Dehydrogenation of the liquid organic hydrogen carrier perhydrodibenzyltoluene – reaction pathway over Pt/Al2O3†
Received
24th August 2022
, Accepted 28th September 2022
First published on 30th September 2022
Abstract
The catalytic dehydrogenation of perhydro-dibenzyltoluene (H18-DBT) to dibenzyltoluene (DBT) on a Pt/Al2O3 catalyst was investigated using 1H NMR spectroscopy and GC-MS. It has been found that the dehydrogenation of a cyclohexyl ring of H18-DBT is very rapid and that Hx-DBT species with partially dehydrogenated rings can be neglected. Four different dehydrogenation pathways of H18-DBT to DBT, based on the ring dehydrogenation sequence were considered, including side–middle–side order, middle–side–side order, side–side–middle order, and statistical dehydrogenation with no ring preference. The H18-DBT dehydrogenation proceeds with a high preference for the side–middle–side (SMS) sequence of ring dehydrogenation. Steric hindrance leads to one of the side rings of H18-DBT dehydrogenating first. Subsequent adsorption of the H12-DBT with one side ring dehydrogenated, via the phenyl ring on the Pt surface is more stable and stronger than that of the cyclohexyl ring, causing the middle ring to be more accessible to the Pt sites and to undergo dehydrogenation. Finally, the remaining side ring is dehydrogenated to complete the SMS dehydrogenation pathway.
1. Introduction
To control greenhouse gas emissions and reduce the impact of global gas and oil consumption on climate change, hydrogen produced without CO2 emissions, has attracted the attention of numerous countries around the world.1–3 However, hydrogen storage and transportation are key barriers for the large-scale use of hydrogen as an energy carrier.4 Several hydrogen storage strategies have been proposed such as compressed hydrogen, cryogenic liquid hydrogen, metal hydrides, and liquid organic hydrogen carriers (LOHCs).5–8 LOHCs have excellent performance in terms of safety and hydrogen storage capacity.9 Various LOHCs have been proposed such as toluene,10–13N-ethylcarbazole (NEC),14–18 methylindole19–22 and dibenzyltoluene (DBT).23–26 Storage of hydrogen on LOHCs is based on the hydrogenation of hydrogen-lean organic compounds and the dehydrogenation of the corresponding hydrogen-rich compounds.27,28 A key requirement for the application of LOHCs is a low energy demand for the hydrogenation and dehydrogenation processes.29–31
DBT is a promising LOHC first proposed in 2014 by Brückner et al.23 DBT has a low vapor pressure, low ignitability, good thermal stability, and a high hydrogen storage capacity of 6.2 wt%.23,25 Liquid DBT, with a low melting point (−35 °C) and high boiling point (390 °C), is easy to store and transport at ambient conditions of various environments.32,33 In addition, the conventional crude oil infrastructure can be applied to the storage and transportation of DBT without major modification. Nevertheless, several urgent problems need to be addressed before DBT can be widely applied as a LOHC. For example, the hydrogen release rate at low temperature is unacceptably low, and side reactions such as cracking and condensation lead to irreversible loss of the DBT hydrogen carrier.34 To address these issues, dehydrogenation catalysts with improved activity and selectivity are required. Furthermore, understanding the DBT dehydrogenation mechanism would be beneficial to the identification of improved catalysts.
The DBT hydrogenation pathway was investigated by Do et al.35 over a Ru/Al2O3 catalyst using 1H NMR and HPLC. Their results showed that the hydrogenation of DBT occurred by a series of hydrogenation steps in which there was a very high preference for hydrogenation of the side phenyl rings before hydrogenation of the middle phenyl ring of DBT. To our knowledge, there are no reports on the reaction mechanism of H18-DBT dehydrogenation.
In this contribution we investigate the reaction pathway of the heterogeneously catalyzed dehydrogenation of H18-DBT to DBT over Pt/Al2O3. GC-MS, 1H NMR and Raman spectroscopy are the usual analytic methods used to quantify Hx-DBT compounds.33,35 In this paper, we investigate the H18-DBT dehydrogenation sequence using GC-MS and 1H NMR spectroscopy. This combination of analytic methods allowed us to clearly identify the H18-DBT dehydrogenation sequence.
2. Experimental
2.1 Materials
DBT (>98%) was purchased from Arkema. Activated aluminum oxide powder (Al2O3, CATALOX SCCA-25/200) was provided by Sasol. Chloroplatinic acid hexahydrate (H2PtCl6·6H2O) was purchased from Sigma. Compressed hydrogen (H2, grade 5.0, >99.999%) and argon (Ar, grade 5.0, >99.999%) were purchased from Praxair.
