DOI:
10.1039/D3RA06299F
(Paper)
RSC Adv., 2023,
13, 34598-34609
A heterostructural MoS2QDs@UiO-66 nanocomposite for the highly efficient photocatalytic degradation of methylene blue under visible light and simulated sunlight†
Received
15th September 2023
, Accepted 2nd November 2023
First published on 27th November 2023
Abstract
The development of recyclable photocatalysts with high activity and stability has piqued the interest of researchers in the field of wastewater treatment. In this study, an ultrasonic probe approach was used to immerse a sequence of heterojunctions formed by metal–organic frameworks (UiO-66) and different amounts of molybdenum disulfide quantum dots (MoS2QDs), resulting in a highly recyclable MoS2QDs@UiO-66 photocatalyst. Multiple advanced techniques, such as XPS, XRD, TEM, XRF, and UV-vis spectrophotometry, were used to characterize and confirm the successful preparation of UIO-66 impregnated with MoS2QDs. The results indicated that the best heterostructure catalyst exhibited superior efficiency in the photocatalytic degradation of methylene blue (MB) in water, achieving approximately 99% removal within 30 minutes under simulated sunlight, while approximately 97% removal under visible light. The outstanding photocatalytic performance is predominantly attributed to the photoinduced separation of carriers in this heterostructure system. This study proposes a unique, simple, and low-cost method for improving the degradation performance of organic contaminants in water.
1. Introduction
Photocatalysis of the semiconductor material is a powerful process for environmental detoxification that converts hazardous and non-biodegradable organic molecules to carbon dioxide, inorganic salts, and water.1–5 Metal–organic frameworks (MOFs), which are porous structures consisting of metal building units and organic bridging ligands, have been identified as possible photocatalysts.6,7 Metal–organic frameworks (MOFs) with high porosity display semiconducting properties when exposed to sunlight, suggesting they might be used as photocatalysts.5,8,9 As a result of their semiconducting nature, they might be viable options for wastewater treatment. Additionally, their catalytic capabilities can be used for the environment-friendly reduction of hazardous chemical compounds. Most MOFs cannot absorb visible light or do not have enough catalytic sites to photocatalytically produce H2.10,11 Two typical methods have been used for the production of photocatalytically active MOFs. The first method is doping MOFs with light-absorbing metal complexes. The second method involves including light-absorbing organic building blocks into MOFs, for instance, porphyrins and 2-amino terephthalate, to capture visible light. Water pollution degradation, water splitting, CO2 reduction, and organic synthesis are examples of photocatalytic MOF solar-driven applications.12,13
UiOs are classified as a stable group of MOFs. The zirconium-based MOF UiO-66 exhibits high porosity14,15 as well as has high thermal, water, and acid stability (acetone, benzene, and DMF). UiO-66 is extremely versatile in its functionalization and application. However, the adsorption of dyes from solution on MOFs has rarely been studied.16,17 Moreover, UiO-66, compared to other inorganic catalysts, fails to reach reasonable photocatalytic performances owing to light absorption. Thus, to enhance the efficiency of the UiO-66 family photocatalyst, several composites, such as CdSe quantum dots, have been added to UiO-66 to remove the RHB dye. In addition, nanoparticles of Au were added to the pure sample. The above results signify that the UiO-66 analogies are worthy candidates as photocatalysts. Also, other QDs@MOF composite photocatalysts have been reported, such as CdS/MIL-101,18 CdS/MIL-125, and MoS2QDs@UiO-66–NH2/G, where MoS2QDs effectively increased the photo-generated charge carriers and electron transport, resulting in a significant increase in photocatalytic hydrogen evolution.19 Therefore, it can be supposed that MoS2QDs and UiO-66 can complement each other to overcome the limitations of individual materials. Since Mo is a mandatory element for both plants and animals, this technique is environment-friendly and is appropriate for large-scale preparation.20
Here, we first describe the use of UiO-66@MoS2QDs as a photocatalyst with visible-light activity and its application in water treatment. The primary goal of this research is to create a UiO-66@MoS2QDs compound with desirable photocatalytic capabilities and adequate stability for organic dye degradation. The UiO-66@MoS2QDs photocatalyst was created using a straightforward solution approach in which UiO-66 served as both, a substrate and a mediator, for the synthesis of MoS2QDs. The photocatalytic activity of UiO-66@MoS2QDs photocatalyst was tested by degrading MB under both simulated and real sunlight irradiation. In addition, the photocatalytic mechanism and photocatalyst recycling are discussed.
2. Experimental method
2.1. Chemicals
1,4-benzenedicarboxylic acid, 1,4-naphthalenedicarboxylic acid, zirconium tetrachloride, terephthalic acid (H2BDC), N,N-dimethylformamide (DMF), benzoic acid (BA), ammonium molybdate, thiourea, isopropanol, and MB were acquired from Alfa Aesar and utilized without additional purification.
2.2. Characterizations tools
The elemental structure and binding behavior of the UiO-66@MoS2QDs photocatalyst were analyzed using X-ray photoelectron spectroscopy (XPS; Thermo-Fisher Sci., USA). The structural properties of UiO-66@MoS2QDs samples were studied using X-ray diffraction (XRD), a D2 Phaser instrument, employing CuKα radiation (wavelength is equal to 1.540598 Å). The microstructure of these samples was investigated using TEM on the FEI Tecnai G2 Spirit TWIN instrument operating at 80 kV. The elemental chemical analysis of UiO-66@MoS2QDs samples was examined employing a Bruker M4-Tornado X-ray fluorescence (XRF) instrument. The energy gap was computed by measuring the absorbance using a Shimadzu UV-2600 instrument with λ ranging from 190 to 900 nm.
2.3. Synthesis of UiO-66@MoS2QDs photocatalyst
UiO-66 was synthesized following our previous report.21 ZrCl4 was dispersed in DMF and some drops of deionized water were added to the reaction. Then, benzoic acid was added until a clear solution was achieved. The clear solution was placed in a preheated oven for crystallization at ∼120 °C for one day. The as-prepared MoS2 was synthesized based on our previous work.22 To fabricate UiO-66 impregnated by MoS2QDs, a simple approach of ultrasonic mixing route was used; firstly, 50 mg of UiO-66 was well dispersed in 30 mL of deionized water. Different concentrations of MoS2QDs were added, and the mixture was then exposed to ultrasonic waves for a further one hour. The product was washed several times and then dried overnight at 60 °C to obtain UiO-66@MoS2QDs.
