DOI:
10.1039/D3RA05741K
(Paper)
RSC Adv., 2023,
13, 30443-30452
Oxygen-doped antimonene monolayer as a promising anchoring material for lithium–sulfur batteries: a first-principles study
Received
22nd August 2023
, Accepted 10th October 2023
First published on 16th October 2023
Abstract
To effectively mitigate the dissolution of lithium polysulfides (Li2Sx) in the electrolyte, the search for an effective anchoring material is crucial. In this study, we employed density functional theory (DFT) computations to investigate the adsorption behavior of long-chain Li2Sx species on an O-doped antimonene monolayer. Our results demonstrate that the O-doped antimonene mono-layer exhibits stronger adsorption for long-chain Li2Sx species compared to the pristine antimonene monolayer, resulting in enhanced adsorption energies. This improved adsorption effectively curtails the dissolution of lithium polysulfides and preserves the structural integrity of the Li2Sx species. The charge transfer analysis also revealed the strong chemical interactions between the Li2Sx species and the O-doped antimonene monolayer. These findings suggest that the O-doped anti-monene monolayer holds promise as an effective anchoring material for enhancing the performance of lithium–sulfur batteries.
I. Introduction
To meet the ever-increasing global energy consumption1,2 and accommodate the grow-ing use of electric devices, electric vehicles, and renewable energy sources,3–5 the develop-ment of high-performance rechargeable batteries is desirable. Currently, lithium-ion batter-ies (LIBs) are the optimal rechargeable battery, featuring excellent environmental compat-ibility, high energy density, and long cycle life.6,7 However, LIBs have limited applications in electric vehicles due to safety, durability, and cost considerations.4,6,8 In fact, LIBs are approaching their theoretical energy density limit.9,10 As a potential alternative, lithium–sulfur (Li–S) batteries have gathered great interest due to their higher energy density and theoretical capacity.11,12 Furthermore, the natural abundance, low cost, and non-toxicity of sulfur make the development of Li–S batteries more attractive.13,14 Yet the practical ap-plication of lithium–sulfur batteries is impeded by multiple major obstacles.15 One critical issue is the dissolution of soluble long-chain lithium polysulfides (Li2Sx, x = 4, 6, 8) in the electrolyte during the charge/discharge process. The phenomenon referred to as the shuttle effect results in the loss of active materials, rapid capacity fade, and self-discharge.16–18
Extensive research has been performed to inhibit the shuttle effect, and various strategies have been proposed to suppress it. One effective strategy is to use anchoring materials to bind lithium polysulfides onto their surface through physical/chemical interactions.19 Many anchoring materials have been studied, including various carbon materials such as carbon composites,20 carbon heterostructures21 because of their high conductivity and large surface area. However, these carbon materials exhibited weak interaction with lithium polysulfides, making them ineffective in fully suppressing the shuttle effect.22,23 Many other functional materials have also been studied, including polymers,24,25 metal oxides and sulfides,26 metal organic frameworks,27,28 and other metal compounds.29 Although these functional materi-als have demonstrated strong chemical adsorption strength with lithium polysulfides, their reversible capacity and cycle stability remain unsatisfactory.30,31 In light of this, previous studies have demonstrated that two-dimensional,32–34 with their unique electronic properties,35,36 high surface-volume ratio,37 and multiple adsorption sites38 are promising anchoring materials.
Various two-dimensional materials have been investigated to anchor lithium polysul-fides, including transition metal sulfides (e.g. TiS2,32 VS2,33 WS2 (ref. 34)), other metal com-pounds (e.g. SiC2,39 V2CS2,40 Ti2C41) and monoelemental two-dimensional materials (e.g. borophene,42 phosphorene,43 arsenene,44 and bismuthene44). Many of them exhibit weak interactions with lithium polysulfides. Therefore, further studies have explored several ap-proaches to enhance the adsorption strength of lithium polysulfides onto two-dimensional materials. With vacancies, substitution doping of atoms, and surface functionalizations with atom and molecules, these methods not only improved the adsorption capability of two-dimensional materials but also exposed more adsorption sites.34,45,46 For example, N-doping of graphene,47 transition metal doping of C2N,48 and S-termination of Ti2C Mxene41 have all improved their adsorption capabilities. As such, the use of these strategies are of great interest towards strengthening the performance of anchoring materials.
One promising anchoring material for Li–S batteries is antimonene. Due to its moderate band gap,49 high carrier mobility,50,51 and high structural stability at ambient temperatures,46,52 antimonene is promising for application in energy storage. While pre-vious research has demonstrated its effectiveness as an electrode material for LIBs53 and sodium-ion batteries,54 recent studies have explored its potential for Li–S batteries.44,49 However, pristine antimonene exhibits only weak to moderate adsorption capabilities of anchoring lithium polysulfides in Li–S batteries.44 To overcome this limitation, researchers have turned to doping strategies, with vanadium, tin, and selenium dopants showing promis-ing results through atom substitution.49 Nevertheless, concerns remain about the strength of adsorption and potential detachment of the adsorbed polysulfides from the doped an-choring material.55 To address this challenge, oxygen doping has shown promise. Studies involving oxygen doping, such as carbon nitride tubes, revealed improved adsorption of lithium polysulfides through chemical interactions upon substantial oxygen doping.56–58 Furthermore, doping can decrease the band gap of the monolayer and enhance the intrinsic conductivity, facilitating better lithium diffusion.59 Thus, we aim to study the adsorption of lithium polysulfides on oxygen-doped antimonene monolayer, potentially improving the performance and stability of Li–S batteries.
We performed first-principle calculations based on Density Functional Theory (DFT) to study the structural and electronic properties of lithium polysulfides adsorbed on pure and doped antimonene. The adsorption energies of the lithium polysulfides adsorbed on pure and oxygen-doped antimonene were calculated to study the suppression of the shuttle effect. In addition to adsorption energies, the band structure, and charge transfer were calculated.
II. Methods
A. Computational details
We performed first-principle calculations based on Density Functional Theory (DFT) within the Perdew–Burke–Ernzerhof (PBE) Generalized Gradient Approximation (GGA) implemented in the ABINIT60 code. We used the Projected Augmented Wave (PAW) method61 with projectors generated with the ATOM code.62 The cut-off radii are 1.0, 1.5, 1.4, 2.4, 1.6, and 1.9 Bohr, and the electrons configurations are 1s1, [He] 2s2 2p2, [He] 2s2 2p4, [Kr] 5s2 5p3 4d10, 1s2 2s1, and [Ne] 3s2 3p4 for H, C, O, Sb, Li, and S, respectively.
