo imaging.9 Thus, alternative techniques that can be readily applied and minimize the use of radioactive materials for in vivo sensing are needed. Traditionally, 1H magnetic resonance imaging (MRI) is used to obtain anatomical and physiological images with high temporal resolution and depth penetration without the use of ionizing radiation; however, abundant endogenous 1H signals deriving from water and lipids results in low imaging specificity. As an alternative, fluorine (19F) MRI provides the same excellent depth penetration, and importantly, higher specificity as there is no detectable endogenous fluorine present in the body. Additionally, fluorine-19 is 100% isotopically abundant, and contains high MR receptivity that nears that of 1H (83% of 1H).10 In addition to its generally favorable magnetic resonance properties, the 19F nucleus can display MR signals over a broad range of chemical shifts (>350 ppm).11 A major advantage of molecular MR imaging agents is the exquisite ability to design selective analyte-responsive agents. However, this is partially counter-balanced by high limits of detection on the order of 10−4 M or more, which is a barrier to the application of small molecule agents. Regardless, elaborating new mechanisms for MR-based sensing with fluorinated small molecules will inform the development of MR sensors with much greater fluorine density (polymers and other macromolecules, nanoparticles) that provide the fluorine concentrations applicable to in vivo detection.
A number of transition metals and lanthanides have been used for redox sensing using magnetic resonance techniques including EuII/III, MnII/III, FeII/III, CoII/III, and CuI/II.12–37 In order to monitor dynamic redox events in biology, a reversible sensing agent is desirable and this requires a ligand that can effectively cage and sequester a metal ion in two oxidation states. Interest in the use of FeII/III has increased due to the superior biocompatibility of iron relative to other redox active metals and the ability to achieve redox reversibility in biological contexts.10,30–35 From a magnetic resonance perspective, the differences in electronic relaxation times of the different oxidation states lead to modulation of MR properties, with high spin FeIII complexes exhibiting substantial 19F MR signal attenuation due to strong paramagnetic relaxation enhancement (PRE) and FeII complexes exhibiting robust and detectable 19F MR signals due to weak PRE. To the best of our knowledge, while Fe complexes have been reported for 19F MRI applications,38–45 this is the first demonstration of a reversible, redox-responsive Fe complex for 19F MR biosensing.
Most small molecule 19F MRI agents utilize –CF3 containing moieties as 19F tags; however, the large ppm range that is available for the 19F nucleus opens up opportunities to design imaging agents with chemical shift values that can be differentiated via chemical shift-specific magnetic resonance imaging, enabling ‘multicolor’ (or chemical shift-selective) imaging of multiple species containing distinct 19F frequencies within the same specimen. The pentafluorosulfanyl (–SF5) moiety is promising in this respect due to its biostability46 and distinct chemical shift relative to –CF3 (∼+60 ppm for –SF5, ∼−70 ppm for –CF3). One disadvantage of an –SF5 tag is the presence of inequivalent fluorine atoms that reduce signal density and result in doublet (equatorial fluorine atoms) and quintet (axial fluorine atom) signals. Regardless, the value of the –SF5 tag has been successfully demonstrated in drug tracking studies,47 though it has never been incorporated for biosensing applications as will be described.
Herein, we report the first Fe-based redox responsive 19F MRI agent, FeIIIDO3ASF5 (Scheme 1), capable of reversibly detecting reducing environments. This agent contains a novel –SF5 tag that has potential to be used simultaneously alongside a –CF3 containing agent for multicolor 19F MR imaging.14
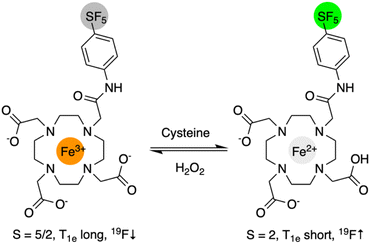 |
| Scheme 1 Design strategy for reversible redox responsive 19F MRI probe exploiting FeII/III redox chemistry. The initial “off” 19F signal “turns-on” following reduction of FeIII to FeII by cysteine and “turns-off” following oxidation by H2O2. An –SF5 tag provides a means to simultaneously image these agents with –CF3 and other 19F-tagged species. | |
Results and discussion
DO3ASF5 was synthesized from tBuDO3A, chloroacetyl chloride, and 4-(pentafluorosulfanyl)aniline in good yield (48%) following tert-butyl ester deprotection (Scheme S1†). The ligand was complexed with anhydrous FeCl3 to obtain FeIIIDO3ASF5 (75%) or with aqueous Fe(BF4)2 to obtain FeIIDO3ASF5 (64%). DO3ASF5 and FeII/IIIDO3ASF5 were purified using C18 reverse-phase chromatography. Full details are provided in the ESI.† Previous reports have shown that FeIIIDO3A complexes form neutral seven-coordinate complexes derived from three carboxylate and four nitrogen donors.48 We propose that FeIIIDO3ASF5 forms a similar neutral structure due to its low solubility in water and the one proton difference seen by mass spectrometry between FeIIIDO3ASF5 and FeIIDO3ASF5. These observations are reinforced by a remarkable increase in water solubility of FeIIDO3ASF5, which would have a water solubilizing free carboxylic acid and only two carboxylate donors directly bound to the metal. The possibility of the amide group also coordinating cannot be ruled out since seven- and eight-coordinate FeII complexes have been reported.49–51
