DOI:
10.1039/D3RA06514F
(Paper)
RSC Adv., 2023,
13, 30002-30009
An investigative study into an oscillatory reaction in acoustically levitated droplets†
Received
24th September 2023
, Accepted 5th October 2023
First published on 13th October 2023
Abstract
For the first time we have studied an oscillatory chemical reaction (the well-known Belousov–Zhabotinsky (BZ) reaction) in acoustically levitated droplets. Acoustically levitated droplets allow wall-less reaction studies, reduce consumption of sample/reagents, offer high throughput measurements, and enable environmentally friendly chemistry by significantly reducing plastic waste. In this work, microdroplets of the BZ reactants were mixed at the central axis of a low-cost acoustic levitator. The chemical reaction observed in acoustically levitated droplets proceeded in the same way as that in both stirred and unstirred vials where the volume of droplets was 750-fold lower than the solutions in vials. The observed oscillation frequency in droplets was lower than that observed in vials, possibly as a result of evaporative cooling of the droplets. This work has shown that oscillatory reactions can be successfully carried out in acoustically levitated droplets, which allows the application of this technique to areas such as analysis, synthesis and actuation of smart materials and studies of the origins of life.
1 Introduction
Chemical reactions can be performed in droplet microfluidic devices1–3 to reap benefits such as automation and small volumes.4 However, in current microfluidic devices, (1) droplets are in contact with either solid walls5–7 or immiscible liquids,8 and (2) operations on droplets are conducted on two-dimensional surfaces. While the former can result in loss of sample/reagents and cross-contamination, the latter can limit throughput per unit footprint of devices. These issues can be addressed by performing reactions in droplets levitated in air in three-dimensional space. Furthermore, the use of levitated droplets can reduce laboratory plastic waste, which is currently estimated to be about a thousand kilograms per researcher per year.9,10
Levitation of droplets requires an upward force sufficient to counteract the downward gravitational force.11 This upward force can be generated using light,12 electric13 or magnetic14 fields, aerodynamics,12 and acoustics.12 The use of acoustics is beneficial because droplets of up to 100 μL of all types of materials can potentially be levitated stably.15 Acoustic levitators are often formed by placing two surfaces opposite to each other where one surface comprises of ultrasound emitter(s) and the other surface is either a reflector or comprises of ultrasound emitter(s).11,16 Standing pressure waves are formed between two oppositely placed surfaces with low pressure regions (i.e., nodes) separated by approximately half a wavelength of the sound waves produced by the emitters.17,18 Acoustic radiation forces are maximum at pressure nodes. Thus, objects are levitated around pressure nodes. Acoustically levitated droplets have so far successfully been applied for a range of applications including sample preparation,19 acid–base titrations,20 synthesis,15,21 crystallisation,22 enzymatic assays,23 and chemical analysis.21 However, there are no reports on the use of acoustically levitated droplets for performing oscillatory chemical reactions. Oscillatory chemical reactions have applications ranging from analysis24 to synthesis25 and actuating26 smart materials to studying origin of life.27
A well-known oscillatory reaction is the Belousov–Zhabotinsky (BZ) reaction.28–30 Overall, the BZ reaction involves oxidation of malonic acid by bromate ions, and is summarised in eqn (1).31,32
|
5CH2(COOH)2 + 3BrO3− + 3H+ → 3BrCH(COOH)2 + 2HCOOH + 4CO2 + 5H2O
| (1) |
The oscillating reaction consists of ten fundamental interacting chemical reactions,31 which are provided in eqn (2)–(11). A double arrow indicates a reversible reaction; a single arrow indicates an essentially irreversible reaction.