2.4. The photocatalytic performance of UiO-66@MoS2QDs
Under UV irradiation, photocatalytic studies of UiO-66@MoS2QDs were evaluated for MB degradation. In the typical experimental methodology, 25 mg of UiO-66@MoS2QDs was contacted with 50 mL of MB-dye (10 ppm) solution. When the filtration was completed, the material was centrifuged at 5000 rpm for 10 min before examining it using UV-vis spectroscopy. The UiO-66@MoS2QDs photocatalyst and the solution of MB were mixed for 30 min in the dark box before exposure to radiation to complete the adsorption process and desorption equilibrium. UiO-66@MoS2QDs adsorbed with MB molecules resulted in a forthright reaction of MB with electron plus hole pairs. A closed dark reactor was employed, with a 10 cm gap between the UV lamp and the MB–UiO-66@MoS2QDs beaker. To measure the catalytic effect under real sung light for MB,23,24 50 mg of the photocatalyst was added to 0.010 L of the MB dye solution (10 mg L−1) in the reactor independently. Before irradiation, we followed the same protocol for the simulated sunlight since the mixture was mixed for 180 seconds in the dark to realize an adsorption/desorption equilibrium for the dye as well as photocatalysts. After half an hour in the dark, the solution was exposed to sunlight under continuous stirring. The photocatalysis experiment involving the MB dye was performed outside in the solar light on a sunny day in October from 12 a.m. and 3 p.m. where the solar intensity was 7.2–7.8 kW h per m2 per day at the Al-Azhar University, Assiut, Egypt. At a specified time interval, ∼3 mL of the solution was separated from the reactor and centrifuged to separate the catalysts by analysis. The absorbance of the MB solution at 665 nm was also measured to test MB photodegradation. To compute the efficiency of photodegradation/removal, the following equation was used:25
Following the completion of the photocatalytic degradation process, the starting and final MB concentrations (Ci and Cf) were calculated. A series of photocatalytic experiments were conducted to determine the effects of pH, contact length, dose, and temperature on photocatalytic activity. After the photocatalytic procedure was completed, the consumed UiO-66@MoS2QDs was collected and mixed with 0.1 M HNO3 before rinsing twice with DI water under ultrasonic conditions to eliminate any residual MB. The regenerated photocatalyst UiO-66@MoS2QDs can be used up to six times with outstanding efficiency.
3. Result and discussion
3.1. Characterization of the UiO-66@MoS2QDs photocatalyst
The refined powder XRDs of pristine UiO-66 and UiO-66 coated with different concentrations of MoS2 quantum dots are shown in Fig. 1. X-ray diffraction proved that all samples have a single cubic phase (Fm
m) according to the information card for entry #4512072 (crystallography open database). All peaks were indexed for UiO-66 without any impurities from other peaks linked to other phases. Moreover, for UiO-66@MoS2QDs, no additional peaks correspond to MoS2 due to the small amount of MoS2. As shown in Fig. 2a–f, the size and microstructure of the pure UiO-66 NPs, as well as the UiO-66 coating with varied MoS2QD levels, were determined by TEM. According to Fig. 2a, the average size of the smooth cubic crystal of pure UiO-66 was less than 100 nm, and the average size for all samples was less than 100 nm. Although the nanoparticles of UiO-66 are small, they seem to be agglomerated and have a large particle size. Additionally, TEM images of UiO-66@MoS2QD nanocomposites displayed that the QDS of the MoS2 was efficiently and successfully connected to the UiO-66 surface, which benefitted the charge carrier transfer in the heterostructures, as a result, it could enhance the photocatalytic activity of the UiO-66@MoS2QDs nanocomposites, as illustrated in Fig. 3b–f. For further confirmation of MoS2, the samples were analyzed by XRF, and the concentrations of each element were exhibited, as shown in Table 1.
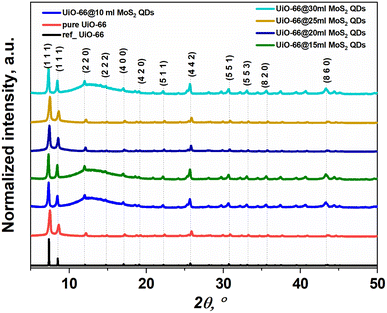 |
| Fig. 1 The XRD powder patterns of the samples ref UiO-66 (the information card for entry #4512072 (crystallography open database)), pure UiO-66, and UiO-66@MoS2QDs at different amounts of MoS2QDs (10, 15, 20, 25, and 30 mL). | |