Cyclic voltammetry of 1 mM FeIIIDO3ASF5 in 0.1 M KCl was conducted to characterize the complex's ability to switch between the FeIII and FeII oxidation states. FeIIIDO3ASF5 displayed a half wave potential, E1/2, at 389 mV vs. normal hydrogen electrode (NHE, Fig. 1A) with an anodic peak potential, Epa, at 358 mV vs. NHE and a cathodic peak potential, Epc, at 420 mV vs. NHE, consistent with a reversible redox process. This potential is similar to an FeIIIDOTA peptide conjugate reported in the literature (E1/2 = 396 mV vs. NHE).52 With an E1/2 of 389 mV, both the ferric and ferrous oxidation states of FeDO3ASF5 should be biologically accessible, as this potential is near that of the one electron reduction of H2O2 at pH 7 (380 mV vs. NHE).53 Compared to reversible redox responsive agents for 1H MRI (FePyC3A E1/2 = 230 mV vs. NHE,31 MnHBET E1/2 = 356 mV vs. NHE),281H/19F MRI (MnHTFBED E1/2 = 250 mV vs. NHE),29 and CEST (CoTPT, E1/2 = −107 mV vs. NHE),21FeIIIDO3ASF5 has a slightly higher potential, indicating our agent favors the reduced state more than these previously reported agents. The cyclic voltammograms of FeIIIDO3ASF5 were similar when the scan speed varied from 50–400 mV s−1, demonstrating the complex is stable and exhibits reversible redox behavior within the given scan speed range.
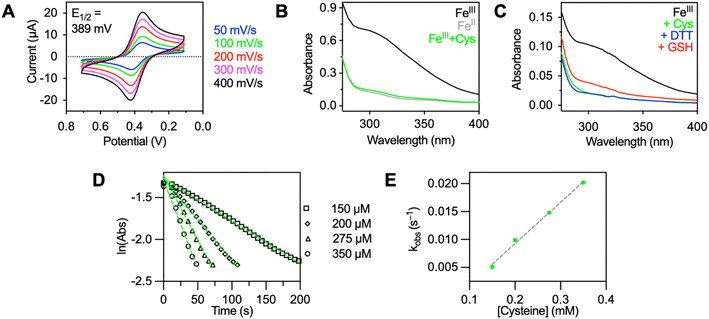 |
| Fig. 1 (A) Cyclic voltammograms of 1 mM FeIIIDO3ASF5 in 0.1 M KCl recorded at various scan speeds (50–400 mV s−1). (B) UV-vis absorption spectra of 100 μM FeIIIDO3ASF5 in pH 7.4 buffer (black), 100 μM of FeIIDO3ASF5 in pH 7.4 buffer (gray), and 100 μM FeIIDO3ASF5 in pH 7.4 buffer generated in situ from FeIIIDO3ASF5 with one equivalent of cysteine (green). (C) UV-vis absorption spectra of 25 μM FeIIIDO3ASF5 in deoxygenated pH 7.4 buffer (black) with five equivalents cysteine after five minutes (blue), five equivalents DTT after five minutes (green), and five equivalents of GSH after ten minutes (red). (D) The natural log of the change of absorbance at 310 nm vs. time of the reduction of 25 μM FeIIIDO3ASF5 with 150–350 μM of cysteine. Slopes of linear regression fits correspond to the pseudo first-order reaction rates, kobs. (E) Plot of kobsvs. concentration of cysteine, slope of linear regression corresponds to second order rate constant. 5 mM HEPES (pH 7.4) with 10 mM KNO3 was used for all UV-vis experiments. | |
The absorption spectrum for FeIIIDO3ASF5 displays a characteristic charge-transfer band (extinction coefficient, ε = 3280 M−1 cm−1) at 310 nm (Fig. 1B, black trace). The FeIIDO3ASF5 complex displays a lower absorbance at 310 nm relative to the FeIII complex (Fig. 1B, gray trace). Therefore, we performed an initial screen of different reducing agents and monitored the FeIII to FeII change using UV-vis spectroscopy. Cysteine, dithiothreitol (DTT), and glutathione (GSH) were selected since they are either commonly used in biological assays (DTT) or because they are biologically relevant agents (cysteine and GSH) that have been shown to reduce other metal MRI agents.16,54 The initial screening of the three reducing agents showed that DTT and cysteine caused the disappearance of the absorbance peak at 310 nm within 5 minutes. This disappearance is assigned to the reduction of the FeIII complex to FeII since the resulting absorbance matches the spectrum of FeIIDO3ASF5 (Fig. 1B and C). In contrast, when 5 equivalents of GSH was used, the reduction was incomplete after 10 minutes (Fig. 1C). However, full reduction was observed when the GSH concentration was increased to 25 equivalents (Fig. S1†). Notably, FeIIIDO3ASF5 was reduced within five minutes by just one equivalent of cysteine (green trace, Fig. 1B), an improvement over our previously reported macrocyclic CuII-based sensor that required three equivalents of cysteine for full reduction.16
Given the promising reduction results and the biological relevance of cysteine, the kinetics of cysteine-mediated reduction were measured via UV-vis (Fig. S2†). The measurements were determined under pseudo-first order conditions with excess (6–14-fold) cysteine in HEPES buffer (pH 7.4) (Fig. 1D). The reduction is first-order in cysteine and gives a second-order rate constant of k = 74 M−1 s−1 (Fig. 1E). This rate constant is ∼50-fold faster than the redox activated 1H agent, FePyC3A. This is consistent with FeIIIDO3ASF5 having a higher E1/2 (389 mV compared to 230 mV vs. NHE).31
We further characterized these complexes by measuring their solution magnetic moments (μeff, Evans NMR method).55,56FeIIIDO3ASF5 exhibited a μeff of 5.7, consistent with a high spin, S = 5/2 FeIII species. With this spin state, we expect strong PRE-induced signal attenuation of the –SF5 tag in this FeIII complex, resulting in the oxidized state being “off” in 19F MR measurements. FeIIDO3ASF5 had a μeff of 4.5 consistent with a high spin S = 2 species.57 While containing four unpaired electrons, the electronic relaxation times of high spin FeII species are typically very short, making them inefficient for PRE.10 Thus we expect to observe significantly less signal broadening for FeIIDO3ASF5 compared to the FeIII complex.