Convergence was carried out to determine the appropriate converged values for the kinetic energy cutoff, Monkhorst–Pack k point grids, and vacuum. The values were considered converged when the difference in total energy was less than 1.0 × 10−4 Hartree twice consecutively.63 During the convergence calculations, the self-consistent field (SCF) total energy calculations were considered complete when the total energy difference was less than 1.0 × 10−10 Hartree for the second time.63
The Broyden–Fletcher–Goldfarb–Shanno64 (BFGS) method was used for the relax-ation of the lattice parameters and atomic structure. During relaxation calculations, SCF iterations were completed when the total difference in forces was less than 2.0 × 10−5 Hartree Bohr−1 twice consecutively. The relaxation calculations were considered complete when all of the forces were less than 6.0 × 10−4 Hartree Bohr−1 (around 0.03 eV Å−1).65
B. Atomic structure
Monolayer antimonene exists in several allotropes, differentiated by their prefixes, α, β, γ, and others.46 It has been predicted by phonon dispersion spectra, mechanically, and thermally that the α- and β-phases are stable and semiconducting and β-phase is the most stable allotrope.66
Previous studies demonstrated β-phase antimonene has nonplanar structure and hexagonal arrangement.66 In this study, a 4 × 4 × 1 supercell of β-phase antimonene with 32 Sb atoms was used for calculations. We will be substitutionally doping the monolayer with O to enhance its effects on the adsorption of long-chain lithium polysulfides Li2S4, Li2S6, and Li2S8. The defect formation energy (Eform)67 is defined by
|
Eform = EOSbML − ESbML − EO + ESb
| (1) |
where
EOSbML is the total energy of the O-doped antimonene monolayer,
ESbML is the total energy of the pristine antimonene monolayer,
EO is the chemical potential of the O atom, and
ESb is the chemical potential of the Sb atom.
C. Li2Sx adsorption
To demonstrate the adsorption capabilities of the antimonene monolayer, the ad-sorption energies of Li2Sx (x = 1, 2, 4, 6, 8) with the typical electrolytes dimethyl ether (DME)/1,3-dioxolane (DOL) were calculated with the following equation |
Ebind = ELiPS+electro − ELiPS − Eelectro
| (2) |
where ELiPS+electro is the total energy of the lithium polysulfide and electrolyte DME/DOL complex, ELiPS is the total energy of the Li2Sx (x = 1, 2, 4, 6, 8), and Eelectro is the total energy of the electrolyte DME/DOL.
Alongside this, the adsorption energies Eads of long-chain lithium polysulfides Li2Sx (x = 4, 6, 8) on the pristine and O-doped antimonene monolayers were calculated by the following equation
|
Eads = ELiPS+ML − EML − ELiPS
| (3) |
where
ELiPS+ML is the total energy of the lithium polysulfide and pristine or O-doped antimonene monolayer complex,
EML is the total energy of the pristine or O-doped anti-monene monolayer, and
ELiPS is the total energy of the Li
2S
x (
x = 4, 6, 8), respectively.
D. Electronic structure
The band structure of the antimonene system was calculated before and after the adsorption of Li2Sx (x = 4, 6, 8) polysulfides, and it was plotted using the high symmetry k-points Γ (0, 0, 0) M (1/2, 0, 0) K (2/3, 1/3, 0) and Γ (1, 1, 1).
The interaction between adsorbed Li2Sx (x = 4, 6, 8) and O-doped antimonene was further confirmed by calculating the charge transfer. The charge transfer Δρ(r) is defined by
|
Δρ(r) = ρLiPS/OSbML(r) − ρOSbML(r) − ρLiPS(r)
| (4) |
where
ρLiPS/OSbML(
r) is the charge density of the lithium polysulfide-adsorbed antimonene monolayer system,
ρOSbMl(
r) is the charge density of the O-doped antimonene monolayer, and
ρLiPS(
r) is the charge density of the lithium polysulfide.
III. Results and discussion
We carried out first-principle calculations to investigate the adsorption of lithium poly-sulfides species on commonly used electrolytes DME and DOL molecules, and both pristine and oxygen-doped antimonene monolayers. We fully relaxed the atomic structures and cal-culated the adsorption energy, band structure, as well as charge transfer.
A. Adsorption of Li2Sx species on typical electrolytes