|
HOBr + Br− + H+ Br2 + H2O
| (2) |
|
HBrO2 + Br− + H+ → 2HOBr
| (3) |
|
BrO3− + Br− + 2H+ → HBrO2 + HOBr
| (4) |
|
2HBrO2 → BrO3− + HOBr + H+
| (5) |
|
 | (6) |
|
 | (7) |
|
 | (8) |
|
Br2 + CH2(COOH)2 → CHBr(COOH)2 + Br− + H+
| (9) |
|
6M4+ + CH2(COOH)2 + 2H2O → 6M3+ + HCOOH + 2CO2 + 6H+
| (10) |
|
4M4+ + CHBr(COOH)2 + 2H2O → 4M3+ + Br− + HCOOH + 2CO2 + 5H+
| (11) |
Where M is a suitable metal ion. The oscillating reaction consists of three main processes. Process 1 is the reduction of bromate to bromine by the three steps in
eqn (2)–(4) and the concomitant production of bromomalonic acid
via eqn (9). Process 2 is the reduction of bromate by bromous acid
via eqn (5) and
(6). Process 3 is the reduction of the metal ion catalyst
via the reactions in
eqn (8),
(10) and
(11). Process 1 decreases the concentration of bromide, and once this concentration falls below some threshold, process 2 begins to dominate and bromous acid starts to reduce bromate. As this occurs, the concentration of M
4+ increases rapidly
via eqn (6) and
(7), thus increasing the rate of process 3 which in turn increases the concentration of bromide. As the bromide concentration varies, either process 1 or 2 dominates the reaction, resulting in oscillations in the relative concentrations of M
4+ and M
3+ thus causing cyclic changes in the colour of the solution. Commonly cerium(
IV) ammonium nitrate is used as the metal catalyst, resulting in the colour of the BZ reaction solution oscillating between yellow and colourless. To make the colour oscillations more prominent, ferroin (
i.e., tris(1,10-phenanthroline) ferrous sulphate) can be added. In this case, BZ reaction solutions undergo four colour oscillations as species are oxidised and reduced.
The BZ reaction has been studied in droplets, but in all the previously reported studies the droplets were in contact with solid walls and/or with an immiscible carrier fluid. Delgado et al.33 produced uniform 1 nL droplets in a microfluidic device and reported coupling of the reactions in adjacent droplets mediated by exchange of bromine via the fluorinated carrier fluid. They also noted that the BZ reaction cannot be carried out in unsaturated carrier fluids as bromine reacts with carbon–carbon double bonds. Similarly, Szymanski et al.34 showed that signals could be propagated by diffusion of bromine between droplets of the BZ reaction mixture suspended in decane as a carrier fluid. Kitahata et al.35 showed that the BZ reaction in a droplet suspended in oleic acid could induce motion of the droplet. In this study, bromine would have been lost from the reaction as oleic acid contains a carbon–carbon double bond. Unlike the present work, in previous work the droplets were unstirred and could lose reactants, particularly bromine, into the carrier fluid.
This is an unprecedented report on the study of an oscillatory BZ chemical reaction in acoustically levitated droplets. We showed that the oscillation period of the BZ reaction in 4 μL levitated droplets is comparable to 3 mL solutions in vials. Equally, we showed that multiple acoustically levitated droplets of BZ reaction solutions can be studied with high reproducibility and repeatability. Thus, the reported approach of performing reactions in acoustically levitated droplets when used in combination with automated fluid sampling methods will be well suited for high-throughput experimentation.
2 Experimental
2.1 Chemicals and materials
Sulphuric acid (H2SO4), malonic acid (CH2(COOH)2), potassium bromate (KBrO3), potassium bromide (KBr), cerium(IV) ammonium nitrate (Ce(NH4)2(NO3)6), iron(II) sulphate heptahydrate (FeSO4·7H2O), and 1,10-phenanthroline were purchased from Sigma-Aldrich. The components of the TinyLev device were bought from eBay and assembled in-house.
2.2 Instrumentation
To study the BZ reaction in acoustically levitated droplets, the TinyLev device (see Fig. 1) reported by Marzo et al.36–40 was used. The main structure of the levitator was 3D printed with polylactic acid (PLA) filament and comprised of two curved surfaces placed ∼15 cm apart. Each curved surface had a total of 36 ultrasonic emitters (MSO-P1040H07T, Manorshi) arranged in rings of 6, 12, and 18 with hexagonal packing. Each emitter was 10 mm in diameter and produced sound waves of 40 kHz, which corresponds to a wavelength of 8.65 mm in air. Thus, the minimum separation distance between levitated objects was ∼4.32 mm. The ultrasound emitters were driven by a square wave with peak-to-peak voltage of up to 20 V, which was generated using a combination of an Arduino Nano and a L298N Dual Motor Drive board. The acoustic levitator was placed inside a black box. Images were captured using a CMOS camera (UI3580LE-C-HQ with an imaging area of 4.2 × 5.6 mm2 and pixel size of 2.2 × 2.2 μm2, iDS Imaging) and lens (MVL25M23, Thorlabs). A daylight wafer light box (model number: D/E/U/A 35040, 4 W LEDs) was used as a light source. The light source and camera were placed opposite to each other to measure absorbance of droplets.