The 19F NMR spectrum of 1 mM FeIIIDO3ASF5 in deoxygenated pH 7.4 HEPES (Fig. S3†) contained a severely broadened peak centered at +63.7 ppm with a very low signal-to-noise ratio (SNR). On the other hand, the 19F NMR spectrum of 1 mM FeIIDO3ASF5 contained a doublet at +63.4 ppm (Fig. S4†), similar to ligand DO3ASF5, which displayed a doublet at +63.2 ppm. The quintet of FeIIDO3ASF5 was not visible even at concentrations as high as 2.5 mM, and therefore, our discussion will focus on the doublet. To confirm that the reduction can be monitored via19F NMR, 5 equivalents of cysteine were introduced to a sample of FeIIIDO3ASF5, which caused the broadened signal to sharpen and increase in intensity, resulting in a doublet at +63.4 ppm, equivalent to that of FeIIDO3ASF5 (Fig. S5†). The relaxation times for FeIIIDO3ASF5 alone could not be measured due to the severely broadened nature of its 19F NMR peaks. Given this, the 19F longitudinal (T1) and transverse (T2) relaxation times of purified FeIIDO3ASF5 and in situ generated FeIIDO3ASF5 following reduction of FeIIIDO3ASF5 were measured at room temperature. FeIIDO3ASF5 had T1 and T2 values of 155 and 6.3 ms, respectively. The relaxation times measured for in situ generated FeIIDO3ASF5 (FeIIIDO3ASF5 + 5 equivalents DTT) were in strong agreement (T1 = 152 ms, T2 = 5.7 ms).
We next investigated the ability of our complex to reversibly detect environmental redox changes. A 0.5 mM sample of FeIIIDO3ASF5 in deoxygenated HEPES was subjected to multiple reduction and oxidation cycles using cysteine and H2O2, respectively (Fig. 2). Initially, the 0.5 mM sampled displayed no 19F MR signal (Fig. 2B), exhibited an absorption spectrum consistent with the FeIII species (Fig. 2A), and had the deprotonated FeIIIDO3ASF5 mass (ESI+m/z = 659). After adding one equivalent of cysteine, a 19F doublet appeared at +63.4 ppm (Fig. 2B), the FeIII was fully reduced to FeII according to UV-vis (Fig. 2A), and the protonated FeIIDO3ASF5 mass was observed (ESI+m/z = 660). The same sample was reacted with 10 equivalents of H2O2, regenerating FeIIIDO3ASF5 which caused a broadening in the 19F NMR signal (Fig. 2B), a reappearance of the FeIII charge-transfer band (Fig. 2A), and a compound with an m/z of 659. This entire process was repeated multiple times with similar results. Oxidation from FeII to FeIII with H2O2 occurs rapidly, with complete conversion occurring within 5 minutes in the presence of as low as one equivalent of H2O2 as monitored by UV-vis (Fig. S6†).