1. Atomic structural properties of Li2Sx species. To mitigate the shuttle effect within Li–S batteries, understanding the formation of lithium polysulfides is critical. During the discharge process, Li is oxidized and travels to-ward the cathode. The reaction between the Li ions and different sulfur concentrations form lithium polysulfides: Li2Sx (x = 1, 2, 4, 6, 8).68–70 The molecular models of Li2Sx (x = 1, 2, 4, 6, 8) were taken from previous research.71 First, we examined the structural properties of the lithium polysulfides. The most stable configurations after geometric op-timization/relaxation are shown in Fig. 1. The insoluble species Li2S and Li2S2 have a Li–S bond length of 2.22 and 2.34 Å. The soluble Li2S4, Li2S6, and Li2S8 with shortest bond lengths of Li–S and S–S of about 2.38, and 2.08 Å, respectively. In addition, it is found that all of these species are three-dimensional rather than linear, which is consistent with previ-ous theoretical studies.70 As shown in Table 1, the Li–Li distance decreases, while the Li–S bond distance increases when the number of S atoms increases. Not only this, the Li–S–Li bond angle decreases as the number of S atoms increased, meaning the molecules thickness decreases with the addition of S. Overall, the structural data of lithium polysulfides, as shown in Table 1, are in good agreement with previous theoretical calculations,72–74 sug-gesting our methods are reliable. Long-chain lithium polysulfides Li2S4, Li2S6, and Li2S8, were selected due to their high solubility in the organic electrolytes leading to large capacity fading during the cycling or the shuttle effect.75,76 In comparison, the short-chain lithium polysulfides Li2S and Li2S2 are insoluble in the typical electrolytes DOL and DME, which is why we did not complete further research.75,76
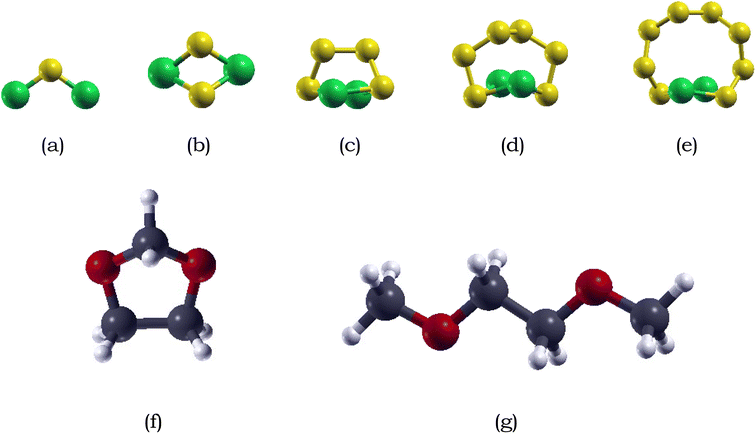 |
| Fig. 1 Optimized atomic structures of Li2Sx (x = 1, 2, 4, 6, 8) and electrolytes DME/DOL: (a) Li2S, (b) Li2S2, (c) Li2S4, (d) Li2S6, (e) Li2S8 (f) DOL, and (g) DME. H, O, C, Li and S atoms are represented by white, red, blue, green, and yellow, respectively. | |
Table 1 The optimized structural parameters of Li2Sx (x = 1, 2, 4, 6, 8): bond length of S–S (dS–S), bond length of Li–S (dLi–S), bond angle of Li–S–Li (θLi–S–Li), and bond distance of Li–Li (dLi–Li) in Li2Sx (x = 1, 2, 4, 6, 8)
Species |
Li2S |
Li2S2 |
Li2S4 |
Li2S6 |
Li2S8 |
Ref. 71. Ref. 72. Ref. 73. |
dS–S (Å) |
— |
2.19 |
2.09 |
2.04 |
2.08 |
dS–S (Å) |
— |
2.19a |
2.19a |
2.08a |
2.07a |
dS–S (Å) |
— |
— |
2.141b |
2.261b |
2.087b |
dLi–S (Å) |
2.09 |
2.22 |
2.34 |
2.38 |
2.38 |
dLi–S (Å) |
2.09a |
2.22a |
2.48a |
2.55a |
2.42a |
dLi–S (Å) |
2.073b |
2.227b |
2.377b |
2.407b |
2.412b |
dLi–S (Å) |
2.09c |
2.23c |
2.36/2.40c |
2.35/2.41c |
2.38/2.39c |
θLi–S–Li (deg) |
109.3 |
95.32 |
77.38 |
68.65 |
66.25 |
θLi–S–Li (deg) |
131.8c |
96.8c |
73.5c |
69.1c |
66.3c |
dLi–Li (Å) |
3.41 |
3.28 |
2.82 |
2.67 |
2.59 |
2. Atomic structural properties of typical electrolytes DME and DOL. The electrolyte is also critical to the electrochemical performance of Li–S batteries, as it governs the movement of lithium ions between the electrodes during charging and discharg-ing. Additionally, the electrolyte plays a crucial role in forming a stable solid electrolyte interphase (SEI) with the electrodes, which significantly impacts battery performance.77,78 Extensive experimentation has led to the standard electrolyte formulation: a 1
:
1 mixture of dimethyl ether (DME) and 1,3-dioxolane (DOL). This combination exhibits superior reactivity with polysulfides, ensuring enhanced stability and improved electrochemical performance in Li–S batteries.68,79,80The optimized structure of DME and DOL are shown in Fig. 1. DME consists of a central ethane backbone with a methyl (–CH3) group attached to each of the carbon atoms. The calculated C–O–C bond angle after relaxation was measured to be 112.13°, indicating a bent molecular geometry, which is in good agreement with the experimentally calculated angle of approximately 111.43°.81 DOL adopts a puckered five-member ring, where the ring is not perfectly planar but instead exhibits slight deviation from planarity. The bond lengths between the carbon and oxygen atoms in DOL are relatively equal about 2.7 Å.
3. Adsorption of Li2Sx species on typical electrolytes. To investigate the adsorption of lithium polysulfides on commonly used electrolytes (DME/DOL), we calculated the structural properties of lithium polysulfides and DME/DOL, which is shown in Fig. 2. For DOL, the shortest intermolecular distance between Li and O is 2.04, 2.02, 2.15, 1.92, and 1.94 Å for Li2S, Li2S2, Li2S4, Li2S6, and Li2S8, respectively. The shortest intermolecular distance between Li2S, Li2S2, Li2S4, Li2S6, and Li2S8 and DME is 1.99 Å, 1.92 Å, 2.01 Å, 2.01 Å, and 2.01 Å respectively. To compare, the Li atoms are closer to the O atoms than the S atoms when interacting with both DOL and DME. In addition, the shortest distances are approximately the sum of the covalaent radii between Li atom and O atom. Therefore, the Li2Sx species and typical electrolytes tend to form Li–O bonds, which demonstrates that the interaction between the lithium polysulfide species and electrolytes is partially due to chemical interaction.