 |
| Fig. 1 Schematic of the TinyLev integrated with absorption measurements where each ultrasound emitter is about 10 mm. | |
2.3 Procedure
Stock solutions of 0.5 M KBrO3, 1 M KBr, 1 M CH2(COOH)2 and 0.5 M Ce(NH4)2(NO3)6 were prepared in 0.9 M sulphuric acid. A stock solution of 0.5% (w/v) ferroin was made by adding 0.024 g iron(II) sulphate heptahydrate and 0.046 g 1,10-phenanthroline in 10 mL 0.9 M sulphuric acid.
To study the chemical oscillator in vials, stock solutions of KBrO3, KBr and CH2(COOH)2 were added in selected volumes in 0.9 M sulphuric acid to make 3 mL solution containing 77 mM, 20 mM, and 100 mM, respectively. The solution in one of the vials was unstirred while the other was stirred at ∼300 rpm using a magnetic bar and plate. To both unstirred and stirred vials, stock solutions of ferroin and Ce(NH4)2(NO3)6 were added to obtain final concentrations of 0.05% (w/v) and 8 mM, respectively. We call the resulting solution as 1× mixture (see Table 1). Movies of the reaction in vials were recorded using an Android phone camera at 30 frames per second.
Table 1 Composition of 1× mixture
Chemical |
H2SO4 |
KBrO3 |
KBr |
CH2(COOH)2 |
Ferroin |
Ce(NH4)2(NO3)6 |
Concentration |
0.9 M |
77 mM |
20 mM |
100 mM |
0.05% (w/v) |
8 mM |
Expanded polystyrene particles were first levitated to serve as a guide for finding the central axis of the TinyLev. Up to five 4 μL droplets of solution containing KBrO3, KBr and CH2(COOH)2 were pipetted in the central axis of the levitator and were successfully levitated. Subsequently, selected volumes of ferroin and Ce(NH4)2(NO3)6 solutions were pipetted into the levitated droplets. We studied 1× and 2× (double the concentrations of 1× mixture) mixtures in acoustically levitated droplets. A thin piece of tissue was used to cover the transducers on the bottom surface to prevent any damage by falling droplets. Movies of levitated droplets were made using uEye Cockpit software (iDS Imaging) at 4.4 frames per second.
The ImageJ open-source image processing program was used to extract information frame by frame from movies of the droplets. An ImageJ macro was written to pick the centre of either the solutions in the vials or the levitated droplets. The movies were split into a sequence of images, after which each image was separated into blue, green, and red colour channels. An average grayscale value for all three colours were determined over a rectangle of 100 pixels wide and 100 pixels high centred around the picked point. Transmission was calculated by taking the ratio of average grayscale values at a given time and those before adding reagents. Transmissions were then converted to absorbances. Absorbances versus time for the green channel showed the greatest change and hence were fast Fourier transformed (FFT) to determine the frequency of oscillations.
3 Results and discussion
3.1 Glass vials
Initially the BZ chemical oscillator was studied in glass vials before carrying out experiments in acoustically levitated droplets. Because it is known41 that acoustically levitated droplets undergo stirring arising from the levitating acoustic field, the reaction was studied under both unstirred and stirred conditions. As shown in Fig. S1 (in the ESI)† oscillations in colour were observed in both cases, but the unstirred vial shows both temporal and spatial variations in color while the stirred vial is spatially uniform. Similar studies could not be performed for 2× mixture as the absorbance was too high.