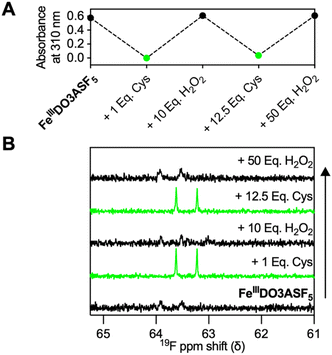 |
| Fig. 2 Repeated reduction and oxidation of FeIIIDO3ASF5 analyzed via (A) UV-vis (0.1 mM complex, absorbance value at 310 nm) and (B) 19F NMR (0.5 mM) using cysteine (“Cys”) and H2O2 in 5 mM HEPES buffer (pH 7.4) with 10 mM KNO3. | |
The kinetic and chemical stabilities of FeIIDO3ASF5 and FeIIIDO3ASF5 were then examined under multiple conditions. The stability of FeIIIDO3ASF5 was studied in the presence of various biologically relevant metal salts, human serum, and different pH values using UV-vis spectroscopy or 19F NMR. The stability of FeIIIDO3ASF5 (100 μM) in the presence of different metal salts (100 mM NaCl, 100 mM KCl, 10 mM CaCl2, 10 mM MgCl2, and 100 μM ZnCl2) was monitored before and after incubation by UV-vis (Fig. S7†). The results showed that FeIIIDO3ASF5 has high stability with no significant absorbance changes observed after 24 h of incubation at 37 °C. Given that FeIIIDO3ASF5 has a E1/2 = 389 mV vs. NHE, it may be sensitive to biological reductants while circulating through the body causing the complex to exist in the FeII state. If this is the case, the FeIIDO3ASF5 complex will dominate, and no selective “turn-on” will be possible. To evaluate this possibility, we monitored FeIIIDO3ASF5 in human serum (HS) by 19F NMR to estimate its redox sensitivity in that environment. No 19F NMR signal changes were observed for a solution of FeIIIDO3ASF5 in 80% HS (20% D2O) or 8% HS (20% D2O) in 1X Minimum Essential Medium (MEM) after incubating at 37 °C for 24 h (Fig. S8 and S9†). FeIIDO3ASF5 and FeIIIDO3ASF5 were also stable under acidic (pH 6) conditions as evaluated by 19F NMR (Fig. S10–S12†). The stability of FeIIDO3ASF5 under acidic conditions matched well with the in situ generated FeIIDO3ASF5 (Fig. S11 and S12†). The stability of FeIIDO3ASF5 with metal cations was determined with 19F NMR due to the low absorbance at wavelengths >300 nm of FeIIDO3ASF5 (Fig. S13–S17†). Similar to the FeIII complex, FeIIDO3ASF5 showed good stability in the presence of abundant metal cations. The air stability of FeIIDO3ASF5 was also evaluated in HEPES buffer by UV-vis. Upon exposure to atmospheric oxygen, only minimal absorbance changes were observed over 18 h (Fig. S18†) demonstrating that the complex is relatively stable to oxygen following reduction.
We measured the 1H longitudinal relaxivity (r1) and transverse relaxivity (r2) of FeIIIDO3ASF5 at 60 MHz to gauge the potential presence of an inner sphere water molecule in this complex. The r1 and r2 were found to be 0.37 mM−1 s−1 and 0.39 mM−1 s−1, respectively. The r1 of FeIIIDO3ASF5 is ∼3–22-fold less than many of the previously reported r1 values for Fe(III) relaxation agents.58 These lower values are likely because the metal center is coordinatively saturated by the macrocyclic ligand framework and contains no exchangeable protons, which leaves outer-sphere proton relaxation as the only possible mechanism.59 This coordinatively saturated structure is consistent with the observed high kinetic stability of the complexes.
To validate our –SF5 labelled agent's ability to “turn-on” and be monitored in concert with a –CF3 labelled agent, FeIIIDO3ASF5 and previously reported hypoxia sensor CuATSM-F3 (Fig. S19†)14 were subjected to 19F MRI using a 7 T scanner. Samples were imaged both at the –SF5 doublet frequency (282.588 MHz) and the –CF3 frequency (282.550 MHz). As shown in Fig. 3, 3 mM FeIIIDO3ASF5 only provides a signal at the –SF5 frequency when reduced with one equivalent of cysteine (SNR = 25.38); no signal is seen in this sample at the –CF3 frequency (SNR = 1.99). CuATSM-F3 was imaged alongside FeIIIDO3ASF5 to show the agents will only provide signals when reduced and imaged using their respective imaging frequency. Specifically, 5 mM CuATSM-F3 displayed a signal when reduced with one equivalent of sodium dithionite (Na2S2O4) using the –CF3 frequency (SNR = 28.92) and no signal when imaged at the –SF5 frequency (SNR = 1.26). We note that CuATSM-F3 is unable to provide a “turn-on” response to cysteine, as more reducing conditions typically associated with severe hypoxia are needed to activate this agent (Fig. S20†). The reversibility of FeIIIDO3ASF5 can also be monitored using MR imaging (Fig. S21†) as initially no signal is observed for 3 mM FeIIIDO3ASF5 (SNR = 2.04), which then “turns-on” with one equivalent of cysteine (SNR = 25.38), and “turns-off” with hydrogen peroxide (SNR = 3.68). Finally, due to the shorter T1 of FeIIDO3ASF5, using a rapid acquisition with relaxation enhancement (RARE) pulse sequence we were able to triple the number of averages for the –SF5 image compared to the –CF3 image. This resulted in the Fe complex displaying a similar SNR when compared to reduced CuATSM-F3 even though the fluorine concentration was smaller (12 mM 19F for FeIIIDO3ASF5 and 15 mM 19F for CuATSM-F3).