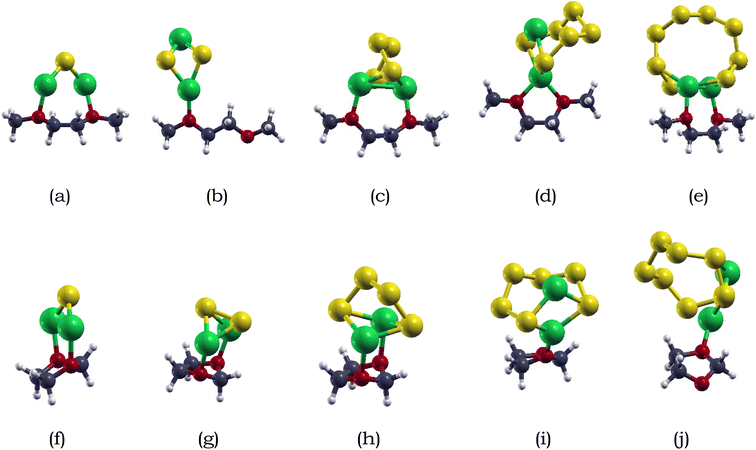 |
| Fig. 2 Optimized atomic structures of Li2Sx (x = 1, 2, 4, 6, 8) adsorbed with electrolytes DME/DOL: (a) Li2S + DME, (b) Li2S2 + DME, (c) Li2S4 + DME, (d) Li2S6 + DME, (e) Li2S8 + DME, (f) Li2S + DOL, (g) Li2S2 + DOL, (h) Li2S4 + DOL, (i) Li2S6 + DOL, (j) Li2S8 + DOL. H, O, C, Li, and S atoms are represented by white, red, blue, green and yellow. | |
The adsorption energies of lithium polysulfides on DME and DOL are summarized in Table 2. The adsorption energies of Li2S, Li2S2, Li2S4, Li2S6, and Li2S8 on DOL are −0.83, −0.94, −0.78, −0.69, and −1.05 eV respectively. For DME, the adsoprtion energies of Li2S, Li2S2, Li2S4, Li2S6, and Li2S8 are −0.94, −0.73, −0.62, −1.05, and −0.77 eV, respectively. The adsorption energies between the lithium polysulfides and electrolytes range of −0.69 to −1.05 eV for DOL, and −0.62 to −1.05 eV for DME, showing that the adsorption of lithium polysulfides on DME and DOL do not significantly differ. Therefore, the preferable range of adsorption energies would be from around −1.00 eV to −2.00 eV, as extreme adsorption can hinder the detachment of adsorbed lithium polysulfide from the anchoring material.82
Table 2 The binding energy Ebind (eV) of Li2Sx (x = 1, 2, 4, 6, 8) molecules with DME and DOL molecules
|
Li2S |
Li2S2 |
Li2S4 |
Li2S6 |
Li2S8 |
Ebind-DME (eV) |
−0.94 |
−0.73 |
−0.62 |
−1.05 |
−0.77 |
Ebind-DOL (eV) |
−0.83 |
−0.94 |
−0.78 |
−0.69 |
−1.05 |
B. Adsorption of Li2Sx species on pristine antimonene monolayer
1. Atomic structural and electronic properties of pristine antimonene monolayer. Fig. 3 shows the 4 × 4 × 1 antimonene monolayer, where two Sb atoms make up a unit cell, which are stacked in a zigzag monolayer. Each Sb atom binds with three adjacent atoms in a different plane. Due to the same bond angle of 91.42° between Sb atoms, the monolayer has hexagonal arrangement. The fully relaxed lattice parameters of the pristine antimonene monolayer is a = b = 4.12 Å and the bond length between Sb–Sb is found to be 2.89 Å, as presented in Table 5. Overall, our calculated values of the lattice parameters and bond lengths are in good agreement with previously reported works.49
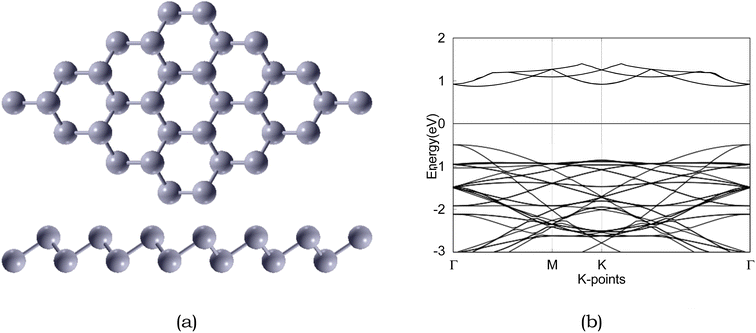 |
| Fig. 3 Atomic structure and band structure of 4 × 4 × 1 pristine. The Fermi level is set to 0. Sb is represented by silver, respectively. | |
Due to the insulating nature of sulfur, an ideal anchoring material for the Li–S battery should possess excellent electronic conductivity, which will greatly affect the performance and operability of the battery. Therefore, we have computed the electronic band structure to understand the electronic properties of antimonene. The monolayer exhibits a band gap of 1.52 eV, as presented in Table 5. The monolayer is an indirect band gap semiconductor between the Γ and M points, as presented in Fig. 3.
2. Adsorption of Li2Sx species on pristine antimonene monolayer. An optimal anchoring material for Li–S batteries should be able to immobilize lithium polysulfides effectively with strong chemical adsorption. However, extreme adsorption is also undesirable as large adsorption energies, greater than 3.00 eV, can impede the charge/discharge process and cause deformation of polysulfides.71 Following previous research, we adsorbed the long-chain polysulfides Li2Sx (x = 4, 6, 8) onto pristine antimonene monolayer.49 The optimized configurations of Li2Sx (x = 4, 6, 8) on the antimonene mono-layer are shown in Fig. 4a–c, respectively.
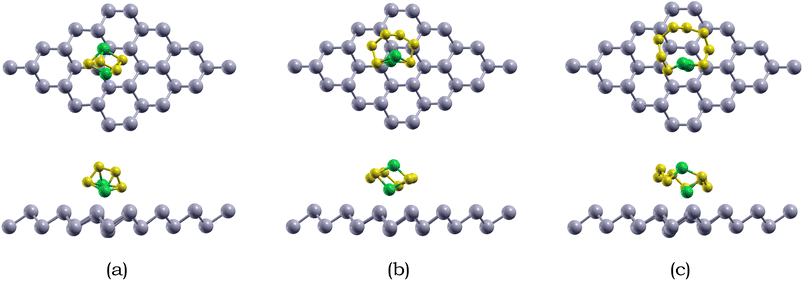 |
| Fig. 4 Top and side views of the optimized atomic structures Li2Sx (x = 4, 6, 8) adsorbed on 4 × 4 × 1 pristine antimonene monolayer: (a) Li2S4, (b) Li2S6, and (c) Li2S8. Sb, Li, and S are represented by silver, green, and yellow, respectively. | |
For Li2S4 adsorption, the Li atoms face downwards towards the monolayer, while for Li2S6 and Li2S8 adsorption, the S chain is parallel to the monolayer surface. The shortest intermolecular distance between Li2S6, Li2S6, and Li2S8 and the antimonene monolayer is 2.93, 2.98, and 3.16 Å, respectively. Specifically, the Li atoms are closer than the S atoms when interacting with the antimonene monolayer, and the two Li atoms prefer to adsorb around the hexagonal edges of antimonene through Li–Sb interactions.