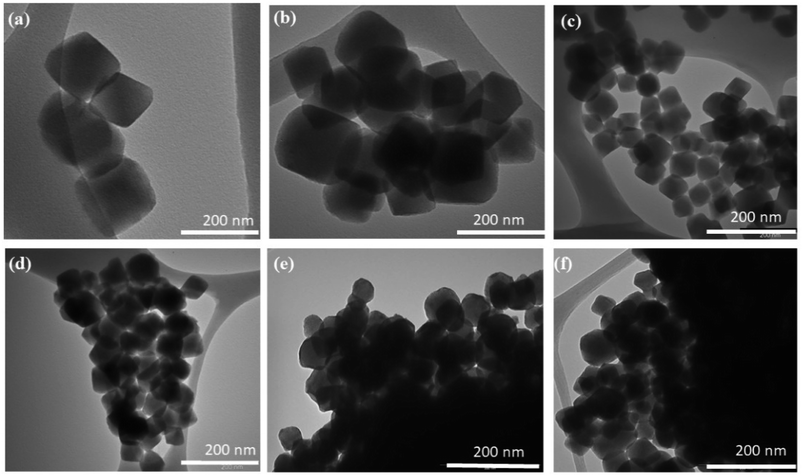 |
| Fig. 2 The TEM images of the samples (a) pure-UiO-66, (b) 10 mL UiO-66@MoS2QDs, (c) 15 mL UiO-66@MoS2QDs, (d) 20 mL UiO-66@MoS2QDs, (e) 25 mL UiO-66@MoS2QDs, and (f) 30 mL UiO-66@MoS2QDs. | |
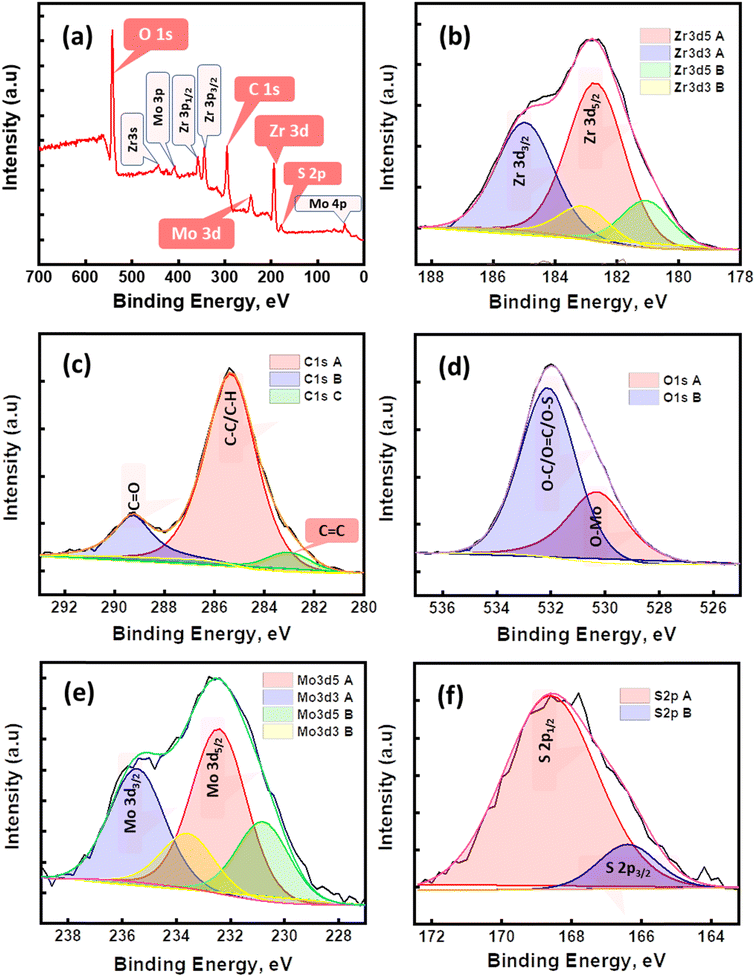 |
| Fig. 3 The XPS spectra of 15 mL UiO-66@MoS2QDs: (a) survey spectra, (b) Zr 3d, (c) C 1s, (d) O 1s, (e) Mo 3d, and (f) S 2p. | |
Table 1 The element analysis of UiO-66 coatings at different concentrations of MoS2QDs by XRF
Sample |
Element |
Atomic ratios (at%) |
UiO-66 |
Zr |
100 |
10 mL MoS2QDs@UiO-66 |
Zr |
74.89 |
Mo |
6.28 |
S |
18.83 |
15 mL MoS2QDs@UiO-66 |
Zr |
54.76 |
Mo |
8.64 |
S |
36.60 |
20 mL MoS2QDs@UiO-66 |
Zr |
51.38 |
Mo |
9.64 |
S |
38.98 |
25 mL MoS2QDs@UiO-66 |
Zr |
47.8 |
Mo |
10.65 |
S |
41.55 |
30 mL MoS2QDs@UiO-66 |
Zr |
44.3 |
Mo |
11.62 |
S |
44.08 |
XPS analysis was carried out to explore the surface valence state plus the elemental structure of the 15 mL UiO-66@MoS2QDs photocatalyst, as shown in Fig. 3. The survey spectrum (Fig. 3a) proved the elemental components of MoS2QDs@UiO-66, where the peaks of zirconium (Zr 3d), carbon (C 1s), oxygen (O 1s), molybdenum (Mo 3d), and sulfur (S 2p) were obtained, proving the presence of MoS2QDs along the surface of UiO-66 matrix. The peaks shown in Fig. 3b, at the binding energies of 184.98 eV and 182.7 eV, could be attributed to Zr 3d3/2 and Zr 3d5/2 of UiO-66 in the UiO-66@MoS2QDs photocatalyst, respectively. In the C 1s spectrum (Fig. 3c), the C
O, C–C/C–H, and C
C bonds appeared at 289.27 eV, 285.3 eV, and 282.9 eV, respectively. Fig. 3d shows two clear peaks at 530.2 and 532.1 eV in the O 1s spectrum, these peaks could be named the O–Mo bonds and O–C/O
C/O–S bonds as a result of MoS2QDs grafting with the UiO-66 platform. The Mo 3d spectrum data is shown in Fig. 3e. Two characteristic peaks at 235.4 eV (Mo 3d3/2) and 232.4 eV (Mo 3d5/2) are attributed to the Mo(IV) oxidation states of MoS2. On the other hand, there are two additional weak peaks at 233.5 eV (Mo 3d3/2), and 230.8 eV (Mo 3d5/2) attached to the Mo(VI) oxidation state. The peaks of S 2p1/2 and S 2p3/2 binding energies were presented at 168.6 eV and 166.3 eV, respectively, relating to the characteristic values for S2−, as shown in Fig. 3f. These obtained results demonstrated that UiO-66@MoS2QDs photocatalysts were successfully fabricated.
3.2. Effect of impregnated MoS2QDs amounts
The photocatalytic activity of UiO-66@MoS2QDs was assessed by varying the quantity of additional MoS2QDs added. The UV-vis absorption spectra of MB (at λmax 665 nm) before and after stirring with UiO-66 and UiO-66@MoS2QDs are explained in Fig. 4a–f. With the increasing exposure time, the intensity of the MB peak was decreased. The photocatalytic performance of UiO-66@MoS2QDs composites is superior to that of pure UiO-66. Fig. 4a shows that the effectiveness of MB degradation by UiO-66's was approximately 49.5%. In the case of the UiO-66@MoS2QDs photocatalyst, the photocatalytic performance improved with an increase in the MoS2QDs contents, where the MB-degradation efficiency was increased to approximately 99% in the case of UiO-66@MoS2QDs (15 mL) (Fig. 4b and c). Fig. 4d and f show that the photocatalytic performance of the photocatalyst towards MB dye decreased upon increasing the number of MoS2QDs. Initially, the photocatalytic degradation of MB was improved by the addition of a modest quantity of MoS2QDs; however, this effect was quickly reversed by an increase in the MoS2QDs content. However, the UiO-66@MoS2QDs experiment had too many MoS2QDs. The higher amount of photo-generated electrons is responsible for this. The photocatalytic performance was, however, reduced as the number of MoS2QDs increased. Because of the extra MoS2QDs, the composites are inclined to aggregation.26 As a result, the high concentrations of MoS2QDs in a composite may probably increase the likelihood of recombination of photo-generated electron–hole pairs in the composite.27 As a result of this test, 15 mL UiO-66@MoS2QDs was selected for the next experiments.