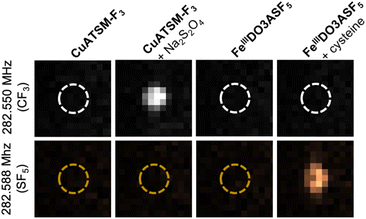 |
| Fig. 3
19F MRI phantoms of 5 mM CuATSM-F3 in the presence and absence of Na2S2O4 and 3 mM FeIIIDO3ASF5 in the presence and absence of cysteine. Images were obtained at both the –CF3 (282.550 MHz) and –SF5 (282.588 MHz) frequencies. Acquisition time for all images was 15 minutes. | |
Conclusions
In conclusion, FeIIIDO3ASF5 is a redox-responsive 19F MR agent that can undergo reversible redox chemistry and be detected using the distinct 19F frequency associated with the biostable –SF5 moiety. Conversion from a high spin S = 5/2 FeIII species to a high spin S = 2 FeII species following cysteine addition provides a mechanism for MR signal “turn-on”. Oxidation following addition of H2O2 provides a mechanism for signal “turn-off”. While these species are physiologically present at micromolar concentrations or below in the extracellular space, and thus may be difficult for detection by MRI, previous work in MRI-based redox imaging suggests a huge potential for monitoring redox environments associated with inflammation or hypoxia using MR imaging agents.31 In proof-of-concept experiments, we demonstrate that this complex can be imaged in concert with the –CF3 tagged hypoxia-responsive agent CuATSM-F3, opening up possibilities for multiplexed biosensing using 19F MR. Ongoing studies include investigating the effects of ligand structure on Fe redox reactivity and biosensing properties and further development of agents with unique tags for multicolor imaging.
Data availability
This data is archived in the Texas Data Repository, https://dataverse.tdl.org/dataverse/emilyque/.
Author contributions
RTK, RTR, and ELQ designed experiments. RTK, RTR, and DJC performed experiments and characterized compounds. RTK, RTR, DJC, and ELQ all contributed to data analysis and manuscript writing.
Conflicts of interest
There are no conflicts of interest to declare.
Acknowledgements
This work was supported by the National Science Foundation (1945401) (ELQ), the Welch Foundation (F-1883) (ELQ), a UT Austin Graduate Student Summer Fellowship (RTK), and a UT Austin Provost's Graduate Excellence Fellowship (DJC). We acknowledge the Biomedical Imaging Center at UT Austin for access to their facilities and Que Lab members for helpful discussions.
References
- D. E. Handy and J. Loscalzo, Responses to reductive stress in the cardiovascular system, Free Radical Biol. Med., 2017, 109, 114–124 CrossRef CAS PubMed.
- W. Xiao and J. Loscalzo, Metabolic Responses to Reductive Stress, Antioxid. Redox Signaling, 2020, 32, 1330–1347 CrossRef CAS PubMed.
- I. Pérez-Torres, V. Guarner-Lans and M. E. Rubio-Ruiz, Reductive Stress in Inflammation-Associated Diseases and the Pro-Oxidant Effect of Antioxidant Agents, Int. J. Mol. Sci., 2017, 18, 2098 CrossRef PubMed.
- R. Masia, W. J. McCarty, C. Lahmann, J. Luther, R. T. Chung, M. L. Yarmush and G. Yellen, Live cell imaging of cytosolic NADH/NAD(+) ratio in hepatocytes and liver slices, Am. J. Physiol.: Gastrointest. Liver Physiol., 2018, 314, G97–G108 CrossRef PubMed.
- A. L. Vāvere and J. S. Lewis, Cu-ATSM: a radiopharmaceutical for the PET imaging of hypoxia, Dalton Trans., 2007, 4893–4902, 10.1039/b705989b.
- J. J. Vaquero and P. Kinahan, Positron Emission Tomography: Current Challenges and Opportunities for Technological Advances in Clinical and Preclinical Imaging Systems, Annu. Rev. Biomed. Eng., 2015, 17, 385–414 CrossRef CAS PubMed.
- Y. Wang, X. Han, X. Zhang, L. Zhang and L. Chen, A high-selectivity fluorescent probe for hypoxia imaging in cells and a tumor-bearing mouse model, Analyst, 2020, 145, 1389–1395 RSC.
- X. Tian, Z. Li, Y. Sun, P. Wang and H. Ma, Near-Infrared Fluorescent Probes for Hypoxia Detection via Joint Regulated Enzymes: Design, Synthesis, and Application in Living Cells and Mice, Anal. Chem., 2018, 90, 13759–13766 CrossRef CAS PubMed.
- M. L. James and S. S. Gambhir, A molecular imaging primer: modalities, imaging agents, and applications, Physiol. Rev., 2012, 92, 897–965 CrossRef CAS PubMed.
- D. Xie, M. Yu, R. T. Kadakia and E. L. Que, 19F Magnetic Resonance Activity-Based Sensing Using Paramagnetic Metals, Acc. Chem. Res., 2020, 53, 2–10 CrossRef CAS PubMed.
- I. Tirotta, V. Dichiarante, C. Pigliacelli, G. Cavallo, G. Terraneo, F. B. Bombelli, P. Metrangolo and G. Resnati, 19F Magnetic Resonance Imaging (MRI): From Design of Materials to Clinical Applications, Chem. Rev., 2015, 115, 1106–1129 CrossRef CAS PubMed.