We also calculated the variances of the corresponding structural parameters for both Li2Sx and pristine antimonene as summarized in Table 3. The average change of the Li–S bond in Li2S4, Li2S6, and Li2S8 is 0.04, 0.02, and 0.07 Å. Not only this, for the antimonene monolayer, the nearby Sb–Sb bonds increased only around 0.01 Å. Therefore, little structural deformation is observed for both the adsorbed long-chain lithium polysulfides and the antimonene monolayer, which is preferable as severe deformation away from the stable configuration is unfavorable.83
Table 3 The shortest distance between Li2Sx (x = 4, 6, 8) and pristine antimonene monolayer dSb–Li, the change of the distance between Li and S atoms in Li2Sx (x = 4, 6, 8) ΔdLi–S, and the change in the bond length between Sb and Sb atoms in pristine antimonene monolayer ΔdSb–Sb
Species |
dSb–Li (Å) |
ΔdLi–S (Å) |
ΔdSb–Sb (Å) |
Li2S4 |
2.93 |
0.04 |
0.01 |
Li2S6 |
2.98 |
0.02 |
0.01 |
Li2S8 |
3.16 |
0.07 |
0.01 |
The adsorption energies of the Li2Sx (x = 4, 6, 8) species on antimonene are listed in Table 4. The adsorption energies of Li2S4, Li2S6, and Li2S8 on the antiomonene monolayer are −0.90, −0.82, and −0.70 eV, respectively. The overall adsorption energies of antimonene to the Li2Sx (x = 4, 6, 8) species range from −0.70 to −0.90 eV, which are similar to previous results.49
Table 4 Adsorption energy Eads (eV) of Li2Sx (x = 4, 6, 8) on both pristine antimonene (SbML) and O-doped antimonene monolayer (OSbML) substrates
LiPS |
Li2S4 |
Li2S6 |
Li2S8 |
Ref. 49. |
Eads-SbML (eV) |
−0.90 |
−0.82 |
−0.70 |
Eads-SbML (eV) |
−1.01a |
−1.164a |
−1.40a |
Eads-OSbML (eV) |
−1.24 |
−1.21 |
−1.12 |
C. Adsorption of Li2Sx species on O-doped antimonene monolayer
1. Atomic structural and electronic properties of O-doped antimonene monolayer. To further improve the performance of antimonene for applications in Li–S bat-tery, a doping modification was applied. Among the various dopants used in previous studies,41,47–49 oxygen doping has been far less studied and applied for Li–S batteries. However, it has demonstrated success with strong chemical interactions with Li–O bonds forming in its applications in carbon–nitride tubes,57 carbon nanofiber interlayers,58 and other carbon materials.56 Therefore, we doped antimonene with oxygen and evaluated its performance. After geometric optimization, we obtained a stable oxygen-doped antimonene monolayer. As shown in Fig. 5, the oxygen atom coordinates with the 3 adjacent Sb atoms in the corresponding configuration. It can be observed that the O atom slightly deformed the six-member ring near the doping site and shrank into the antimonene monolayer. The O atom shrank into the monolayer due to the smaller atomic radii of O atom than Sb atoms. The calculated O–Sb bond length after relaxation was 2.23. The bond angle of the Sb–O–Sb was found to be 111.09°. The lattice constants was measured to be 4.07 Å decreased from pristine antimonene's lattice constant of 4.12 Å, as presented in Table 5. To evaluate the thermodynamic stability of the oxygen-doped system, the formation energy was calculated using eqn (1). The defect formation energy was calculated to be 1.09 eV, as presented in Table 5. The low positive formation energy demonstrates the applicability of oxygen doping within antimonene, as the small magnitude of the formation energy demonstrates the material should be mechanically stable in a natural environment. Therefore, we further investigated the structural and electronic properties of the doped system. As shown in Fig. 5, the doped monolayer exhibits a band gap of 1.37 eV. The monolayer is an indirect band gap semiconductor between the Γ and M points, as presented in Fig. 5. The re-duced bandgap will increase the electrical conductivity and improve the performance of Li–S batteries.
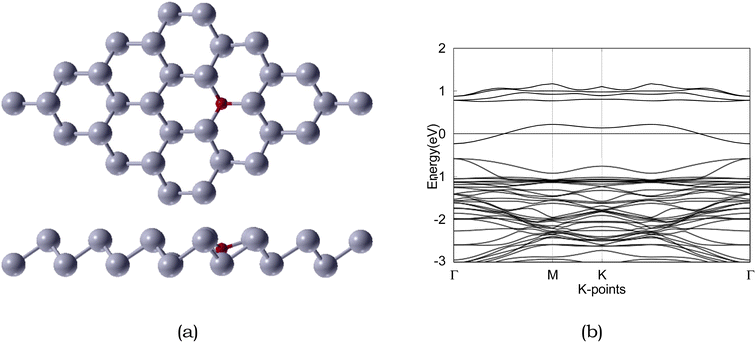 |
| Fig. 5 Atomic structure and band structure of 4 × 4 × 1 O-doped antimonene monolayer. The Fermi level is set to 0. Sb and O are represented by silver and red, respectively. | |
Table 5 Optimized atomic structure of 4 × 4 × 1 pristine antimonene monolayer (SbML) and O-doped antimonene monolayer (OSbML): lattice constant a (Å), bond length of Sb–Sb in pristine antimonene dSb–Sb (Å), bond length of O–Sb in O-doped antimonene dO–Sb (Å), bond angle of Sb–Sb–Sb in pristine antimonene θSb–Sb–Sb (deg), bond angle of Sb–O–Sb in O-doped antimonene θSb–Sb–Sb (deg), defect formation energy Eform (eV), and bandgap Eg (eV)
Configuration |
a (Å) |
dSb–Sb (Å) |
dO–Sb (Å) |
θSb–Sb–Sb (deg) |
θSb–O–Sb (deg) |
Eform (eV) |
Eg (eV) |
Ref. 49. |
SbML |
4.12 |
2.89 |
— |
91.42 |
— |
0.00 |
1.52 |
SbML |
4.07a |
2.84a |
— |
91.47a |
— |
0.00a |
1.37a |
OSbML |
4.07 |
— |
2.23 |
— |
111.09 |
1.07 |
0.00 |
2. Adsorption of Li2Sx species on O-doped antimonene monolayer. We first examine the adsorption strength between the Li2Sx species and the O-doped antimonene monolayer. Various initial adsorption configurations for Li2Sx (x = 4, 6, 8) were considered. The optimized configurations of Li2Sx (x = 4, 6, 8) are shown in Fig. 6a–c, respectively. For Li2S4 adsorption, the Li atoms slant downwards towards the monolayer, while for Li2S6 and Li2S8 adsorption, the S chain is parallel to the monolayer surface. The shortest intermolecular distance between Li2S4, Li2S6, and Li2S8 is 1.95, 1.87, and 1.88 Å, respectively. Specifically, the Li atoms are closer than the S atoms when interacting with the doped monolayer, and the Li atoms prefer to adsorb around the O atom through Li–O interactions. In addition, for all the long-chain lithium polysulfides the shortest distances are approximately the sum of the covalent radii between the Li atom and Sb atom. Therefore, the Li2Sx species and the monolayers tend to form Li–O bonds, which confirms the anchoring effect in these adsorption systems has a partial contribution from the chemical interaction. Comparatively, the distances for O-doped antimonene are considerably smaller than that of the pristine antimonene reflecting the stronger interactions between the adsorbate and substrate.
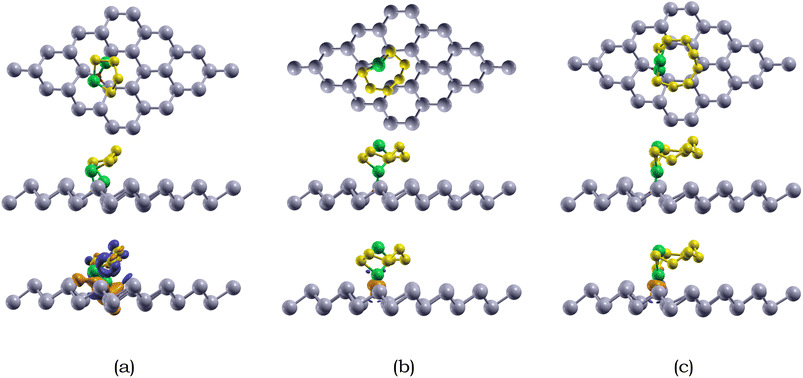 |
| Fig. 6 Top view (1st row) and side view (2nd row) of the optimized atomic structure and charge transfer (3rd row) of Li2Sx (x = 4, 6, 8) species on the O-doped antimonene monolayer (OSbML) (a) Li2S4/OSbML (b) Li2S6/OSbML, and (c) Li2S8/OSbML. The orange and dark blue bubbles represent charge accumulation and depletion, respectively. The isosurface is 0.002 e per Å3. Sb, O, Li, and S are represented by silver, red, green, and yellow, respectively. | |
We also calculated the variances of the corresponding structural parameters for both Li2Sx (x = 4, 6, 8) and the O-doped antimonene monolayer, as summarized in Table 6. The average change of the Li–S bond in Li2S4, Li2S6, and Li2S8 is 0.44, 0.04, and 0.07 Å. Not only this, for the antimonene monolayer, the nearby O–Sb bonds increased around 0.57, 0.43, and 0.47 Å for the adsorption of Li2S4, Li2S6, and Li2S8. The considerable change in the substrate is observed due to the attraction between the Li and O atoms, resulting in the O atom moving away from one of the adjacent Sb atoms to bond with the Li atoms. The variances of the structural parameters of both adsorbates and substrates are considerably larger, indicating that the Li2Sx species experienced some deformation, but are nearly intact.