2.2 Catalyst preparation
Al2O3 was calcined at 600 °C for 4 h in advance. The Pt/Al2O3 catalyst with a Pt loading of 1 wt% was synthesized by impregnating Al2O3 with aqueous H2PtCl6 solution. The impregnation mixture was dried in an oven at 80 °C overnight. The dried sample was reduced at 350 °C for 2 h in a flow of hydrogen and argon (1
:
1). The Pt/Mo–Al2O3 catalyst with a Pt loading of 1 wt% was prepared by using the method in the literature.36
2.3 H18-DBT dehydrogenation
The dehydrogenation reaction was carried out in a 200 ml stainless-steel batch autoclave (Autoclave Engineers Limited) with a mechanical stirrer (n = 700 rpm). 45 g of DBT and 1 g of Pt/Al2O3 were loaded into the vessel. After purging the vessel with Ar, the autoclave was heated to 160 °C. Then the pressure was adjusted and held at 1.38 MPa with a constant hydrogen flow until DBT fully hydrogenated to H18-DBT. Then the dehydrogenation of H18-DBT was carried out at 270 °C and atmospheric pressure for 9 hours. After that the dehydrogenation temperature was raised to 290 °C for another 7 hours. The dehydrogenation degree is defined as the ratio of released hydrogen divided by the maximum hydrogen uptake capacity of DBT under consideration. The dehydrogenation degree versus dehydrogenation time at different reaction temperatures is plotted in Fig. S1.† It can be seen that the dehydrogenation degree at 290 °C after 14 h was 91.0%, which is satisfactory for a study of the dehydrogenation sequence of the 3 rings. As shown in Fig. S2,† there are light cracking byproducts such as toluene and benzyltoluene and heavy condensed byproducts were detected in the product mixture. The light and heavy byproducts in the product mixture of 300 °C are much more than that of 270 °C, so that we use the lower temperature 270–290 °C to study the dehydrogenation pathway. Liquid samples were also obtained during the dehydrogenation experiment at various time intervals.
2.4 GC-MS analysis
Gas chromatography-mass spectrometry was applied to quantify the components in the liquid samples from the dehydrogenation reaction. A QP-2010S instrument from Shimadzu with Restek RTX5 chromatographic column was applied. 0.04 mL of liquid sample was diluted in 1 mL of decalin for sample preparation. The GC injector temperature was set at 200 °C, the initial column temperature was 40 °C held for 5 min, and the increased at a ramp rate of 5 °C min−1, to the final temperature of 200 °C that was held for 15 min.
2.5 NMR analysis
Nuclear magnetic resonance (NMR) with an Avance 400 from Bruker was applied in the analysis of the liquid samples during the dehydrogenation experiment. 0.04 mL of liquid sample was diluted in 0.8 mL of dichloromethane-d2 for sample preparation.
3. Results and discussion
The GC-MS results of different liquid samples collected at different times during dehydrogenation are shown in Fig. 1. The many peaks of H18-DBT and DBT indicate that both contained many isomers. Only H18-DBT, H12-DBT, H6-DBT and DBT were detected in the liquid samples collected during dehydrogenation, which indicates that other species only exist in small amounts in the product mixture and are below the detection limit. Therefore, species with partially dehydrogenated rings could be neglected. The mass percentage of all Hx-DBT species plotted versus reaction time is shown in Fig. S3.† After 2 hours of dehydrogenation, the peaks of H12-DBT were detected indicating that one of the cyclohexyl rings of the H18-DBT molecule transformed into a phenyl ring. The peaks of H6-DBT appeared at 6 h of dehydrogenation which indicated that a second cyclohexyl ring had dehydrogenated. As a consequence, we conclude that the H18-DBT dehydrogenation process occurs ring-by-ring rather than by the simultaneous dehydrogenation of the 3 phenyl rings. However, the dehydrogenation sequence of each of the three rings of H18-DBT cannot be determined by GC-MS analysis. Neglecting partially dehydrogenated rings, six Hx-DBT species may occur during the dehydrogenation process from H18-DBT to DBT. Based on the above GC-MS analysis, the four possible dehydrogenation reaction pathways are shown in Fig. 2(a)–(d). For clarity only one regioisomer of every species is shown in Fig. 2. The four dehydrogenation pathways can be classified according to the sequence of phenyl ring dehydrogenation as: side–middle–side order (SMS), middle–side–side order (MSS), side–side–middle order (SSM), and statistical (or random) dehydrogenation with no ring preference (statistical).