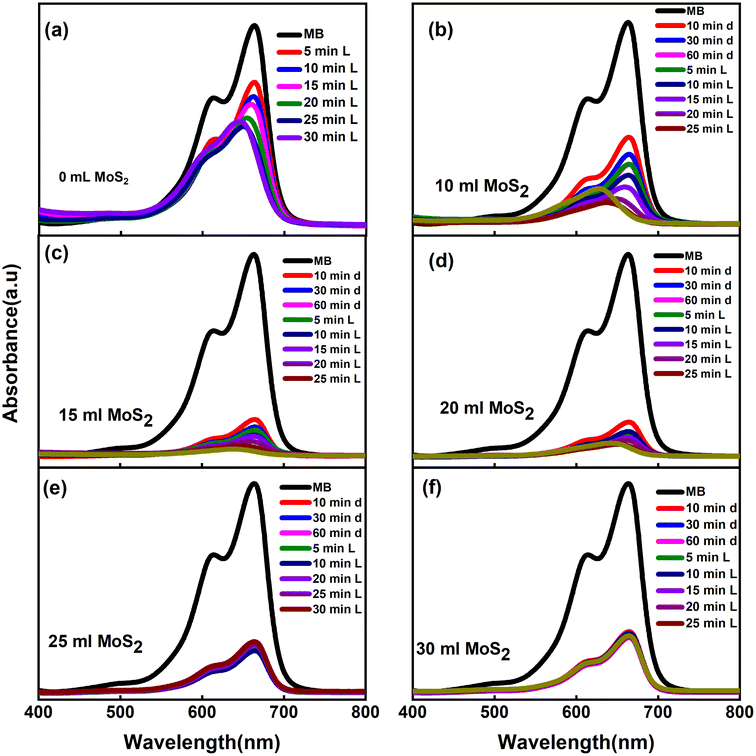 |
| Fig. 4 (a) The UV-vis absorption spectrum of UiO-66, and (b–f) UV-vis absorption spectra of UiO-66@MoS2QDs at different concentrations of MoS2QDs (10, 15, 20, 25, and 30 mL). | |
3.3. Optimizing of the MB-photocatalytic decomposition
The precise mechanism by which photocatalyst degrades the MB dye is unknown because it is dependent on various experimental setup parameters, such as the pH of the solution, photocatalyst dosage, solution temperature, period of irradiation, the concentration of MB-pollutant, and presence of co-existing ions. The primary objective of this work is to validate how the factors listed above affect the efficacy of MB degradation under UV irradiation.
The pH of the photocatalytic medium influences the % photocatalytic degradation efficiency owing to it altering the surface charge of UiO-66@MoS2QDs during ionization of the surface functional groups. The percent degradation efficiency of photocatalysis is represented as a percentage. The anionic dye molecule is transformed into anionic chloride ions, in this example, which are subsequently converted back to the anionic dye molecules. As a consequence, MB exists as a positively charged ion when the pH is higher than seven.28 When 25 mg of UiO-66@MoS2QDs was mixed in fifty ml of MB-solution (ten ppm) for 30 minutes at RT, it was discovered that the solution's pH used influenced the photocatalytic degradation of MB. It was necessary to employ buffer solutions to adjust the pH of the MB solutions from 2 to 10. When the pH of the photocatalytic medium was increased, it was found that the MB quantity was reduced by the photocatalytic medium (as shown in Fig. 5a). The outer surface of MoS2QDs@UiO-66 was transformed into a charged surface when the pH was raised to 7. It is hypothesized that these protonated active sites would inhibit the interaction of cationic MB molecules with UiO-66@MoS2QDs, hence slowing down MB degradation. When pH levels are acidic, it has been shown that the breakdown rate of the cationic MB molecules is lowered as a result of competition between H+ ions and MB molecules for available active sites.29 As the pH is reduced below 7, the surface of the UiO-66@MoS2QDs becomes negative, increasing the adsorption of cationic MB-molecules, and hence the proportion of the MB-dye is destroyed. This resulted in a favorable breakdown of MB using UiO-66@MoS2QDs in an alkaline pH medium. Because the difference between pH 7 and pH 7 is very small, it was established that pH 7.0 gave the best MB degradation; hence, more photocatalytic tests were conducted at this pH value.
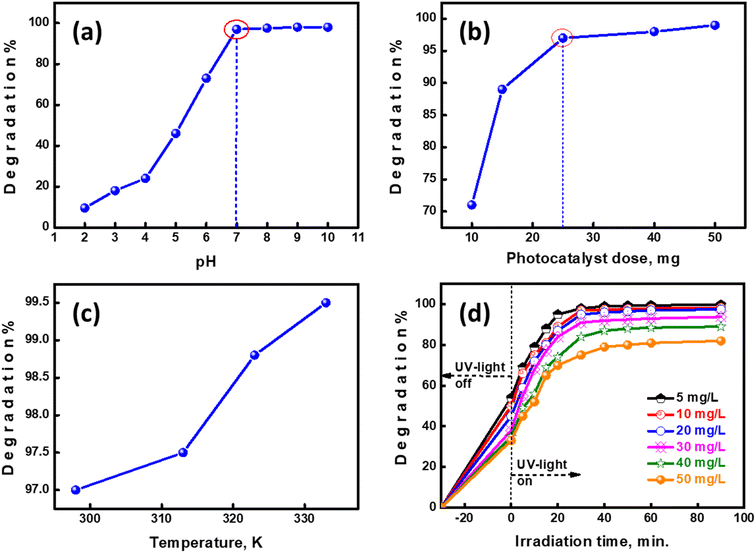 |
| Fig. 5 The effect of (a) pH, (b) photocatalyst dose, (c) temperature, and (d) CV-concentration on the MB-photocatalytic degradation using UiO-66@MoS2QDs. | |
The quantity of the applied photocatalyst affects the photocatalytic activity and MB degradation. This was performed by increasing the amount of UiO-66@MoS2QDs from 10 to 50 mg, as shown in Fig. 5b, while maintaining the same MB concentration (10 ppm). The MB-dye solution was spun and irradiated at different concentrations of the photocatalyst for 30 minutes under UV light at pH 7. By increasing the dose of UiO-66@MoS2QDs, the % photodegradation was increased. Due to the reduced amount of the utilized photocatalyst, the photodegradation percentage was lower due to the deficiency of the surface-active sites in the photocatalyst. Increases in the dosage of UiO-66@MoS2QDs result in a rise in the UV-exposed area, which results in an increase in the number of photons absorbed by UiO-66@MoS2QDs and the quantity of the adsorbed MB-molecules in the system. 25 mg of UiO-66@MoS2QDs displayed good photocatalytic activity under this condition. In future, the UiO-66@MoS2QDs weight will be eliminated, resulting in a slight increase in catalytic activity. As a consequence, the MB-photodegradation experiment required only 25 mg of UiO-66@MoS2QDs.