Table 6 The shortest distance between Li2Sx (x = 4, 6, 8) and O-doped antimonene monolayer dO–Li, the change of the distance between Li and S atoms in Li2Sx (x = 4, 6, 8) ΔdLi–S, and the change in the bond length between Sb and O atoms in O-doped antimonene monolayer ΔdSb–O
Species |
dO–Li (Å) |
ΔdLi–S (Å) |
ΔdSb–O (Å) |
Li2S4 |
1.95 |
0.44 |
0.57 |
Li2S6 |
1.87 |
0.04 |
0.43 |
Li2S8 |
1.88 |
0.07 |
0.47 |
The adsorption energies of the Li2Sx (x = 4, 6, 8) species on O-doped antimonene are listed in Table 4. The adsorption energies of Li2S4, Li2S6, and Li2S8 on the doped monolayer is −1.24, −1.21, and −1.12 eV, respectively. The overall adsorption energies Li2Sx (x = 4, 6, 8) species adsorbed on O-doped antimonene range from −1.12 to −1.24 eV, stronger than those of pristine antimonene. The higher chemical reactivity between the Li2Sx species and O-doped antimonene than pristine antimonene is due to the electronegativity difference between Li and O.
Moreover, we compared the energy gain for Li2Sx species to form large Li–S interconnected clusters (or networks) and the adsorption energies of the Li2Sx (x = 4, 6, 8) on O-doped antimonene. The energy gain to create interconnected Li2Sx clusters is less than around 0.40 eV for Li2Sx (x = 4, 6, 8),69 which is smaller than the Li2Sx and O-doped anti-monene interactions (−1.24, −1.21, −1.12 eV, respectively). Consequently, the three long-chain lithium polysulfides generally prefer anchoring on O-doped antiomonene than nucleation into larger Li2Sx clusters. In addition, we compared the binding energy for Li2Sx species with DME and DOL and the adsorption energies of Li2Sx (x = 4, 6, 8) on O-doped antimonene. The binding energies of the Li2Sx species with the electrolytes are smaller than those with the O-doped antimonene monolayer. Therefore, it can be seen that the Li2Sx species would prefer to anchor on the O-doped antimonene monolayer and not dissolve in the electrolyte.
Overall, the adsorption energies of the soluble Li2Sx species are moderate (−1.00 to −2.00 eV), the adsorbed Li2Sx species and the O-doped antimonene are nearly intact. Therefore, we expect that O-doped antimonene is suitable as an anchoring material for Li–S batteries.
3. Charge transfer. We also performed charge transfer calculations to investigate the adsorption properties of Li2Sx (x = 4, 6, 8) species on the O-doped antimonene monolayer, following eqn (4). Notably, significant regions of electron accumulation are located between the Li atom of the Li2Sx species and the O dopant, as depicted in the 2nd row of Fig. 6. There was also minimal charge redistribution in Li2S6 and Li2S8 compared to Li2S4. The enhanced adsorption energy can be explained by the large electronegativity difference between lithium and oxygen. Because of the significant difference in electronegativity between lithium (3.44) and oxygen (0.98), the interaction is very analogous to the “Li bond” explained in the Lewis acid–base theory.84,85 The O-doped antimonene with an extra pair of electrons is expected as an electron-rich donor that naturally acts as Lewis base sites to strongly absorb Lewis acidic Li ions through acid–base interactions. The significant electron density visible around the lone pairs of the O atoms strengthens the fact that these extra electrons act as electron-rich donors that interact with the strong Li-ion Lewis acid to form a coordinate covalent bond,86 as shown in the 2nd and 3rd rows of Fig. 6. Overall, our findings confirm the strong chemical interactions between the Li2Sx species and the O-doped antimonene monolayer, further supporting its potential as an effective anchoring material for Li–S batteries.
IV. Conclusion
By using first-principles calculations based on DFT, the adsorption behavior of Li2Sx (x = 4, 6, 8) species on the pristine and O-doped antimonene monolayers was investigated. The Li2Sx species were weakly adsorbed on the pristine antimonene monolayer while mod-erately adsorbed on the O-doped antimonene monolayer. Therefore, the Li2Sx species are adsorbed on the O-doped antimonene monolayer and the dissolution of the Li2Sx species into the electrolyte is prevented from an energetic point of view. Furthermore, the charge transfer from the Li2Sx species to the O-doped antimonene monolayer revealed strong chem–ical interactions between the Li2Sx species and O-doped antimonene monolayer. Therefore, the O-doped antimonene monolayer is a promising anchoring material for high-performance Li–S batteries.
Conflicts of interest
There are no conflicts to declare.
Acknowledgements
We would like to thank Dr Geifei Qian for his technical support throughout our research.
References
- L. Pérez-Lombard, J. Ortiz and C. Pout, Energy Buildings, 2008, 40, 394 CrossRef.
- K. Karpov, Studies on Russian Economic Development, 2019, 30, 38 CrossRef.
- A. Bloom, U. Helman, H. Holttinen, K. Summers, J. Bakke, G. Brinkman and A. Lopez, IEEE Power Energy Mag., 2017, 15, 22 Search PubMed.
- M. M. Thackeray, C. Wolverton and E. D. Isaacs, Energy Environ. Sci., 2012, 5, 7854 RSC.
- Y. Wang, B. Liu, Q. Li, S. Cartmell, S. Ferrara, Z. D. Deng and J. Xiao, J. Power Sources, 2015, 286, 330 CrossRef CAS.
- B. Scrosati and J. Garche, J. Power Sources, 2010, 195, 2419 CrossRef CAS.