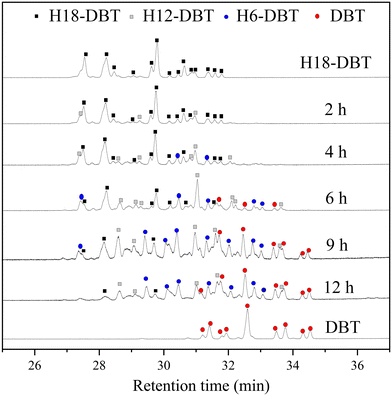 |
| Fig. 1 GC-MS results of H18-DBT, DBT and the liquid samples collected at different time during dehydrogenation. | |
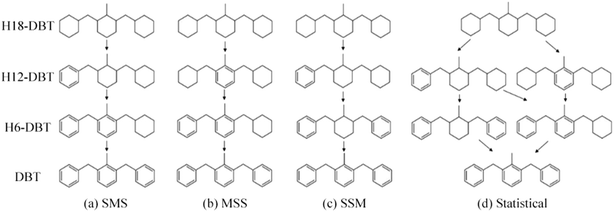 |
| Fig. 2 Different H18-DBT dehydrogenation pathways: (a) SMS: side–middle–side order; (b) MSS: middle–side–side order; (c) SSM: side–side–middle order; (d) statistical: no ring preference. | |
Fig. 3 reports the 1H NMR spectra of different dehydrogenation liquid product samples collected as a function of reaction time. Four different types of hydrogen atoms were detected according to the chemical shifts located at 0.8–1.8 ppm, 2–2.5 ppm, 3.8–4.2 ppm, and 6.8–7.4 ppm respectively, corresponding to the hydrogen atoms of the intermediates of the DBT hydrogenation process.35 The 4 chemical shifts can be assigned to hydrogen atoms in different chemical environments as listed in Table 1.35 As shown in Fig. 3(a), only the peaks at 0.8–1.8 ppm were detected for the H18-DBT feed, indicating that the dehydrogenation reactant was fully saturated. Fig. 3(b–m) also shows that the peaks at 2–2.5 ppm, 3.8–4.2 ppm, and 6.8–7.4 ppm appear after 20 min reaction time and increase gradually with reaction time, indicating that the phenyl ring appears in the product and increases gradually during the dehydrogenation process. The peaks at 0.8–1.8 ppm, corresponding to H atoms bound to cyclohexyl rings decrease gradually indicating progress in dehydrogenation of H18-DBT as the quantity of cyclohexyl rings decreased.
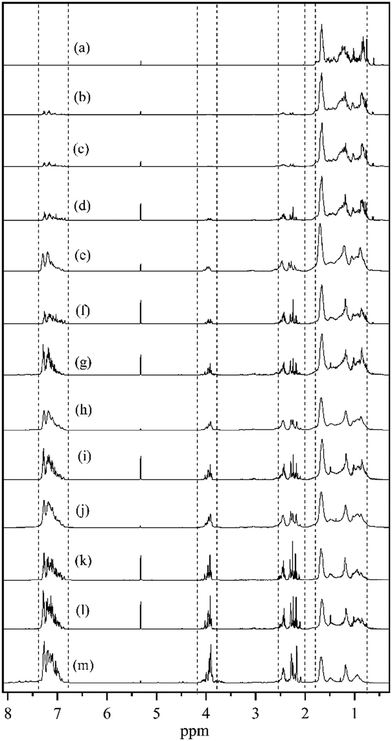 |
| Fig. 3
1H NMR spectra of (a) H18-DBT; dehydrogenation product of Pt/Al2O3 at (b) 20 min; (c) 30 min; (d) 1.5 h; (e) 2 h; (f) 2.5 h; (g) 3 h; (h) 4 h; (i) 4.5 h; (j) 6 h; (k) 8 h; (l) 9 h; (m) 12 h. | |
Table 1 The hydrogen atoms in different chemical structure environment and the corresponding chemical shifts in 1H NMR spectra
Structural environment |
Chemical shift |
|
6.8–7.4 ppm (CS[7.0]) |
|
3.8–4.2 ppm (CS[3.8–4.2]) |
|
2–2.5 ppm (CS[2.0–2.5]) |
|
0.8–1.8 ppm (CS[0.8–1.8]) |
The fractions of the different types of hydrogen atoms in the 1H NMR sample were calculated by the integration of the 1H NMR peaks corresponding to each of the four chemical shifts as shown in eqn (1)–(4). The fractions (f) of CS[0.8–1.8], CS[2–2.5], and CS[3.8–4.2] hydrogen atoms for different Hx-DBT species have been calculated based on the 1H NMR spectra (Fig. 3) and the results are shown in Table 2.