Increased decolorization and removal efficiency may be achieved with increasing temperature. As demonstrated in Fig. 5c, the temperature had a significant impact on MB degradation's performance. By increasing the system temperature (from 298 to 333 K), the degradation percentage of MB increased from 97% to 99.5%. A possible explanation for this trend is an increase in the dispersion and crashing of the key radicals and ions due to the increase in the temperature of the system, which increases the percentage of MB degradation. Another factor that influences the creation of OH is temperature, which may either speed up or slow it down.30 Since there is only a 2.5% difference in the MB degrading efficiency between 298 K and a high temperature, the high temperature might not be profitable because of the additional heat energy costs.
While leaving other parameters constant, such as 25 mg UiO-66@MoS2QDs dosage, 50 mL MB-solution, pH 7, and room temperature, we examined the effect of a lower initial MB concentration (5 to 50 ppm). As exhibited in Fig. 5d, the preliminary MB concentration increased as the MB decolourization percentage reduced. Because the path length of photons through the MB's solution was reduced and the active surface sites of UiO-66@MoS2QDs were protected, this behaviour is adequate. This process results in a reduction in the ultraviolet radiation amount absorbed using MoS2QDs@UiO-66 particles, which in turn decreases the electron–hole pair formation (i.e., reactive radicals), lowering the amount MB photodegradation%.31 This means that the solution of the MB dye with a UiO-66@MoS2QDs photocatalyst should have a concentration of 10 ppm or less. Fig. S1† shows that the MB-adsorption capacity of UiO-66@MoS2QDs was enhanced with an extended initial concentration of MB until it reached the maximum adsorption capacity. The adsorbed amount of MB was found to be 46.5 mg g−1 using the following equation qe (mg g−1) = (Ci − Cf)(V/m). Where Ci and Cf (ppm) are the initial and final MB concentrations; V is the volume of the MB solution (L), and w (g) is the mass of UiO-66@MoS2QDs.
3.4. Mechanism of MB-photocatalytic degradation
To examine the effect of MoS2QDs on the optical properties of the MoS2QDs@UiO-66 photocatalyst, UV-vis spectra of the pure UiO-66 and UiO-66 coatings with different ratios of MoS2QDs heterostructure were investigated. Nevertheless, the UiO-66@MoS2QDs spectrum showed a change in the absorption at the UV-vis region, indicating that the quantum dot MoS2 effect on the absorption spectrum, mainly in the visible region of 400–600 nm. The energy gap (Eg) of all the active materials was computed utilizing Tauc's relation.32 MoS2QDs influence the energy gap of the UiO-66 and range from 3.66 to 4.05 eV, as shown in Fig. 6b. The absorption enhancement of the UiO-66@MoS2QDs nanocomposites in the visible region may improve the photoelectron–hole pairs generation that takes part in the reaction, which is appropriate for the photocatalytic route.
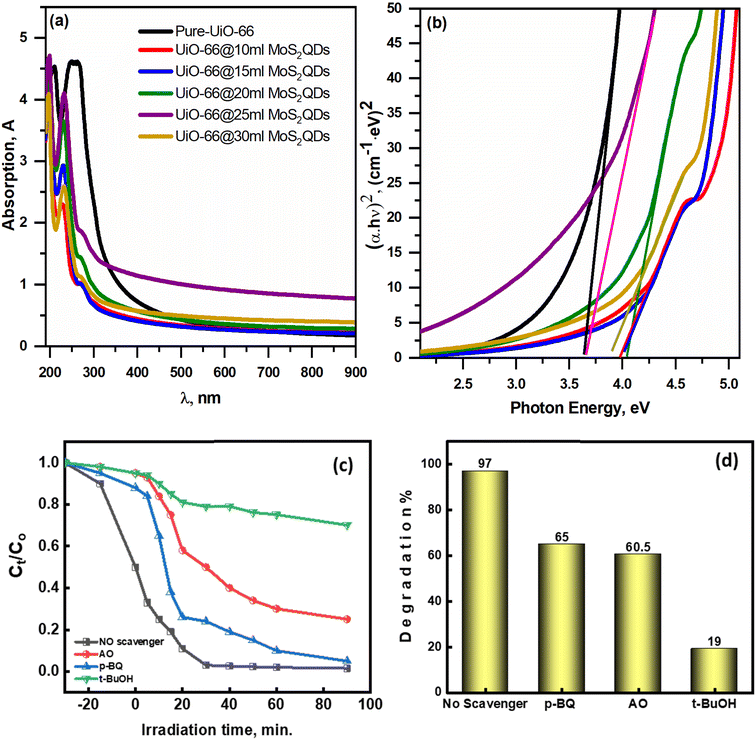 |
| Fig. 6 (a) The UV-vis spectra and (b) Tauc plots of pure UiO-66 NPs and UiO-66@MoS2QDs nanocomposites. The impact of various scavengers on the photocatalytic degradation of MB dye (c and d). | |
As a consequence of the exposure of the UiO-66@MoS2QDs photocatalyst to ultraviolet light with hν higher than the Eg of UiO-66@MoS2QDs, electrons (e−) in the VB can be transferred to a higher energy level (CB), resulting in the formation of positive holes (h+) at the VB level. Electron–hole recombination is reduced, which is a crucial component in improving the photocatalytic efficiency of the system. The e− and h+ ions can be used to begin reactions between activated-e− and oxygen, which can result in the creation of a free radical such as O2˙− in the presence of oxygen. This reaction resulted in the formation of free radicals (OH˙), which are beneficial for the photocatalytic degradation of MB because they have a high oxidative capacity, which allows for the breakdown of larger molecules into smaller molecules and less damaging and harmless species, such as CO2 and H2O, are formed.33 Superoxide can enhance photodegradation by preventing pair recombination of e−–h+ couples. To investigate the active species responsible for MB-photocatalytic degradation using UiO-66@MoS2QDs, many tests were performed using several scavengers, such as p-benzoquinone (p-BQ), tert-butyl alcohol (t-BuOH), and ammonium oxalate (AO) to trap O2˙− OH˙ radicals, and h+, respectively. p-BQ, AO, and t-BuOH were added to the MB solution under optimal degradation conditions. Fig. 6c and d suggested that adding AO and p-BQ has little effect on the MB degradation performance, referring to h+ and O2˙− radicals having no significant impact on MB degradation. On the other hand, adding t-BuOH clearly decreases the MB-degradation rate. Therefore, OH˙ radicals are the main active species in the photocatalytic degradation of MB dye. The photodegradation of UiO-66@MoS2QDs may be characterized using the following equations:
UiO-66@MoS2QDs-66 + hν → UiO-66@MoS2QDs + e− + h+ |
O2˙− + HO˙ + H+ + MB → micromolecules + CO2 + H2O |
3.5. Kinetic study of the photocatalytic degradation of MB dye
To explore the kinetic of MB-photocatalytic degradation process by UiO-66@MoS2QDs, pseudo 1st and 2nd order kinetics styles were studied at the optimum degradation conditions (dose 25 mg, volume 50 mL, concentration 10 ppm, and pH 7), where temperature and irradiation time were variables. The pseudo 1st and 2nd order kinetics models can be demonstrated in the following reactions:23,33
The pseudo-first- and second-order rate constants are indicated by the letters K1 (min−1) and K2 (g mg−1 min−1), respectively. °C represents the initial MB concentration, whereas Ct represents the cumulative MB concentration (t, min). To establish the % photodegradation of MB dye at various temperatures, researchers examined photodegradation kinetic models, such as those shown in Fig. 7a and b. The correlation coefficients (R2), rate constants (K1 and K2), and half-life time associated with the fitted prototypes are listed in Table 2. The half-life time (t0.5, min) for pseudo-1st and 2nd orders may be determined using the (0.693/K1) and (1/K2Co) formulas, respectively. The R2 value of the pseudo-first-order degradation kinetic prototype (ln(Co/Ct) vs. t) was much larger than that of the pseudo-second-order model (1/Ct vs. t), indicating that the pseudo-first-order model was more accurate. As a consequence, the pseudo-first-order model can properly represent the MB-photodegradation of UiO-66@MoS2QDs in this study. The MB-degradation rate constant of UiO-66@MoS2QDs at 333 K is higher than that of UiO-66@MoS2QDs at 323 K > 313 K > 298 K, showing better photocatalytic activity of MB dye under UV-irradiation at high temperature.
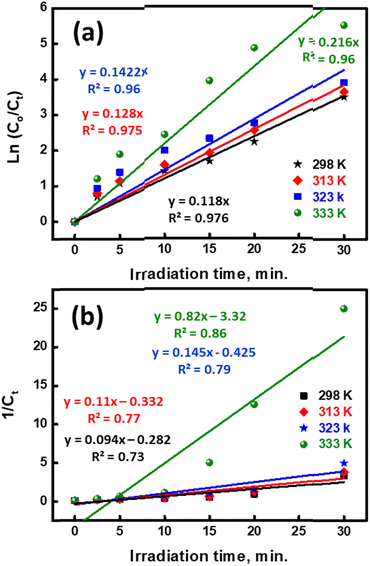 |
| Fig. 7 The kinetic study of MB-photodegradation using UiO-66@MoS2QDs through pseudo 1st order (a) and pseudo 2nd order (b) kinetic models. | |
Table 2 The parameters of pseudo 1st order (A) and pseudo 2nd order (B) kinetic models for MB-photodegradation using the UiO-66@MoS2QDs photocatalyst
|
Temperature K |
R2 |
Rate constant (K) |
T0.5, min |
Pseudo 1st order |
298 |
0.976 |
0.118 |
5.87 |
313 |
0.975 |
0.128 |
5.41 |
323 |
0.96 |
0.1422 |
4.87 |
333 |
0.96 |
0.216 |
3.2 |
Pseudo 2nd order |
298 |
0.73 |
0.094 |
1.06 |
313 |
0.77 |
0.11 |
0.91 |
323 |
0.79 |
0.145 |
0.69 |
333 |
0.86 |
0.82 |
0.122 |
3.6. Thermodynamic study of MB photocatalytic degradation
To compute the activation energy (Ea) and other parameters of the thermodynamics, we utilized the fluctuation of K1 with temperature (303, 313, 323, and 333 K). The Arrhenius equation was utilized to compute the degradation's activation energy:34
Here, Kapp is the constant of the apparent rate (min−1), R is Arrhenius (min−1), A is the universal gas constant (J mol−1 K−1), and T is the temperature (K). A straight line was drawn between ln(Kapp) and 1/T, and from its slope, we were able to compute the activation energy, as illustrated in Fig. 8a. The activation energy was 12.927 kJ mol−1, which is a moderate amount. This indicated that the oxidative process had a low barrier to entry. The photocatalytic decomposition of MB is physical in nature since activation energies of less than 20 kJ mol−1 were found in the experiment findings.35 The following equations were used to calculate the thermodynamic characteristics based on the results from the degradation tests at 298, 313, 323, and 333 K:34
where KB is a Boltzmann constant 1.3806 × 10−23 m2 kg s−2 K−1. ΔH°, ΔS°, and ΔG° are enthalpy (J mol−1), entropy (J mol−1 K−1) and Gibbs free energy (J mol−1), respectively, while ℏ is the Planck constant 6.626 × 10−34 m2 kg s−1. To calculate ΔH°, ΔS°, and ΔG° values, ln(Kapp/T) was plotted versus (1/T), as shown in Fig. 8b. The obtained data of the thermodynamic parameters are given in Table 3. The reduction in unpredictability in the system where deterioration occurred is shown by the negative ΔS° value (−228.47 J mol−1 K−1). As an alternative, due to the positive enthalpy values (10.31 kJ mol−1), the MB degrading process utilizing UiO-66@MoS2QDs photocatalyst is endothermic. The negative value of ΔG° specifies that the photocatalytic degradation process is a non-spontaneous process, where the MB-degradation process requires an input of energy to take place.