- R. T. Kadakia, D. Xie, D. Martinez, M. Yu and E. L. Que, A dual-responsive probe for detecting cellular hypoxia using 19F magnetic resonance and fluorescence, Chem. Commun., 2019, 55, 8860–8863 RSC.
- R. T. Kadakia, D. Xie, H. Guo, B. Bouley, M. Yu and E. L. Que, Responsive fluorinated nanoemulsions for 19F magnetic resonance detection of cellular hypoxia, Dalton Trans., 2020, 49, 16419–16424 RSC.
- D. Xie, T. L. King, A. Banerjee, V. Kohli and E. L. Que, Exploiting Copper Redox for 19F Magnetic Resonance-Based Detection of Cellular Hypoxia, J. Am. Chem. Soc., 2016, 138, 2937–2940 CrossRef CAS PubMed.
- D. Xie, S. Kim, V. Kohli, A. Banerjee, M. Yu, J. S. Enriquez, J. J. Luci and E. L. Que, Hypoxia-Responsive 19F MRI Probes with Improved Redox Properties and Biocompatibility, Inorg. Chem., 2017, 56, 6429–6437 CrossRef CAS PubMed.
- J. S. Enriquez, M. Yu, B. S. Bouley, D. Xie and E. L. Que, Copper(II) complexes for cysteine detection using 19F magnetic resonance, Dalton Trans., 2018, 47, 15024–15030 RSC.
- K. E. Prosser, D. Xie, A. Chu, G. A. MacNeil, B. R. Varju, R. T. Kadakia, E. L. Que and C. J. Walsby, Copper(II) Pyridyl Aminophenolates: Hypoxia-Selective, Nucleus-Targeting Cytotoxins, and Magnetic Resonance Probes, Chem.–Eur. J., 2021, 27, 9839–9849 CrossRef CAS PubMed.
- N. Kitamura, T. Hiraoka, K. Tanaka and Y. Chujo, Reduced glutathione-resisting 19F NMR sensors for detecting HNO, Bioorg. Med. Chem., 2012, 20, 4668–4674 CrossRef CAS PubMed.
- M. Yu, D. Xie, R. T. Kadakia, W. Wang and E. L. Que, Harnessing chemical exchange: 19F magnetic resonance OFF/ON zinc sensing with a Tm(III) complex, Chem. Commun., 2020, 56, 6257–6260 RSC.
- M. Yu, D. Xie, K. P. Phan, J. S. Enriquez, J. J. Luci and E. L. Que, A CoII complex for 19F MRI-based detection of reactive oxygen species, Chem. Commun., 2016, 52, 13885–13888 RSC.
- P. B. Tsitovich, J. A. Spernyak and J. R. Morrow, A Redox-Activated MRI Contrast Agent that Switches Between Paramagnetic and Diamagnetic States, Angew. Chem., Int. Ed., 2013, 52, 13997–14000 CrossRef CAS PubMed.
- D. Xie, M. Yu, Z. L. Xie, R. T. Kadakia, C. Chung, L. E. Ohman, K. Javanmardi and E. L. Que, Versatile Nickel(II) Scaffolds as Coordination-Induced Spin-State Switches for 19F Magnetic Resonance-Based Detection, Angew. Chem., Int. Ed., 2020, 59, 22523–22530 CrossRef CAS PubMed.
- T. Nakamura, H. Matsushita, F. Sugihara, Y. Yoshioka, S. Mizukami and K. Kikuchi, Activatable 19F MRI Nanoparticle Probes for the Detection of Reducing Environments, Angew. Chem., Int. Ed., 2015, 54, 1007–1010 CrossRef CAS PubMed.
- L. A. Basal, M. D. Bailey, J. Romero, M. M. Ali, L. Kurenbekova, J. Yustein, R. G. Pautler and M. J. Allen, Fluorinated EuII-based multimodal contrast agent for temperature- and redox-responsive magnetic resonance imaging, Chem. Sci., 2017, 8, 8345–8350 RSC.
- L. A. Ekanger, L. A. Polin, Y. Shen, E. M. Haacke, P. D. Martin and M. J. Allen, A Eu(II)-Containing Cryptate as a Redox Sensor in Magnetic Resonance Imaging of Living Tissue, Angew. Chem., Int. Ed. Engl., 2015, 54, 14398–14401 CrossRef CAS PubMed.
- E. M. Gale, C. M. Jones, I. Ramsay, C. T. Farrar and P. Caravan, A Janus Chelator Enables Biochemically Responsive MRI Contrast with Exceptional Dynamic Range, J. Am. Chem. Soc., 2016, 138, 15861–15864 CrossRef CAS PubMed.
- E. M. Gale, S. Mukherjee, C. Liu, G. S. Loving and P. Caravan, Structure-redox-relaxivity relationships for redox responsive manganese-based magnetic resonance imaging probes, Inorg. Chem., 2014, 53, 10748–10761 CrossRef CAS PubMed.
- G. S. Loving, S. Mukherjee and P. Caravan, Redox-Activated Manganese-Based MR Contrast Agent, J. Am. Chem. Soc., 2013, 135, 4620–4623 CrossRef CAS PubMed.