| 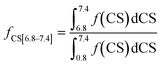 | (1) |
| 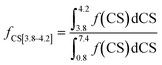 | (2) |
| 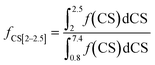 | (3) |
| 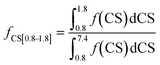 | (4) |
Table 2 The fraction distribution of the different types of hydrogen atoms in the dehydrogenated liquid products
Molecular structure |
Degree of dehydrogenation |
f
CS[0.8–1.8]
|
f
CS[2–2.5]
|
f
CS[3.8–4.2]
|
f
CS[6.8–7.4]
|
H18-DBT  |
0% |
1.00 |
0.00 |
0.00 |
0.00 |
H12-DBT  |
33.3% |
0.69 |
0.22 |
0.00 |
0.09 |
H12-DBT  |
33.3% |
0.78 |
0.06 |
0.00 |
0.16 |
H6-DBT  |
66.7% |
0.46 |
0.16 |
0.00 |
0.38 |
H6-DBT  |
66.7% |
0.42 |
0.19 |
0.08 |
0.31 |
H0-DBT  |
100% |
0.00 |
0.15 |
0.20 |
0.65 |
We analyse the dehydrogenation pathway by plotting two of fCS[0.8–1.8], fCS[2–2.5], fCS[3.8–4.2] and fCS[6.8–7.4] as horizontal axis and vertical axis respectively. Then, by plotting the points of different Hx-DBT species according to the characteristic fractions of different hydrogens from Table 2, the data points are connected as straight lines according to the dehydrogenation pathways (SMS, MSS, SSM) from Fig. 2. We take the statistical pathway as the simultaneous occurrence of the three SMS, MSS, SSM pathways, so that an equal mixture of intermediates from each step of the three pathways are regarded as the two intermediate points of the Statistical pathway. These plots are shown in Fig. 4 and the fractions of chemical shift integrals of the experimental samples from Fig. 3(b)–(m) are plotted as five-pointed stars in Fig. 4.
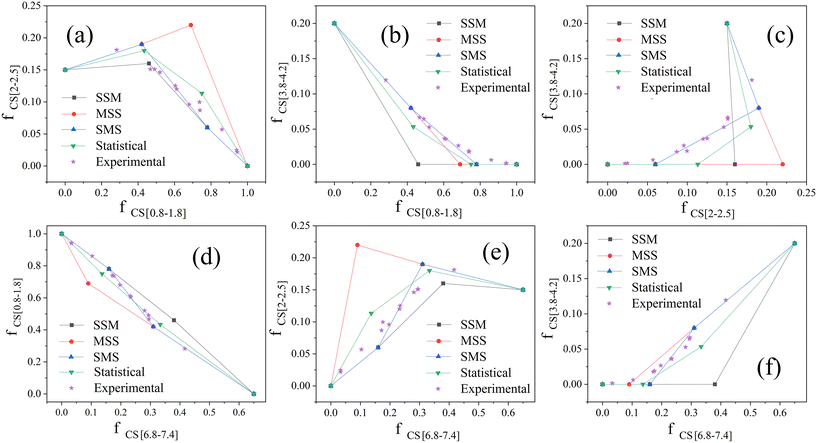 |
| Fig. 4 Calculated fractions of chemical shift integrals of (a) CS[2–2.5] to CS[0.8–1.8]; (b) CS[3.8–4.2] to CS[0.8–1.8]; (c) CS[3.8–4.2] to CS[2–2.5]; (d) CS[0.8–1.8] to CS[6.8–7.4]; (e) CS[2–2.5] to CS[6.8–7.4]; (f) CS[3.8–4.2]–CS[6.8–7.4] and the 1H NMR results of the dehydrogenation products of Pt/Al2O3. | |
As shown in Fig. 4(a) and (c)–(e), the experimental values are significantly displaced from the MSS pathway, so that the MSS pathway is an unlikely candidate for the actual dehydrogenation pathway. Based on the result in Fig. 4(b) and (f), the SSM pathway can also be excluded. Taking Fig. 4(c) into account, the experimental data match the SMS pathway very well. The average residual (AR) based on the absolute distances (AD) between each experimental point and each dehydrogenation pathway was calculated by the eqn (5), and hence, the average residual was listed in Table 3.