Fig. 2 shows the changes in absorbance for the camera green and blue channels as a function of time. Oscillations were clearly visible for both vials, but the amplitude of the oscillations reduced more rapidly for the unstirred vial. This may be a result of the spatial non-uniformity of the unstirred reaction. FFT of all oscillations except the first one in the green channel shown in Fig. 2 was performed, which revealed that the reaction occurred slightly faster in the unstirred vial, with an average frequency of 1.119 ± 0.008 min−1 compared to 0.875 ± 0.017 min−1 for the stirred vial. The corresponding average oscillation periods for the unstirred and stirred vials are 0.894 ± 0.006 min and 1.143 ± 0.017 min, respectively. This is in accordance with the observations of Ruoff and Schwitters.42 Since the reaction proceeded as expected in the stirred vial, it was then carried out in acoustically levitated droplets.
 |
| Fig. 2 Absorbances for the green and blue channels versus time for the BZ chemical oscillator (1× mixture) in (a) unstirred and (b) stirred vials. | |
3.2 Acoustically levitated droplets
In this work the initial droplets and subsequent reagent solutions that initiated the BZ oscillator were pipetted manually. Occasionally droplets were lost during this process and the oscillations had to be started sequentially. These limitations can be overcome by integration of acoustic levitators with 3D printed autosamplers similar to one reported previously by the authors.43
Fig. 3 shows a montage of images taken at 4.4 frames per second after the addition of the ferroin reagent to five droplets levitated simultaneously, showing the acoustically driven mixing that occurs.
 |
| Fig. 3 Montage of images of five droplets levitated simultaneously on addition of the ferroin reagent with a time resolution of 227 ms where the vertical direction is along the central axis of the levitator (images should be read from top left to right and then from top to bottom row, and the size of the box surrounding the five droplets is 3.3 mm by 12.5 mm). | |
Movie S1† showing the addition of ferroin reagent and acoustically driven mixing is included as a ESI.† In this case, full mixing took at most 6.8 s (30 frames) and showed that the centre of the droplet is only weakly stirred by the acoustic field. This implies that internal flow within acoustically levitated droplets was high around their circumference and minimal at their centre. The internal flow patterns in acoustically levitated droplets observed in this work agree with previous reports.44 Because the oscillation period is much longer than this mixing time, we can consider that the droplets were fully stirred during the BZ reaction. Significant distortions of the droplet shape lasting about 3.4 s can also be seen after withdrawal of the pipette. Acoustic radiation force on a small spherical particle is calculated from the gradient of Gor'kov potential.45–47 Changes in the Gor'kov potential are at a maximum along the central axis of the levitator, providing a stronger restoring force in that direction compared to the orthogonal directions. This means that distortions of the droplet are larger in directions orthogonal to the levitator axis, as shown in Fig. 3. This is more pronounced for the droplets furthest from the acoustic focus in the centre of the levitator where the acoustic restoring forces orthogonal to the levitator's vertical axis are weakest.
Fig. 4 is a montage of images of the middle droplet out of the five levitated droplets showing colour oscillations with a time resolution of 1.59 s. Movie S1,† which shows colour oscillations in the five levitated droplets of 2× mixture, is included as a ESI.† Some spatial inhomogeneity in colour is visible in the levitated droplet in the direction perpendicular to the central axis of the levitator, suggesting that mixing in the droplet is not as efficient as in the stirred vial but is better than in the unstirred vial.
 |
| Fig. 4 Montage of images of a single droplet of 2× mixture undergoing oscillations with a time resolution of 1.59 s where the vertical direction is along the central axis of the levitator (images should be read from top left to right and then from top to bottom row, and the size of the box surrounding the droplet is 3.3 mm by 2.5 mm). | |
Two runs of five droplets of the 1× mixture were carried out. Movie S2,† which shows colour oscillations in the five levitated droplets of 1× mixture, is included as a ESI.† Fig. 5 shows the change in absorbance for the red, green and blue camera channels as a function of time for a typical levitated droplet of 1× mixture where, the first oscillation is not shown. The red channel shows very little change, as expected based on the observed colour changes. Both the green and blue channels show strong oscillations with a slow rise and rapid fall in absorbance. Similar behaviour was observed for all droplets of both the 1× and 2× mixtures.