- H. Chen, X. Tang, X. Gong, D. Chen, A. Li, C. Sun, H. Lin and J. Gao, Reversible redox-responsive 1H/19F MRI molecular probes, Chem. Commun., 2020, 56, 4106–4109 RSC.
- H. Wang, A. Wong, L. C. Lewis, G. R. Nemeth, V. C. Jordan, J. W. Bacon, P. Caravan, H. S. Shafaat and E. M. Gale, Rational Ligand Design Enables pH Control over Aqueous Iron Magnetostructural Dynamics and Relaxometric Properties, Inorg. Chem., 2020, 59, 17712–17721 CrossRef CAS PubMed.
- H. Wang, V. C. Jordan, I. A. Ramsay, M. Sojoodi, B. C. Fuchs, K. K. Tanabe, P. Caravan and E. M. Gale, Molecular Magnetic Resonance Imaging Using a Redox-Active Iron Complex, J. Am. Chem. Soc., 2019, 141, 5916–5925 CrossRef CAS PubMed.
- E. M. Snyder, D. Asik, S. M. Abozeid, A. Burgio, G. Bateman, S. G. Turowski, J. A. Spernyak and J. R. Morrow, A Class of FeIII Macrocyclic Complexes with Alcohol Donor Groups as Effective T1 MRI Contrast Agents, Angew. Chem., Int. Ed., 2020, 59, 2414–2419 CrossRef CAS PubMed.
- D. Asik, R. Smolinski, S. M. Abozeid, T. B. Mitchell, S. G. Turowski, J. A. Spernyak and J. R. Morrow, Modulating the Properties of Fe(III) Macrocyclic MRI Contrast Agents by Appending Sulfonate or Hydroxyl Groups, Molecules, 2020, 25, 2291 CrossRef CAS PubMed.
- K. Tanaka, N. Kitamura, Y. Takahashi and Y. Chujo, Reversible signal regulation system of 19F NMR by redox reactions using a metal complex as a switching module, Bioorg. Med. Chem., 2009, 17, 3818–3823 CrossRef CAS PubMed.
- S. Karbalaei, A. Franke, A. Jordan, C. Rose, P. R. Pokkuluri, R. J. Beyers, A. Zahl, I. Ivanović-Burmazović and C. R. Goldsmith, A Highly Water- and Air-Stable Iron-Containing MRI Contrast Agent Sensor for H, Chem.–Eur. J., 2022, e202201179, DOI:10.1002/chem.202201179.
- P. B. Tsitovich, P. J. Burns, A. M. McKay and J. R. Morrow, Redox-activated MRI contrast agents based on lanthanide and transition metal ions, J. Inorg. Biochem., 2014, 133, 143–154 CrossRef CAS PubMed.
- J. R. Morrow, J. J. Raymond, M. S. I. Chowdhury and P. R. Sahoo, Redox-Responsive MRI Probes Based on First-Row Transition-Metal Complexes, Inorg. Chem., 2022, 61, 14487–14499 CrossRef CAS PubMed.
- K. Srivastava, E. A. Weitz, K. L. Peterson, M. Marjańska and V. C. Pierre, Fe- and Ln-DOTAm-F12 Are Effective Paramagnetic Fluorine Contrast Agents for MRI in Water and Blood, Inorg. Chem., 2017, 56, 1546–1557 CrossRef CAS PubMed.
- A. I. Gaudette, A. E. Thorarinsdottir and T. D. Harris, pH-Dependent spin state population and 19F NMR chemical shift via remote ligand protonation in an iron(II) complex, Chem. Commun., 2017, 53, 12962–12965 RSC.
- A. E. Thorarinsdottir, A. I. Gaudette and T. D. Harris, Spin-crossover and high-spin iron(II) complexes as chemical shift 19F magnetic resonance thermometers, Chem. Sci., 2017, 8, 2448–2456 RSC.
- A. A. Kislukhin, H. Xu, S. R. Adams, K. H. Narsinh, R. Y. Tsien and E. T. Ahrens, Paramagnetic fluorinated nanoemulsions for sensitive cellular fluorine-19 magnetic resonance imaging, Nat. Mater., 2016, 15, 662 CrossRef CAS PubMed.
- A. H. Jahromi, C. Wang, S. R. Adams, W. Zhu, K. Narsinh, H. Xu, D. L. Gray, R. Y. Tsien and E. T. Ahrens, Fluorous-Soluble Metal Chelate for Sensitive Fluorine-19 Magnetic Resonance Imaging Nanoemulsion Probes, ACS Nano, 2019, 13, 143–151 CrossRef CAS PubMed.
- J. Rho, E. Stares, S. R. Adams, D. Lister, B. Leach and E. T. Ahrens, Paramagnetic Fluorinated Nanoemulsions for in vivo F-19 MRI, Mol. Imaging Biol., 2020, 22, 665–674 CrossRef CAS PubMed.
- C. Wang, S. R. Adams, H. Xu, W. Zhu and E. T. Ahrens, β-Diketonate-Iron(III) Complex: A Versatile Fluorine-19 MRI Signal Enhancement Agent, ACS. Appl. Bio. Mater., 2019, 2, 3836–3842 CrossRef CAS PubMed.