|  | (5) |
Table 3 The average residual between each experimental point and each dehydrogenation pathway
Plots |
Average residual |
SSM |
MSS |
SMS |
Statistical |
f
CS[0.8–1.8]–fCS[2–2.5] |
0.046 |
0.336 |
0.042 |
0.064 |
f
CS[0.8–1.8]–fCS[3.8–4.2] |
0.142 |
0.039 |
0.016 |
0.047 |
f
CS[2–2.5]–fCS[3.8–4.2] |
0.069 |
0.101 |
0.016 |
0.067 |
f
CS[6.8–7.4]–fCS[0.8–1.8] |
0.129 |
0.196 |
0.022 |
0.053 |
f
CS[6.8–7.4]–fCS[2–2.5] |
0.079 |
0.277 |
0.045 |
0.078 |
f
CS[6.8–7.4]–fCS[3.8–4.2] |
0.145 |
0.035 |
0.021 |
0.047 |
Clearly the experimental points and the SMS pathway, with average residual of 0.042, 0.016, 0.016, 0.022, 0.045, and 0.021 for the six plots, are the lowest among all the pathways. As a consequence, the SMS pathway is identified as the most likely pathway for the dehydrogenation of H18-DBT on the surface of Pt/Al2O3. The same analysis was applied to another Pt-based heterogeneous catalyst Pt/Mo–Al2O3. As shown in Fig. S4 and Table S1,† the experimental points also follow the SMS pathway with average residual of 0.021, 0.010, 0.014, 0.012, 0.014, and 0.009 for the six plots. Therefore, the competitive adsorption of phenyl ring and cyclohexyl ring on Pt surface is the crucial factor in determining the dehydrogenation pathway.
The SMS dehydrogenation pathway is a consequence of steric hindrance effects and competitive adsorption of phenyl ring and cyclohexyl ring on the surface of catalysts. Steric hindrance rather that competitive adsorption leads to the first side ring being dehydrogenated because H18-DBT contains 3 cyclohexyl rings without phenyl ring. Subsequently, the adsorption of the phenyl ring on the Pt surface is more stable and stronger than that of a cyclohexyl ring.37,38 Therefore, as shown in Fig. 5(A), after the dehydrogenation of the first side ring A1, the phenyl side ring A1 adsorbed on the Pt surface will be preferred over the cyclohexyl side ring of H12-DBT. The middle ring A2 will also be more accessible to the Pt surface than the side ring, so the middle cyclohexyl dehydrogenates next. Finally, the other side ring A3 is dehydrogenated as the last dehydrogenation ring. This explanation is also valid for the SSM pathway of hydrogenation of DBT reported in the literature.35 As shown in Fig. 5(B), the first side ring B1 should be hydrogenated due to the steric hindrance. The phenyl side rings B2 or B3 adsorb more easily on the catalyst surface than the cyclohexyl ring B1, so that the side phenyl ring B2 should be hydrogenated second rather than middle phenyl ring B3 due to the steric hindrance. This hydrogenation pathway is in accordance with the results described in the literature.35 This analysis indicates that competitive adsorption between the phenyl ring and the cyclohexyl ring of Hx-DBT on the surface of catalysts is the crucial factor for both the DBT hydrogenation and H18-DBT dehydrogenation pathways.
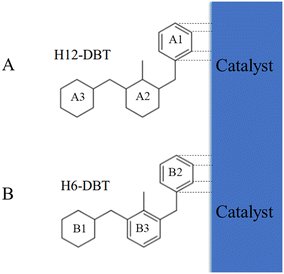 |
| Fig. 5 Hx-DBT and the catalyst surface: (A) H12-DBT after the first side ring dehydrogenation of H18-DBT; (B) H6-DBT after the first side ring hydrogenation of DBT. | |
4. Conclusion
In this contribution the dehydrogenation pathway of H18-DBT to DBT on a Pt/Al2O3 catalyst in 270–290 °C was investigated by using 1H NMR spectroscopy and GC-MS. It has been found that only phenyl and cyclohexyl rings are involved in the H18-DBT dehydrogenation reaction and the Hx-DBT species with partially dehydrogenated rings can be neglected. The competitive adsorption of the phenyl ring and the cyclohexyl ring of Hx-DBT on the surface of the catalysts is the critical factor for both the DBT hydrogenation and H18-DBT dehydrogenation pathways. The H18-DBT dehydrogenation proceeds with a high preference for the pathway of side ring–middle ring–side ring dehydrogenation order. The steric hindrance leads one of the side rings of H18-DBT to dehydrogenate first. Then the adsorption of the phenyl ring on the Pt surface is more stable and stronger than that of the cyclohexyl ring, causing the middle ring to be more accessible to the Pt active sites and undergo dehydrogenation. Finally, the other side ring is the last ring to dehydrogenate. This mechanistic insight may help to understand the effect of active metal and morphology of the catalysts on the H18-DBT dehydrogenation. Moreover, the optimization of catalyst for H18-DBT dehydrogenation may be benefit from this insight.