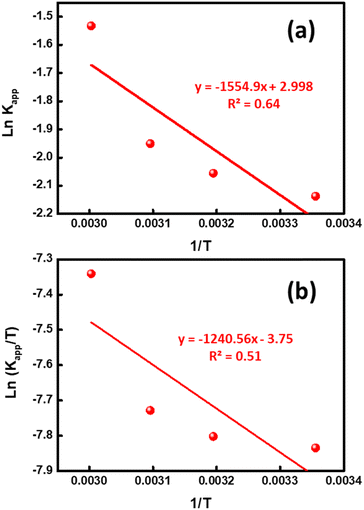 |
| Fig. 8 (a) The plots of ln Kapp vs. 1/T and (b) ln(Kapp/T) vs. 1/T for the MB degradation using the UiO-66@MoS2QDs photocatalyst. | |
Table 3 The thermodynamic parameters for MB degradation using the UiO-66@MoS2QDs photocatalyst
Temperature K |
The rate constant (K) |
R2 |
Ea, kJ mol−1 |
ΔH, kJ mol−1 |
ΔS, J mol−1 K−1 |
ΔG, kJ mol−1 |
298 |
0.118 |
0.976 |
12.927 |
10.314 |
−228.47 |
78.398 |
313 |
0.128 |
0.975 |
81.825 |
323 |
0.1422 |
0.96 |
84.109 |
333 |
0.216 |
0.96 |
86.394 |
3.7. Recycling and reusability of the spent UiO-66@MoS2QDs photocatalyst
Photocatalytic degradation may be employed to renew and reuse the wasted UiO-66@MoS2QDs photocatalyst, hence reducing the total cost of the MB removal process. Thus, the recovery of the spent UiO-66@MoS2QDs photocatalyst was evaluated using centrifugation, decanting, and washing with deionized water. The ultrasonic and 0.1 M HNO3 washing conditions ensured that the MB dye was eliminated and decaying components were removed during the photocatalytic process. Drying at 80 °C was used to remove water from the final product. The regenerated UiO-66@MoS2QDs can be utilized again for a fresh cycle of regeneration. The photostability of the UiO-66@MoS2QDs was tested over six cycles. Using the hetero-system shown in Fig. 9a, the activity of the UiO-66@MoS2QDs structure decreased by less than 10% after the fourth cycle, illustrating the extremely reusable nature of the material. There may be a concentration of the pollutant molecules on the active sites on the surface of the UiO-66@MoS2QDs that is reducing photodegradation. To be more realistic, we applied the photocatalytic performance of the UiO-66 coated with different concentrations of MoS2QDs in real sunlight. Since the experiment of the photocatalyst of MB dye was performed under outside solar light on a sunny day in October from 12 a.m. and 3 p.m., where the solar intensity is 7.2–7.8 kW h per m2 per day in Al-Azhar University, Assiut, Egypt, there was no significant difference between both simulated and real sunlight. The best concentration was 15 mL MoS2 QDs for removal on that day, and it was around 99% for simulated sunlight and 97% for real sunlight, as demonstrated in Fig. 9b.
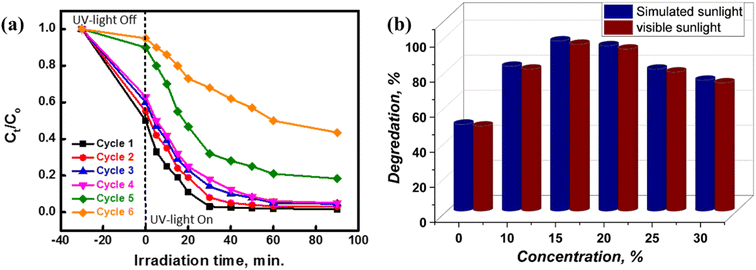 |
| Fig. 9 (a) Recycling of the spent UiO-66@MoS2QDs for six reuse cycles and (b) comparison between the degradation efficiency of the catalytic activity at different concentrations of MoS2 QDs coated with UiO-66 under real sunlight and simulated sunlight. | |
4. Conclusion
A facile and low-cost route was used to construct a heterostructure of UiO-66 coated by MoS2QDs via the ultrasonication method. X-ray diffraction proved that all samples had a single cubic phase of UiO-66 without any additional contaminations from other phases. Morphological measurements (TEM) exhibited the formation of UiO-66@MoS2QDs and the distribution of MoS2QDs on the surface of UiO-66. The photocatalysis performance of UiO-66 was enhanced by increasing the concentration of MoS2QDs up to 15 mL and then decreased by increasing the quantity of MoS2QDs, indicating that the best concentration was 15 mL, which could remove more than 99% of the MB dye within 30 min. The photocatalytic performance of the 15 mL concentration was 97% for real sunlight. The outcomes showed that the proposed photocatalyst retained its performance to remove MB pollutants even after numerous reuse cycles. The current work offers a low-cost, advanced heterostructure, and straightforward photocatalyst manufacturing technique for water purification.
Author contributions
Abdelaziz M. Aboraia: writing – original draft, preparation, and editing. Majd Al-omoush: writing – original draft, preparation and editing. Malak Solayman: validation, software, writing – review & editing. Hatem M. H. Saad: methodology, data curation, writing – original draft, characterization part. Gomaa Khabiri, preparation and editing. M. Saad & Ghalyah M. Alsulaim, methodology, data curation, writing – original draft. Alexander V. Soldatov, review & editing. Yasser A. M. Ismail and H. Gomaa: calculation, review, and writing the manuscript.
Conflicts of interest
The authors have no competing interests to declare that are relevant to the content of this article.
Acknowledgements
The authors extend their appreciation to the Deanship of Scientific Research at King Khalid University for funding this work through a large group Research Project under grant number RGP2/289/44. The authors extend their appreciation to King Khalid University for their continuous support throughout the research.
References
- Y. Shi, J. Ma, Y. Chen, Y. Qian, B. Xu, W. Chu and D. An, Sci. Total Environ., 2022, 804, 150024 CrossRef CAS PubMed.
- Y. Miao, L. Liu, Y. Zhang, Q. Tan and J. Li, J. Hazard. Mater., 2022, 425, 127900 CrossRef CAS PubMed.