- R. T. Ryan, K. M. Scott and E. L. Que, Design Strategies for Responsive Fluorine-19 Magnetic Resonance Probes Using Paramagnetic Metal Complexes, Analysis Sensing, 2023, 3, e202200041 CAS.
- M. V. Westphal, B. T. Wolfstädter, J. M. Plancher, J. Gatfield and E. M. Carreira, Evaluation of tert-butyl isosteres: case studies of physicochemical and pharmacokinetic properties, efficacies, and activities, ChemMedChem, 2015, 10, 461–469 CrossRef CAS PubMed.
- C. Prinz, L. Starke, T. F. Ramspoth, J. Kerkering, V. Martos Riaño, J. Paul, M. Neuenschwander, A. Oder, S. Radetzki, S. Adelhoefer, P. Ramos Delgado, M. Aravina, J. M. Millward, A. Fillmer, F. Paul, V. Siffrin, J. P. von Kries, T. Niendorf, M. Nazaré and S. Waiczies, Pentafluorosulfanyl (SF 5) as a Superior 19 F Magnetic Resonance Reporter Group: Signal Detection and Biological Activity of Teriflunomide Derivatives, ACS Sens., 2021, 6, 3948–3956 CrossRef CAS PubMed.
- C. A. Chang, L. C. Francesconi, M. F. Malley, K. Kumar, J. Z. Gougoutas, M. F. Tweedle, D. W. Lee and L. J. Wilson, Synthesis, characterization, and crystal structures of M(DO3A) (M = iron, gadolinium) and Na[M(DOTA)] (M = Fe, yttrium, Gd), Inorg. Chem., 1993, 32, 3501–3508 CrossRef CAS.
- A. O. Olatunde, C. J. Bond, S. J. Dorazio, J. M. Cox, J. B. Benedict, M. D. Daddario, J. A. Spernyak and J. R. Morrow, Six, Seven or Eight Coordinate Fe(II) , Co(II) or Ni(II) Complexes of Amide-Appended Tetraazamacrocycles for ParaCEST Thermometry, Chem.–Eur. J., 2015, 21, 18290–18300 CrossRef CAS PubMed.
- K. Srivastava, G. Ferrauto, V. G. Young Jr, S. Aime and V. C. Pierre, Eight-Coordinate, Stable Fe(II) Complex as a Dual (19)F and CEST Contrast Agent for Ratiometric pH Imaging, Inorg. Chem., 2017, 56, 12206–12213 CrossRef CAS PubMed.
- X.-H. Bu, W. Chen, Z.-H. Zhang, R.-H. Zhang, S.-M. Kuang and T. Clifford, The first seven-coordinated iron(II) complex with a nitrogen donor set hepta-dentate macrocyclic polyamine ligand and different coordination modes with its cobalt(II) analogue, Inorg. Chim. Acta, 2000, 310, 110–114 CrossRef CAS.
- J. C. Joyner and J. A. Cowan, Targeted Cleavage of HIV RRE RNA by Rev-Coupled Transition Metal Chelates, J. Am. Chem. Soc., 2011, 133, 9912–9922 CrossRef CAS PubMed.
- P. M. Wood, The potential diagram for oxygen at pH 7, Biochem. J., 1988, 253, 287–289 CrossRef CAS PubMed.
- S. M. Pinto, V. Tomé, M. J. F. Calvete, M. M. C. A. Castro, É. Tóth and C. F. G. C. Geraldes, Metal-based redox-responsive MRI contrast agents, Coord. Chem. Rev., 2019, 390, 1–31 CrossRef CAS.
- D. F. Evans, The determination of the paramagnetic susceptibility of substances in solution by nuclear magnetic resonance, J. Chem. Soc., 1959, 2003–2005 RSC.
- P. B. Tsitovich, J. M. Cox, J. B. Benedict and J. R. Morrow, Six-coordinate Iron(II) and Cobalt(II) paraSHIFT Agents for Measuring Temperature by Magnetic Resonance Spectroscopy, Inorg. Chem., 2016, 55, 700–716 CrossRef CAS PubMed.
- C. J. Bond, G. E. Sokolow, M. R. Crawley, P. J. Burns, J. M. Cox, R. Mayilmurugan and J. R. Morrow, Exploring Inner-Sphere Water Interactions of Fe(II) and Co(II) Complexes of 12-Membered Macrocycles To Develop CEST MRI Probes, Inorg. Chem., 2019, 58, 8710–8719 CrossRef CAS PubMed.
- E. A. Kras, E. M. Snyder, G. E. Sokolow and J. R. Morrow, Distinct Coordination Chemistry of Fe(III)-Based MRI Probes, Acc. Chem. Res., 2022, 55, 1435–1444 CrossRef CAS PubMed.
- R. B. Lauffer, Paramagnetic metal complexes as water proton relaxation agents for NMR imaging: theory and design, Chem. Rev., 1987, 87, 901–927 CrossRef CAS.
Footnotes |
† Electronic supplementary information (ESI) available: Experimental details and supplementary figures. See DOI: https://doi.org/10.1039/d2sc05222a |
‡ These authors contributed equally to this work. |
|
This journal is © The Royal Society of Chemistry 2023 |
Click here to see how this site uses Cookies. View our privacy policy here.