Author contributions
Libin Shi: conceptualization, methodology, validation, resources, formal analysis, investigation, writing – original, visualization. Suitao Qi: funding acquisition, supervision, writing – review & editing, project administration. Kevin J. Smith: funding acquisition, supervision, project administration, writing – review & editing. Majed Alamoudi: validation. Yiming Zhou: writing – review & editing.
Conflicts of interest
There are no conflicts to declare.
Acknowledgements
National Natural Science Foundation of China no. 22038011, and China Scholarships Council no. 201906280152 are acknowledged. Funding from the Natural Sciences and Engineering Research Council of Canada is also acknowledged. We also thank Dr. Maria Ezhova in the Department of Chemistry, University of British Columbia for her assistance with 1H NMR experiments.
References
- F. Liu, D. L. Mauzerall, F. Zhao and H. Hao, Deployment of fuel cell vehicles in China: Greenhouse gas emission reductions from converting the heavy-duty truck fleet from diesel and natural gas to hydrogen, Int. J. Hydrogen Energy, 2021, 46, 17982–17997, DOI:10.1016/j.ijhydene.2021.02.198.
- B. E. Lebrouhi, J. J. Djoupo, B. Lamrani, K. Benabdelaziz and T. Kousksou, Global hydrogen development - A technological and geopolitical overview, Int. J. Hydrogen Energy, 2022, 47, 7016–7048, DOI:10.1016/j.ijhydene.2021.12.076.
- C. Zhang, J. B. Greenblatt, M. Wei, J. Eichman, S. Saxena and M. Muratori,
et al., Flexible grid-based electrolysis hydrogen production for fuel cell vehicles reduces costs and greenhouse gas emissions, Appl. Energy, 2020, 278, 115651, DOI:10.1016/j.apenergy.2020.115651.
- J.-W. Grimaldo-Guerrero, J. D. L. H. Barcelo, D. Rivera-Pacheco, L. Ramos-Barrera and U. Martinez-Palacio, A Review of History, Production and Storage of Hydrogen, J. Eng. Sci. Technol. Rev., 2021, 14, 121–134, DOI:10.25103/jestr.145.14.
- Q.-L. Zhu and Q. Xu, Liquid organic and inorganic chemical hydrides for high-capacity hydrogen storage, Energy Environ. Sci., 2015, 8, 478–512, 10.1039/c4ee03690e.
- O. Faye, J. Szpunar and U. Eduok, A critical review on the current technologies for the generation, storage, and transportation of hydrogen, Int. J. Hydrogen Energy, 2022, 47(29), 13771–13802, DOI:10.1016/j.ijhydene.2022.02.112.
- S. P. Patil, J. V. Pande and R. B. Biniwale, Non-noble Ni–Cu/ACC bimetallic catalyst for dehydrogenation of liquid organic hydrides for hydrogen storage, Int. J. Hydrogen Energy, 2013, 38, 15233–15241 CrossRef CAS.
- A. Bourane, M. Elanany, T. V. Pham and S. P. Katikaneni, An overview of organic liquid phase hydrogen carriers, Int. J. Hydrogen Energy, 2016, 41, 23075–23091, DOI:10.1016/j.ijhydene.2016.07.167.
- D. Teichmann, W. Arlt, P. Wasserscheid and R. Freymann, A future energy supply based on Liquid Organic Hydrogen Carriers (LOHC), Energy Environ. Sci., 2011, 4, 2767–2773, 10.1039/c1ee01454d.
- M. S. Akram, R. Aslam, F. S. Alhumaidan and M. R. Usman, An exclusive kinetic model for the methylcyclohexane dehydrogenation over alumina-supported Pt catalysts, Int. J. Chem. Kinet., 2020, 52, 415–449, DOI:10.1002/kin.21360.
- J. Meng, F. Zhou, H. Ma, X. Yuan, Y. Wang and J. Zhang, A Review of Catalysts for Methylcyclohexane Dehydrogenation, Top. Catal., 2021, 64, 509–520, DOI:10.1007/s11244-021-01465-6.