- M.-Y. Yan, G. Li, J. Zhang, Y.-F. Tian, Y.-X. Yin, C.-J. Zhang, K.-C. Jiang, Q. Xu, H.-L. Li and Y.-G. Guo, ACS Appl. Mater. Interfaces, 2020, 12, 27202 CrossRef CAS PubMed.
- L. Lu, X. Han, J. Li, J. Hua and M. Ouyang, J. Power Sources, 2013, 226, 272 CrossRef CAS.
- T. C. Wanger, Conserv. Lett., 2011, 4, 202 CrossRef.
- K. Abraham, J. Phys. Chem. Lett., 2015, 6, 830 CrossRef CAS PubMed.
- K. Divya and J. Østergaard, Electr. Power Syst. Res., 2009, 79, 511 CrossRef.
- J. Cho, S. Jeong and Y. Kim, Prog. Energy Combust. Sci., 2015, 48, 84 CrossRef.
- A. Manthiram, Y. Fu and Y.-S. Su, Acc. Chem. Res., 2013, 46, 1125 CrossRef CAS PubMed.
- Y. Yang, G. Zheng and Y. Cui, Chem. Soc. Rev., 2013, 42, 3018 RSC.
- J. Sun, T. Wang, Y. Gao, Z. Pan, R. Hu and J. Wang, InfoMat, 2022, 4, e12359 CrossRef CAS.
- D. Liu, C. Zhang, G. Zhou, W. Lv, G. Ling, L. Zhi and Q.-H. Yang, Adv. Sci., 2018, 5, 1700270 CrossRef PubMed.
- J. Yan, X. Liu and B. Li, Advanced Science, 2016, 3, 1600101 CrossRef PubMed.
- Y. V. Mikhaylik and J. R. Akridge, J. Electrochem. Soc., 2004, 151, A1969 CrossRef CAS.
- W. Ren, W. Ma, S. Zhang and B. Tang, Energy Storage Mater., 2019, 23, 707 CrossRef.
- G. Li, J. Sun, W. Hou, S. Jiang, Y. Huang and J. Geng, Nat. Commun., 2016, 7, 1 CrossRef.
- C. Zhang, Q. He, W. Chu and Y. Zhao, Appl. Surf. Sci., 2020, 534, 147575 CrossRef CAS.
- L. Chen, H. Zhou, C. Fu, Z. Chen, C. Xu and Y. Kuang, Int. J. Hydrogen Energy, 2016, 41, 21850 CrossRef CAS.
- Q. Pang, J. Tang, H. Huang, X. Liang, C. Hart, K. C. Tam and L. F. Nazar, Adv. Mater., 2015, 27, 6021 CrossRef CAS PubMed.
- Y. Fu, Y.-S. Su and A. Manthiram, J. Electrochem. Soc., 2012, 159, A1420 CrossRef CAS.
- X. Zhao, C. Wang, Z. Li, X. Hu, A. A. Razzaq and Z. Deng, J. Mater. Chem. A, 2021, 9, 19282 RSC.
- X. Liu, J.-Q. Huang, Q. Zhang and L. Mai, Adv. Mater., 2017, 29, 1601759 CrossRef PubMed.
- J. Zheng, J. Tian, D. Wu, M. Gu, W. Xu, C. Wang, F. Gao, M. H. Engelhard, J.-G. Zhang and J. Liu, et al., Nano Lett., 2014, 14, 2345 CrossRef CAS PubMed.
- Y. Hou, H. Mao and L. Xu, Nano Res., 2017, 10, 344 CrossRef CAS.
- J. Xu, T. Lawson, H. Fan, D. Su and G. Wang, Adv. Energy Mater., 2018, 8, 1702607 CrossRef.
- C. Li, Z. Xi, D. Guo, X. Chen and L. Yin, Small, 2018, 14, 1701986 CrossRef PubMed.
- Y. Zhu, S. Wang, Z. Miao, Y. Liu and S.-L. Chou, Small, 2018, 14, 1801987 CrossRef PubMed.
- W. Zhao, L.-C. Xu, R. Li, Y. Guo, Z. Yang, R. Liu and X. Li, Mater. Today Commun., 2022, 30, 103196 CrossRef CAS.
- Y. Wang, Z. Ma, N. Song, T. Zhang, Q. Zhang, D. Yang and F. Wang, Chem. Phys. Lett., 2020, 741, 137121 CrossRef CAS.
- R. Jayan and M. M. Islam, J. Phys. Chem. C, 2020, 124, 27323 CrossRef CAS.
- S. Z. Butler, S. M. Hollen, L. Cao, Y. Cui, J. A. Gupta, H. R. Gutíerrez, T. F. Heinz, S. S. Hong, J. Huang and A. F. Ismach, et al., ACS Nano, 2013, 7, 2898 CrossRef CAS PubMed.
- Q. H. Wang, K. Kalantar-Zadeh, A. Kis, J. N. Coleman and M. S. Strano, Nat. Nanotechnol., 2012, 7, 699 CrossRef CAS PubMed.
- D. Chimene, D. L. Alge and A. K. Gaharwar, Adv. Mater., 2015, 27, 7261 CrossRef CAS PubMed.
- H. Xu, Z. Kong, J. Siegenthaler, B. Zheng, Y. Tong, J. Li, T. Schuelke, Q. H. Fan, K. Wang and H. Xu, et al., EcoMat, 2023, 5, e12286 CrossRef CAS.
- Y. Zhao, J. Zhao and Q. Cai, Appl. Surf. Sci., 2018, 440, 889 CrossRef CAS.
- Y. Wang, J. Shen, L.-C. Xu, Z. Yang, R. Li, R. Liu and X. Li, Phys. Chem. Chem. Phys., 2019, 21, 18559 RSC.
- X. Liu, X. Shao, F. Li and M. Zhao, Appl. Surf. Sci., 2018, 455, 522 CrossRef CAS.
- L. Zhang, P. Liang, H.-b. Shu, X.-l. Man, F. Li, J. Huang, Q.-m. Dong and D.-l. Chao, J. Phys. Chem. C, 2017, 121, 15549 CrossRef CAS.
- H. H. Haseeb, Y. Li, S. Ayub, Q. Fang, L. Yu, K. Xu and F. Ma, J. Phys. Chem. C, 2020, 124, 2739 CrossRef CAS.
- X. Mao, L. Zhu and A. Fu, Int. J. Quantum Chem., 2021, 121, e26661 CrossRef CAS.
- N. Yamsang, J. Sittiwong, P. Srifa, B. Boekfa, M. Sawangphruk, T. Maihom and J. Limtrakul, Appl. Surf. Sci., 2021, 565, 150378 CrossRef CAS.
- G. Wang, R. Pandey and S. P. Karna, ACS Appl. Mater. Interfaces, 2015, 7, 11490 CrossRef CAS PubMed.