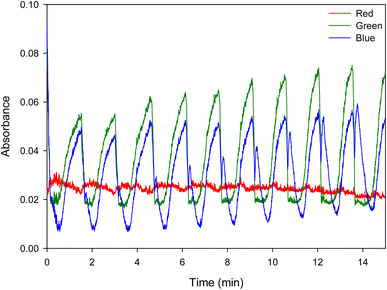 |
| Fig. 5 Plot of the change in absorbance of the red, green and blue channels against time for a typical levitated droplet of 1× mixture. | |
FFTs were taken of the entire time courses for the green channel for both runs of five droplets of 1× mixture as shown in Fig. 6(a). In addition, the FFTs of the green channel time courses in the unstirred and stirred vials are also shown in Fig. 6(a). The peaks in the FFTs of the droplet time courses for both runs were clustered together at a frequency of 0.702 ± 0.041 min−1. A t-test carried out between the oscillation frequencies of the two runs (unpaired, two tailed, homoscedastic) suggested that the two runs are not significantly different (p = 0.554). Further t-tests showed that the oscillation frequencies in both the unstirred and stirred vials were significantly different from the oscillation period in the levitated droplets. One possible explanation of the slower oscillation observed in droplets is evaporative cooling48 reducing the reaction rates as a result of the higher surface area to volume ratio of the droplets. Further work will be required to measure the droplet temperature to determine if this was the cause of the slower oscillations in droplets.
 |
| Fig. 6 (a) FFTs of the entire time courses for two runs with five droplets of 1× mixture levitated simultaneously and in the unstirred and stirred vials and (b) scatter plot with error bars (±1 standard deviation) of the oscillation period derived from the FFTs. | |
A further three runs where, in each run five droplets of 2× mixture were levitated simultaneously, were carried out and the time courses acquired. These in turn were Fourier transformed to determine the oscillation period. The results are summarized in Fig. 7, giving an overall average frequency of 0.814 ± 0.044 min−1. These results were compared to the results from the 1× mixture using a t-test (unpaired, two tailed, homoscedastic), which showed that the periods for 1× and 2× droplets were significantly different (p = 1.54 × 10−6). This is in accordance with the findings of Suzuki and Yoshida,49 who showed that increasing the concentrations of malonic acid and bromate increased the oscillation frequency.
 |
| Fig. 7 Scatter plot of the oscillation periods determined from the FFT of the time courses for three runs where, in each run five droplets of the 2× mixture were levitated simultaneously. | |
4 Conclusions
We have shown that it is possible to study oscillatory reactions such as the Belousov–Zhabotinsky (BZ) oscillator in acoustically levitated droplets. We have obtained results for the oscillation period in acoustically levitated droplets that are consistent with previous work carried out in cuvettes, test tubes, vials and beakers. We have also shown that it is possible to perform multiple simultaneous reactions with good reproducibility and repeatability, thus opening the possibility of using acoustic levitation for high-throughput experimentation. In addition, the solution volume used in the levitator was 4 μL compared to 3 mL in vials, a reduction by a factor of 750, making this method well suited for experimentation with high-cost materials.
This approach to performing oscillatory reactions in levitated droplets using the TinyLev levitator, which is readily available at relatively low cost (∼£15) as a kit of parts that can be assembled in a few hours using only a soldering iron and common laboratory tools. A complete experimental setup can be constructed using the TinyLev levitator, a mobile phone (to record images/video) and a low-cost LED light box of the type commonly used by artists (costing between £5–20). This means that the reported platform for acoustically levitated droplets is within reach of resource constrained laboratories.
Future work will focus on increasing the number of levitated droplets and automating the initial loading of the levitator and subsequent addition of droplets to minimise user interventions and errors.
Conflicts of interest
All authors declare no conflict of interest.
Author contributions
Songsaeng acquired data. Gupta and Goddard did conceptualization. Gupta did funding acquisition, project administration, resources, and supervision. All other activities were done by all authors. All authors have given approval to the final version of the manuscript.
Acknowledgements
This work was supported by the Leverhulme Trust [RPG-2021-137].
References
- H. Song, D. L. Chen and R. F. Ismagilov, Reactions in Droplets in Microfluidic Channels, Angew. Chem., Int. Ed., 2006, 45, 7336 CrossRef CAS PubMed.