- W. Wang, L. Miao, K. Wu, G. Chen, Y. Huang and Y. Yang, Hydrogen evolution in the dehydrogenation of methylcyclohexane over Pt/Ce Mg Al O catalysts derived from their layered double hydroxides, Int. J. Hydrogen Energy, 2019, 44, 2918–2925, DOI:10.1016/j.ijhydene.2018.12.072.
- A. A. Shukla, P. V. Gosavi, J. V. Pande, V. P. Kumar, K. V. R. Chary and R. B. Biniwale, Efficient hydrogen supply through catalytic dehydrogenation of methylcyclohexane over Pt/metal oxide catalysts, Int. J. Hydrogen Energy, 2010, 35, 4020–4026, DOI:10.1016/j.ijhydene.2010.02.014.
- X. Gong, Z. Jiang and T. Fang, Enhancing selectivity and reducing cost for dehydrogenation of dodecahydro-N-ethylcarbazole by supporting platinum on titanium dioxide, Int. J. Hydrogen Energy, 2020, 45, 6838–6847, DOI:10.1016/j.ijhydene.2019.12.203.
- Z. Feng, X. Chen and X. Bai, Hydrogen production from the catalytic dehydrogenation of dodecahydro-N-ethylcarbazole: effect of Pd precursor on the catalytic performance of Pd/C catalysts, Environ. Sci. Pollut. Res., 2021, 28, 61623–61635, DOI:10.1007/s11356-021-15108-6.
- B. Wang, T.-y. Chang, Z. Jiang, J.-j. Wei, Y.-h. Zhang and S. Yang,
et al., Catalytic dehydrogenation study of dodecahydro-N-ethylcarbazole by noble metal supported on reduced graphene oxide, Int. J. Hydrogen Energy, 2018, 43, 7317–7325, DOI:10.1016/j.ijhydene.2018.02.156.
- F. Sotoodeh and K. J. Smith, Structure sensitivity of dodecahydro-N-ethylcarbazole dehydrogenation over Pd catalysts, J. Catal., 2011, 279, 36–47, DOI:10.1016/j.jcat.2010.12.022.
- Z. Jiang, X. Gong, S. Guo, Y. Bai and T. Fang, Engineering PdCu and PdNi bimetallic catalysts with adjustable alloying degree for the dehydrogenation reaction of dodecahydro-N-ethylcarbazole, Int. J. Hydrogen Energy, 2021, 46, 2376–2389, DOI:10.1016/j.ijhydene.2020.10.123.
- P. Bachmann, J. Steinhauer, F. Spath, F. Dull, U. Bauer and R. Eschenbacher,
et al., Dehydrogenation of the liquid organic hydrogen carrier system 2-methylindole/2-methylindoline/2-methyloctahydroindole on Pt(111), J. Chem. Phys., 2019, 151, 144711, DOI:10.1063/1.5112835.
- L. Li, M. Yang, Y. Dong, P. Mei and H. Cheng, Hydrogen storage and release from a new promising Liquid Organic Hydrogen Storage Carrier (LOHC): 2-methylindole, Int. J. Hydrogen Energy, 2016, 41, 16129–16134, DOI:10.1016/j.ijhydene.2016.04.240.
- M. Yang, G. Cheng, D. Xie, T. Zhu, Y. Dong and H. Ke,
et al., Study of hydrogenation and dehydrogenation of 1-methylindole for reversible onboard hydrogen storage application, Int. J. Hydrogen Energy, 2018, 43, 8868–8876, DOI:10.1016/j.ijhydene.2018.03.134.
- A. Søgaard, M. Scheuermeyer, A. Bösmann, P. Wasserscheid and A. Riisager, Homogeneously-catalysed hydrogen release/storage using the 2-methylindole/2-methylindoline LOHC system in molten salt-organic biphasic reaction systems, Chem. Commun., 2019, 55, 2046–2049, 10.1039/c8cc09883b.
- N. Brückner, K. Obesser, A. Bösmann, D. Teichmann, W. Arlt and J. Dungs,
et al., Evaluation of industrially applied heat-transfer fluids as liquid organic hydrogen carrier systems, ChemSusChem, 2014, 7, 229–235, DOI:10.1002/cssc.201300426.
- S. Lee, J. Lee, T. Kim, G. Han, J. Lee and K. Lee,
et al., Pt/CeO2 catalyst synthesized by combustion method for dehydrogenation of perhydro-dibenzyltoluene as liquid organic hydrogen carrier: Effect of pore size and metal dispersion, Int. J. Hydrogen Energy, 2021, 46, 5520–5529, DOI:10.1016/j.ijhydene.2020.11.038.