- G. S. Yi, E. S. Sim and Y.-C. Chung, Phys. Chem. Chem. Phys., 2017, 19, 28189 RSC.
- H. Lin, R. Jin, A. Wang, S. Zhu and H. Li, Ceram. Int., 2019, 45, 17996 CrossRef CAS.
- D. Singh, S. K. Gupta, T. Hussain, Y. Sonvane, P. Gajjar and R. Ahuja, Energy Fuels, 2021, 35, 9001 CrossRef CAS.
- S. Zhang, M. Xie, F. Li, Z. Yan, Y. Li, E. Kan, W. Liu, Z. Chen and H. Zeng, Angew. Chem., 2016, 128, 1698 CrossRef.
- X. Wang, J. Song and J. Qu, Angew. Chem., Int. Ed., 2019, 58, 1574 CrossRef CAS PubMed.
- D. R. Kripalani, A. A. Kistanov, Y. Cai, M. Xue and K. Zhou, Phys. Rev. B, 2018, 98, 085410 CrossRef CAS.
- J. Su, T. Duan, W. Li, B. Xiao, G. Zhou, Y. Pei and X. Wang, Appl. Surf. Sci., 2018, 462, 270 CrossRef CAS.
- S. Upadhyay and P. Srivastava, Mater. Chem. Phys., 2020, 241, 122381 CrossRef CAS.
- L. Zhang, P. Liang, H. B. Shu, X. L. Man, X. Q. Du, D. L. Chao, Z. G. Liu, Y. P. Sun, H. Z. Wan and H. Wang, J. Colloid Interface Sci., 2018, 529, 426 CrossRef CAS PubMed.
- L. Zhang, W. Zhao, S. Yuan, F. Jiang, X. Chen, Y. Yang, P. Ge, W. Sun and X. Ji, J. Energy Chem., 2021, 60, 531 CrossRef CAS.
- L. Ding, Q. Lu, A. D. C. Permana, S. Oswald, M. Hantusch, K. Nielsch and D. Mikhailova, Energy Technol., 2021, 9, 2001057 CrossRef CAS.
- F. Hu, H. Peng, T. Zhang, W. Shao, S. Liu, J. Wang, C. Wang and X. Jian, J. Energy Chem., 2021, 58, 115 CrossRef CAS.
- J. Zhao, Y. Yang, R. S. Katiyar and Z. Chen, J. Mater. Chem. A, 2016, 4, 6124 RSC.
- X. Gonze, B. Amadon, P.-M. Anglade, J.-M. Beuken, F. Bottin, P. Boulanger, F. Bruneval, D. Caliste, R. Caracas and M. Cote, et al., Comput. Phys. Commun., 2009, 180, 2582 CrossRef CAS.
- P. Blochl, Phys. Rev. B: Condens. Matter Mater. Phys., 1994, 50, 17953 CrossRef PubMed.
- N. A. W. Holzwarth, A. R. Tackett and G. E. Matthews, Comput. Phys. Commun., 2001, 135, 329 CrossRef CAS.
- C. Liu and X. Luo, J. Mater. Chem. B, 2021, 9, 2736 RSC.
- J. D. Head and M. C. Zerner, Chem. Phys. Lett., 1985, 122, 264 CrossRef CAS.
- W. Lee and X. Yao, Comput. Mater. Sci., 2015, 106, 76 CrossRef CAS.
- B. Zhang, H. Zhang, J. Lin and X. Cheng, Phys. Chem. Chem. Phys., 2018, 20, 30257 RSC.
- A. Zhang and X. Luo, Mater. Adv., 2022, 3, 5845 RSC.
- M. R. Kaiser, S. Chou, H.-K. Liu, S.-X. Dou, C. Wang and J. Wang, Adv. Mater., 2017, 29, 1700449 CrossRef PubMed.
- B. Wang, S. M. Alhassan and S. T. Pantelides, Phys. Rev. Appl., 2014, 2, 034004 CrossRef CAS.
- C. Barchasz, F. Molton, C. Duboc, J.-C. Leprêtre, S. Patoux and F. Alloin, Anal. Chem., 2012, 84, 3973 CrossRef CAS PubMed.
- Q. Zhang, X. Zhang, Y. Xiao, C. Li, H. H. Tan, J. Liu and Y. Wu, ACS Omega, 2020, 5, 29272 CrossRef CAS PubMed.
- T.-T. Yu, P.-F. Gao, Y. Zhang and S.-L. Zhang, Appl. Surf. Sci., 2019, 486, 281 CrossRef CAS.
- T. Li, C. He and W. Zhang, J. Mater. Chem. A, 2019, 7, 4134 RSC.
- L.-C. Yin, J. Liang, G.-M. Zhou, F. Li, R. Saito and H.-M. Cheng, Nano Energy, 2016, 25, 203 CrossRef CAS.
- X. Liu, N. Xu, T. Qian, J. Liu, X. Shen and C. Yan, Nano Energy, 2017, 41, 758 CrossRef CAS.
- V. Kolosnitsyn and E. Karaseva, Russ. J. Electrochem., 2008, 44, 506 CrossRef CAS.
- W. Wang, Y. Wang, Y. Huang, C. Huang, Z. Yu, H. Zhang, A. Wang and K. Yuan, J. Appl. Electrochem., 2010, 40, 321 CrossRef CAS.
- D. Aurbach, J. Power Sources, 2000, 89, 206 CrossRef CAS.
- R. Rauh, K. Abraham, G. Pearson, J. Surprenant and S. Brummer, J. Electrochem. Soc., 1979, 126, 523 CrossRef CAS.
- L. Lodovico, PhD thesis, dissertation, Karlsruher Institut für Technologie (KIT), Karlsruhe, 2019.
- U. Blukis, P. H. Kasai and R. J. Myers, J. Chem. Phys., 1963, 38, 2753 CrossRef CAS.
- Q. Zhang, Y. Wang, Z. W. Seh, Z. Fu, R. Zhang and Y. Cui, Nano Lett., 2015, 15, 3780 CrossRef CAS PubMed.
- R. A. Jarrin, et al., PhD thesis, Johns Hopkins University, 2023.
- T.-Z. Hou, X. Chen, H.-J. Peng, J.-Q. Huang, B.-Q. Li, Q. Zhang and B. Li, Small, 2016, 12, 3283 CrossRef CAS PubMed.
- C. Tang, H.-F. Wang, X. Chen, B.-Q. Li, T.-Z. Hou, B. Zhang, Q. Zhang, M.-M. Titirici and F. Wei, Adv. Mater., 2016, 28, 6845 CrossRef CAS PubMed.
- K. C. Wasalathilake, M. Roknuzzaman, K. K. Ostrikov, G. A. Ayoko and C. Yan, RSC Adv., 2018, 8, 2271 RSC.
|
This journal is © The Royal Society of Chemistry 2023 |