- T. S. Kaminski and P. Garstecki, Controlled Droplet Microfluidic Systems for Multistep Chemical and Biological Assays, Chem. Soc. Rev., 2017, 46, 6210 RSC.
- Y. T. Zeng, J. W. Khor, T. L. van Neel, W. C. Tu, J. Berthier, S. Thongpang, E. Berthier and A. B. Theberge, Miniaturizing Chemistry and Biology using Droplets in Open Systems, Nat. Rev. Chem, 2023, 7, 439 CrossRef PubMed.
- W. Yuetong, C. Zhuoyue, B. Feika, S. Luoran, Z. Kaixuan, Z. Yuanjin, W. Yuetong, C. Zhuoyue, B. Feika, S. Luoran, Z. Kaixuan and Z. Yuanjin, Advances of Droplet-based Microfluidics in Drug Discovery, Expert Opin. Drug Discovery, 2020, 15, 969 CrossRef PubMed.
- H. Y. Geng and S. K. Cho, Dielectrowetting for Digital Microfluidics: Principle and Application. A Critical Review, Rev. Adhes. Adhes., 2017, 5, 268 CrossRef CAS.
- Y. Koroyasu, T.-V. Nguyen, S. Sasaguri, M. Marzo, I. Ezcurdia, Y. Nagata, T. Yamamoto, N. Nomura, T. Hoshi, Y. Ochiai and T. Fushimi, Microfluidic Platform Using Focused Ultrasound Passing Through Hydrophobic Meshes with Jump Availability, PNAS Nexus, 2023, 2, 207 CrossRef PubMed.
- H. Ong, H. Pang, J. Zhuo, R. Tao, P. Agarwal, H. Torun, K. Thummavichai, J. Luo, K. Tao, Q. Wu, H. Chang and Y.-Q. Fu, ZnO/Glass Thin Film Surface Acoustic Waves for Efficient Digital Acoustofluidics and Active Surface Cleaning, Mater. Chem. Phys., 2022, 287, 126290 CrossRef CAS.
- L. Shang, Y. Cheng and Y. Zhao, Emerging Droplet Microfluidics, Chem. Rev., 2017, 117, 7964 CrossRef CAS PubMed.
- T. Arai, T. Sato and T. Matsubara, Effective Cell Transfection in An Ultrasonically Levitated Droplet for Sustainable Technology, Adv. Sci., 2022, 9, 2203576 CrossRef CAS PubMed.
- M. A. Urbina, A. J. R. Watts and E. E. Reardon, Labs Should Cut Plastic Waste Too, Nature, 2015, 528, 479 CrossRef CAS PubMed.
- R. H. Morris, E. R. Dye, P. Docker and M. I. Newton, Beyond the Langevin Horn: Transducer Arrays for the Acoustic Levitation of Liquid Drops, Phys. Fluids, 2019, 31, 101301 CrossRef.
- S. Santesson and S. Nilsson, Airborne Chemistry: Acoustic Levitation in Chemical Analysis, Anal. Bioanal. Chem., 2004, 378, 1704 CrossRef CAS PubMed.
- P. F. Paradis, T. Ishikawa, G. W. Lee, D. Holland-Moritz, J. Brillo, W. K. Rhim and J. T. Okada, Materials Properties Measurements and Particle Beam Interactions Studies Using Electrostatic Levitation, Mater. Sci. Eng., R, 2014, 76, 1 CrossRef.
- K. A. Mirica, S. T. Phillips, C. R. Mace and G. M. Whitesides, Magnetic Levitation in the Analysis of Foods and Water, J. Agric. Food Chem., 2010, 58, 6565 CrossRef CAS PubMed.
- J. Park, A. H. Min, J. Theerthagiri, M. Ashokkumar and M. Y. Choi, In Situ Studies on Free-Standing Synthesis of Nanocatalysts via Acoustic Levitation Coupled with Pulsed Laser Irradiation, Ultrason. Sonochem., 2023, 94, 106345 CrossRef CAS PubMed.
- M. A. B. Andrade, A. Marzo and J. C. Adamowski, Acoustic Levitation in Mid-Air: Recent Advances, Challenges, and Future Perspectives, Appl. Phys. Lett., 2020, 116, 250501 CrossRef CAS.