- S. Tiwari, S. Kumar and A. K. Ganguli, J. Photochem. Photobiol., A, 2022, 424, 113622 CrossRef CAS.
- H. He, Z. Luo and C. Yu, J. Alloys Compd., 2020, 816, 152652 CrossRef CAS.
- H. He, Z. Luo, Z.-Y. Tang and C. Yu, Appl. Surf. Sci., 2019, 490, 460–468 CrossRef CAS.
- J. Xue, M. Xu, J. Gao, Y. Zong, M. Wang and S. Ma, Colloids Surf., A, 2021, 628, 127288 CrossRef CAS.
- T. Xia, Y. Lin, W. Li and M. Ju, Chin. Chem. Lett., 2021, 32, 2975–2984 CrossRef CAS.
- C. Du, Z. Zhang, G. Yu, H. Wu, H. Chen, L. Zhou, Y. Zhang, Y. Su, S. Tan, L. Yang, J. Song and S. Wang, Chemosphere, 2021, 272, 129501 CrossRef CAS PubMed.
- H. He, L. Zeng, X. Peng, Z. Liu, D. Wang, B. Yang, Z. Li, L. Lei, S. Wang and Y. Hou, Chem. Eng. J., 2023, 451, 138628 CrossRef CAS.
- A. Al Obeidli, H. B. Salah, M. Al Murisi and R. Sabouni, Int. J. Hydrogen Energy, 2022, 47, 2561–2593 CrossRef CAS.
- J. He, J. Wang, Y. Chen, J. Zhang, D. Duan, Y. Wang and Z. Yan, Chem. Commun., 2014, 50, 7063–7066 RSC.
- A. Zada, M. Khan, M. A. Khan, Q. Khan, A. Habibi-Yangjeh, A. Dang and M. Maqbool, Environ. Res., 2021, 195, 110742 CrossRef CAS PubMed.
- A. Kidanemariam, J. Lee and J. Park, Polymers, 2019, 11(12), 2090 CrossRef CAS PubMed.
- T. K. Vo, J. Kim, T. H. Vu, V. C. Nguyen and D. T. Quang, Sep. Purif. Technol., 2022, 283, 120237 CrossRef CAS.
- Z. Man, Y. Meng, X. Lin, X. Dai, L. Wang and D. Liu, Chem. Eng. J., 2022, 431, 133952 CrossRef CAS.
- G. Jajko, J. J. Gutiérrez-Sevillano, A. Sławek, M. Szufla, P. Kozyra, D. Matoga, W. Makowski and S. Calero, Microporous Mesoporous Mater., 2022, 330, 111555 CrossRef CAS.
- V. V. Butova, A. M. Aboraia, M. Solayman, I. S. Yahia, H. Y. Zahran, A. F. Abd El-Rehim, H. Algarni, G. Khabiri and A. V. Soldatov, Microporous Mesoporous Mater., 2021, 325, 111314 CrossRef CAS.
- L. Ni, Y. Zhu, J. Ma and Y. Wang, Water Res., 2021, 188, 116554 CrossRef CAS.
- X. Hao, Z. Jin, H. Yang, G. Lu and Y. Bi, Appl. Catal., B, 2017, 210, 45–56 CrossRef CAS.
- Y. Zhang, W. Li, Z. Hu, X. Jing and L. Yu, Chin. Chem. Lett., 2023, 108938 Search PubMed.
- V. V. Butova, A. P. Budnyk, K. M. Charykov, K. S. Vetlitsyna-Novikova, A. L. Bugaev, A. A. Guda, A. Damin, S. M. Chavan, S. Øien-Ødegaard, K. P. Lillerud, A. V. Soldatov and C. Lamberti, Inorg. Chem., 2019, 58, 1607–1620 CrossRef CAS.
- G. Khabiri, A. M. Aboraia, M. Soliman, A. A. Guda, V. V. Butova, I. S. Yahia and A. V. Soldatov, Ceram. Int., 2020, 46, 19600–19608 CrossRef CAS.
- A. Dasari and V. Guttena, Mater. Today Commun., 2019, 19, 157–169 CrossRef CAS.
- M. Liu, Y. Li, L. Yu, Q. Xu and X. Jiang, Sci. China: Chem., 2018, 61, 294–299 CrossRef CAS.
- S. E. A. Elashery, I. Ibrahim, H. Gomaa, M. M. El-Bouraie, I. A. Moneam, S. S. Fekry and G. G. Mohamed, Magnetochemistry, 2023, 9(2), 56 CrossRef CAS.
- Y. Ding, Y. Zhou, W. Nie and P. Chen, Appl. Surf. Sci., 2015, 357, 1606–1612 CrossRef CAS.
- J. Li, X. Liu, L. Pan, W. Qin, T. Chen and Z. Sun, RSC Adv., 2014, 4, 9647–9651 RSC.
- M. Khnifira, S. El Hamidi, M. Sadiq, S. Şimşek, S. Kaya, N. Barka and M. Abdennouri, Appl. Surf. Sci., 2022, 572, 151381 CrossRef CAS.
- K. O. Kassem, M. A. T. Hussein, M. M. Motawea, H. Gomaa, Z. A. Alrowaili and M. Ezzeldien, J. Cleaner Prod., 2021, 326, 129416 CrossRef CAS.
- K. Ghosh, N. Bar, A. B. Biswas and S. K. Das, Sustainable Chem. Pharm., 2021, 19, 100370 CrossRef CAS.
- F. H. Mustapha, A. A. Jalil, M. Mohamed, S. Triwahyono, N. S. Hassan, N. F. Khusnun, C. N. C. Hitam, A. F. A. Rahman, L. Firmanshah and A. S. Zolkifli, J. Cleaner Prod., 2017, 168, 1150–1162 CrossRef CAS.
- A. M. El Sayed and G. Khabiri, J. Electron. Mater., 2020, 49, 2381–2392 CrossRef CAS.
- S. V. P. Vattikuti, I.-L. Ngo and C. Byon, Solid State Sci., 2016, 61, 121–130 CrossRef CAS.
- A. H. Jawad, R. A. Rashid, M. A. M. Ishak and L. D. Wilson, Desalin. Water Treat., 2016, 57, 25194–25206 CrossRef CAS.
- K. Sharma, R. K. Vyas and A. K. Dalai, J. Chem. Eng. Data, 2017, 62, 3651–3662 CrossRef CAS.
|
This journal is © The Royal Society of Chemistry 2023 |