- K. Müller, R. Aslam, A. Fischer, K. Stark, P. Wasserscheid and W. Arlt, Experimental assessment of the degree of hydrogen loading for the dibenzyl toluene based LOHC system, Int. J. Hydrogen Energy, 2016, 41, 22097–22103, DOI:10.1016/j.ijhydene.2016.09.196.
- A. Ali, G. U. Kumar and H. J. Lee, Investigation of hydrogenation of Dibenzyltoluene as liquid organic hydrogen carrier, Mater. Today: Proc., 2021, 45, 1123–1127, DOI:10.1016/j.matpr.2020.03.232.
- T. He, Q. Pei and P. Chen, Liquid organic hydrogen carriers, J. Energy Chem., 2015, 24, 587–594, DOI:10.1016/j.jechem.2015.08.007.
- P. C. Rao and M. Yoon, Potential Liquid-Organic Hydrogen Carrier (LOHC) Systems: A Review on Recent Progress, Energies, 2020, 13, 6040, DOI:10.3390/en13226040.
- A. Shukla, J. V. Pande and R. B. Biniwale, Dehydrogenation of methylcyclohexane over Pt/V2O5 and Pt/Y2O3 for hydrogen delivery applications, Int. J. Hydrogen Energy, 2012, 37(4), 3350–3357, DOI:10.1016/j.ijhydene.2011.11.078.
- A. N. Kalenchuk, V. I. Bogdan, S. F. Dunaev and L. M. Kustov, Dehydrogenation of polycyclic naphthenes on a Pt/C catalyst for hydrogen storage in liquid organic hydrogen carriers, Fuel Process. Technol., 2018, 169, 94–100, DOI:10.1016/j.fuproc.2017.09.023.
- A. Wunsch, T. Berg and P. Pfeifer, Hydrogen Production from the LOHC Perhydro-Dibenzyl-Toluene and Purification Using a 5 microm PdAg-Membrane in a Coupled Microstructured System, Materials, 2020, 13(2), 277, DOI:10.3390/ma13020277.
- K. Müller, K. Stark, V. N. Emel'yanenko, M. A. Varfolomeev, D. H. Zaitsau and E. Shoifet,
et al., Liquid Organic Hydrogen Carriers: thermophysical and thermochemical studies of benzyl- and dibenzyl-toluene derivatives, Ind. Eng. Chem. Res., 2015, 54, 7967–7976, DOI:10.1021/acs.iecr.5b01840.
- P. Inhetveen, N. S. A. Alt and E. Schluecker, Measurement of the hydrogenation level of dibenzyltoluene in an innovative energy storage system, Vib. Spectrosc., 2016, 83, 85–93, DOI:10.1016/j.vibspec.2016.01.008.
- L. Shi, Y. Zhou, S. Qi, K. J. Smith, X. Tan and J. Yan,
et al., Pt Catalysts Supported on H2 and O2 Plasma-Treated Al2O3 for Hydrogenation and Dehydrogenation of the Liquid Organic Hydrogen Carrier Pair Dibenzyltoluene and Perhydrodibenzyltoluene, ACS Catal., 2020, 10, 10661–10671, DOI:10.1021/acscatal.0c03091.
- G. Do, P. Preuster, R. Aslam, A. Bösmann, K. Müller and W. Arlt,
et al., Hydrogenation of the liquid organic hydrogen carrier compound dibenzyltoluene – reaction pathway determination by 1H NMR spectroscopy, React. Chem. Eng., 2016, 1, 313–320, 10.1039/c5re00080g.
- L. Shi, Y. Zhou, X. Tan, S. Qi, K. J. Smith and C. Yi,
et al., The effects of alumina morphology and Pt electron property on reversible hydrogenation and dehydrogenation of dibenzyltoluene as a liquid organic hydrogen carrier, Int. J. Hydrogen Energy, 2022, 47, 4704–4715, DOI:10.1016/j.ijhydene.2021.11.076.
- F. Chen, Y. Huang, C. Mi, K. Wu, W. Wang and W. Li,
et al., Density functional theory study on catalytic dehydrogenation of methylcyclohexane on Pt(111), Int. J. Hydrogen Energy, 2020, 45, 6727–6737, DOI:10.1016/j.ijhydene.2019.12.096.
- W. Zhao, C. Chizallet, P. Sautet and P. Raybaud, Dehydrogenation mechanisms of methyl-cyclohexane on γ-Al2O3 supported Pt13: Impact of cluster ductility, J. Catal., 2019, 370, 118–129, DOI:10.1016/j.jcat.2018.12.004.
|
This journal is © The Royal Society of Chemistry 2023 |