- F. Zhang and Z. Jin, The Experiment of Acoustic Levitation and the Analysis by Simulation, Open Access Libr. J., 2018, 5, 1 Search PubMed.
- E. H. Brandt, Suspended by Sound, Nature, 2001, 413, 474 CrossRef CAS PubMed.
- E. Welter and B. Neidhart, Acoustically Levitated Droplets – A New Tool for Micro and Trace Analysis, Fresenius' J. Anal. Chem., 1997, 357, 345 CrossRef CAS.
- L. Cohen, M. I. Quant and D. J. Donaldson, Real-Time Measurements of pH Changes in Single, Acoustically Levitated Droplets Due to Atmospheric Multiphase Chemistry, Earth Space Chem., 2020, 4, 854 CrossRef CAS.
- T. Matsubara and K. Takemura, Containerless Bioorganic Reactions in a Floating Droplet by Levitation Technique Using an Ultrasonic Wave, Adv. Sci., 2021, 8, 2002780 CrossRef CAS PubMed.
- L. B. Bunio, J. Y. Wang, R. Kannaiyan and I. D. Gates, Evaporation and Crystallization of NaCl-Water Droplets Suspended in Air by Acoustic Levitation, Chem. Eng. Sci., 2022, 251, 117441 CrossRef CAS.
- D. D. Weis and J. D. Nardozzi, Enzyme Kinetics in Acoustically Levitated Droplets of Supercooled Water: A Novel Approach to Cryoenzymology, Anal. Chem., 2005, 77, 2558 CrossRef CAS PubMed.
- J. Chen, L. Hu, G. Hu, Y. Zhang, Y. Hu and J. Song, An Application of Chemical Oscillation: Distinguishing Two Isomers between Cyclohexane-1,3-dione and 1,4-cyclohexanedione, Electrochim. Acta, 2016, 195, 223 CrossRef CAS.
- A. Osypova, M. Dübner and G. Panzarasa, Oscillating Reactions Meet Polymers at Interfaces, Materials, 2020, 13, 2957 CrossRef CAS PubMed.
- A. Isakova and K. Novakovic, Oscillatory Chemical Reactions in the Quest for Rhythmic Motion of Smart Materials, Eur. Polym. J., 2017, 95, 430 CrossRef CAS.
- D. Papineau, K. Devine and B. A. Nogueira, Self-Similar Patterns from Abiotic Decarboxylation Metabolism through Chemically Oscillating Reactions: A Prebiotic Model for the Origin of Life, Life, 2022, 13, 551 CrossRef PubMed.
- J. N. Demas and D. Diemente, An Oscillating Chemical Reaction with a Luminescent Indicator, J. Chem. Educ., 1973, 50, 357 CrossRef CAS.
- R. Yoshida, Creation of Softmaterials Based on Self-Oscillating Polymer Gels, Polym. J., 2022, 54, 827 CrossRef CAS.
- G. A. Frerichs and D. Yengi, Complex Oscillations in the Belousov–Zhabotinsky Batch Reaction with Methylmalonic Acid and Manganese(II), RSC Adv., 2021, 11, 16435 RSC.
- R. J. Field, E. Koros and R. M. Noyes, Oscillations in Chemical Systems. II. Thorough Analysis of Temporal Oscillation in the Bromate–Cerium–Malonic Acid System, J. Am. Chem. Soc., 1972, 94, 8649 CrossRef CAS.
- R. M. Noyes, R. Field and E. Koros, Oscillations in Chemical Systems. I. Detailed Mechanism in a System Showing Temporal Oscillations, J. Am. Chem. Soc., 1972, 94, 1394 CrossRef CAS.
- J. Delgado, N. Li, M. Leda, H. O. González-Ochoa, S. Fraden and I. R. Epstein, Coupled Oscillations in a 1D Emulsion of Belousov–Zhabotinsky Droplets, Soft Matter, 2011, 7, 3155 RSC.
- J. Szymanski, J. N. Gorecka, Y. Igarashi, K. Gizynskia, J. Goreckia, K.-P. Zauner and M. de Planque, Droplets with Information Processing Ability, Int. J. Unconv. Comput., 2011, 7, 185 Search PubMed.
- H. Kitahata, N. Yoshinaga, K. H. Nagai and Y. Sumino, Spontaneous Motion of a Droplet Coupled with a Chemical Wave, Phys. Rev. E, 2011, 84, 015101 CrossRef PubMed.
- A. Marzo, A. Barnes and B. W. Drinkwater, TinyLev: A Multi-Emitter Single-Axis Acoustic Levitator, Rev. Sci. Instrum., 2017, 88, 085105 CrossRef PubMed.
- J. Li, W. D. Jamieson, P. Dimitriou, W. Xu, P. Rohde, B. Martinac, M. Baker, B. W. Drinkwater, O. K. Castell and D. A. Barrow, Building Programmable Multicompartment Artificial Cells Incorporating Remotely Activated Protein Channels Using Microfluidics and Acoustic Levitation, Nat. Commun., 2022, 13, 4125 CrossRef CAS PubMed.
- M. A. Meneses-Nava, I. Rosas-Roman, O. Barbosa-García, M. Rodriguez and J. L. Maldonado, Stability Evaluation of Water Droplets Levitated by a TinyLev Acoustic Levitator for Laser Induced Breakdown Spectroscopy, Spectrochim. Acta, Part B, 2020, 168, 105855 CrossRef CAS.
- A. McElligott, A. Guerra, M. J. Wood, A. D. Rey, A.-M. Kietzig and P. Servio, TinyLev Acoustically Levitated Water: Direct Observation of Collective, Inter-droplet Effects through Morphological and Thermal Analysis of Multiple Droplets, J. Colloid Interface Sci., 2022, 619, 84 CrossRef CAS PubMed.
- R. H. Morris, E. R. Dye, D. Axford, M. I. Newton, J. H. Beale and P. T. Docker, Non-Contact Universal Sample Presentation for Room Temperature Macromolecular Crystallography Using Acoustic Levitation, Sci. Rep., 2019, 9, 12431 CrossRef CAS PubMed.
- A. Watanabe, K. Hasegawa and Y. Abe, Contactless Fluid Manipulation in Air: Droplet Coalescence and Active Mixing by Acoustic Levitation, Sci. Rep., 2018, 8, 10221 CrossRef PubMed.
- P. Ruoff and B. Schwitters, Oscillations, Excitability and Stirring Effects in Closed Methylmalonic Acid Belousov-Zhabotinsky Systems, Z. Phys. Chem., 1983, 135, 171 CrossRef CAS.
- N. J. Goddard and R. Gupta, 3D Printed Analytical Platform for Automation of Fluid Manipulation Applied to Leaky Waveguide Biosensors, IEEE Trans. Instrum. Meas., 2021, 70, 7502612 Search PubMed.
- Y. Yamamoto, Y. Abe, A. Fujiwara, K. Hasegawa and K. Aoki, Internal Flow of Acoustically Levitated Droplet, Microgravity Sci. Technol., 2008, 20, 277 CrossRef.
- H. Bruus, Acoustofluidics 7: The Acoustic Radiation Force on Small Particles, Lab Chip, 2012, 12, 1014 RSC.
- A. Marzo, S. A. Seah, B. W. Drinkwater, D. R. Sahoo, B. Long and S. Subramanian, Holographic Acoustic Elements for Manipulation of Levitated Objects, Nat. Commun., 2015, 6, 8661 CrossRef CAS PubMed.
- L. P. Gor'kov, On the Forces Acting on a Small Particle in an Acoustical Field in an Ideal Fluid, Soviet Phys. Dokl., 1962, 6, 773 Search PubMed.
- A. L. Yarin, G. Brenn, O. Kastner, D. Rensik and C. Tropea, Evaporation of Acoustically Levitated Droplets, J. Fluid Mech., 1999, 399, 151 CrossRef CAS.
- D. Suzuki and R. Yoshida, Effect of Initial Substrate Concentration of the Belousov–Zhabotinsky Reaction on Self-Oscillation for Microgel System, J. Phys. Chem. B, 2008, 112, 12618 CrossRef CAS PubMed.
|
This journal is © The Royal Society of Chemistry 2023 |