DOI:
10.1039/D3RA05298B
(Paper)
RSC Adv., 2023,
13, 25828-25835
A study on rapid and stable catalytic reduction of 4-nitrophenol by 2-hydroxyethylamine stabilized Fe3O4@Pt and its kinetic factors†
Received
5th August 2023
, Accepted 24th August 2023
First published on 30th August 2023
Abstract
The successful development of efficient and stable catalysts for 4-NP reduction reactions is beneficial to the environment and ecology. Fe3O4@Pt exhibits excellent catalytic performance for 4-NP reduction reaction due to the synergistic effect between Fe and Pt. But its structure and catalytic performance are extremely unstable. Here, we utilized the small-scale organic compound 2-hydroxyethylamine as surfactant to construct a stable composite nanomaterial. Then investigated the influence of monochromatic light (650 nm, 808 nm and 980 nm) and temperature on the kinetics of 4-NP reduction reaction by 2-hydroxyethylamine stabilized Fe3O4@Pt. The results indicate that both temperature and monochromatic light radiation can affect kinetic regulation. Increasing temperature can promote the catalytic rate, while monochromatic light radiation can induce agglomeration and inhibit the catalytic rate. This study opens up a new way for developing and regulating catalysts for heterogeneous catalysis reactions.
Introduction
4-Nitrophenol (4-NP) and nitroaromatic compounds are widely used as intermediates in pharmaceutical, pesticide and dye production.1 Their poor biodegradability, high solubility, toxicity and stability in water have caused many ecological problems.2–4 Therefore, most of the nitroaromatic compounds including 4-NP are listed as major pollutants by the U.S. Environmental Protection Agency.5–7 However, the reduced product 4-aminophenol (4-AP) can serve as an intermediate in the synthesis of various analgesic and antipyretic drugs,8 and other aniline derivatives, such as aniline, are important monomers for preparing functional polymers and can be widely used in materials science.9–12 Catalytic reduction of 4-NP in aqueous to obtain 4-AP and similar derived aminophenols is not only environmentally friendly, but also easy to operate and detect, and has gradually developed into an important research field. Therefore, designing and synthesizing efficient and stable catalysts is crucial for this field.
So far, various noble metal nanocatalysts have been successfully applied to 4-NP reduction reactions, but their catalytic performance varies. The catalytic performance of precious metal nanocatalysts largely depends on the inherent physical and chemical properties of the catalyst's nanostructure and composition, such as Au,13 Ag,14 Pd,15 Pt16 and mutual bimetallic nanomaterials.17–20 However, the agglomeration of precious nanocatalysts during catalytic reactions is inevitable.21 In order to achieve more satisfactory stability, as well as improved catalytic activity and selectivity, multi-component composite nanocatalysts are the natural choices. Reduced graphene oxide,22 polydopamine-wrapped reduced graphene oxide,23 metal–organic framework,24 carbon nanotubes,25 as well as inorganic salts CaCO3 (ref. 26) and γ-Al2O3,27 can all be used as support materials to construct composite nanomaterials with precious metals. In addition, support materials can also change the intrinsic electronic properties of metal nanoparticles or generate catalytic active site at the metal support interface through metal–support interaction, providing a key contribution to the overall catalytic activity.28–32
Although organic surfactants with large size and polymer hydrogels can be used to improve the stability of precious nanocatalysts, the surfactants or polymer hydrogels usually passivate the active surface, resulting in loss of catalytic activity or too long induction time.33–35 In our research group's published articles, large-sized sodium bicarbonate crystals can be used to improve the stability of Fe2O3@Pt, the residual polymer SiO2 usually passivates the active surface, leading to a loss of catalytic activity or long induction time.36
In addition, as suggested by Arrhenius equation, the rate of some catalytic reactions can be further increased by increasing the local temperature of the active site on the catalyst surface.37 Another method for regulating reaction kinetics is photoradiation, but most studies are based on the photothermal conversion of photothermal reagents or the localized surface plasmon resonance (LSPR) coupling effect to change the temperature of the solution to explore its impact on catalytic reduction performance.38–42 However, there are few reports on the effect of monochromatic light radiation on catalytic rate without causing temperature changes in the solution.
Herein, Fe3O4 has been chosen as a carrier for loading Pt atomic clusters due to its ability to be adsorbed by magnets and its ease of separation and recovery. Organic surfactant 2-hydroxyethylamine with small size is adsorbed on the surface of Fe3O4@Pt composite nanomaterials to prevent the separation and aggregation of Pt and Fe3O4, and excessive 2-hydroxyethylamine can form a supporting membrane, thereby stabilizing its structural characteristics without affecting its catalytic performance. In addition, the influence of monochromatic light (650 nm, 808 nm and 980 nm) and temperature on the catalytic reduction of 4-nitrophenol were investigated via 2-hydroxyethylamine stabilized Fe3O4@Pt.
Experimental section
Materials
Chloroplatinic acid hexahydrate (H2PtCl6·6H2O) and 4-nitrophenol (4-NP) were obtained from Aladdin reagent (Shanghai) Co., Ltd. Ethanol was obtained from Beijing Chemical Reagent. FeSO4 was obtained from Sinopharm Chemical Reagent Co., Ltd. NaBH4 was obtained from Jinan Yuxin Chemical Co., Ltd. 2-Hydroxyethylamine purchased from Yantai Shuangshuang Chemical Co., Ltd. All reagents used in this study are analytically pure and have not been purified in any way except for special labels. All experiments used deionized water.
Synthesis of Fe3O4@Pt
First, distilled water (5 mL) and FeSO4 (4 mg) were added into a 50 mL beaker for ultrasonic dispersion. Then add ethanol (5 mL) and H2PtCl6 aqueous solution (0.2 mL, 1 g/100 mL). After fully stirring, NaBH4 powder (5 mg) was added into the mixed system. After stirring for 10 minutes, centrifuge and separate. Discard the supernatant, wash the sediment with distilled water, and then disperse the sediment in 2 mL distilled water for standby.
Synthesis of 2-hydroxyethylamine stabilized Fe3O4@Pt
The preparation method of 2-hydroxyethylamine stabilized Fe3O4@Pt is the same as that of Fe3O4@Pt, but the difference is that different amounts of 2-hydroxyethylamine (0, 0.1, 0.2, 0.3, 0.4 and 0.5 mL) need to be added at the same time as 5 mg NaBH4.
The catalytic reduction performance test for 4-NP
The catalytic reduction performance test was conducted using a 4 mL quartz cuvette as the reaction vessel at room temperature. The specific operating steps are as follows: H2O (3 mL), 4-NP (20 μL, 0.01 mol L−1), Fe3O4@Pt or 2-hydroxyethylamine stabilized Fe3O4@Pt (20 μL, 0.001931 mol L−1, taking from 2 mL stock solution) were successively added into a 4 mL quartz colorimetric dish. After the solution was completely dispersed evenly, fresh NaBH4 (100 μL, 0.2 mol L−1) aqueous solution is dropped into the above solution. The molar ratio of NaBH4/4-NP is 100, so the amount of NaBH4 is excess compared to 4-NP. The absorbance performance during reaction was monitored by UV-vis spectra (in the range of 250–500 nm) or 722N spectrophotometer (record the absorbance value at λ = 400 nm).
The catalytic reduction performance test under different temperatures and light radiation is similar to the above method, except that before starting the test, the aqueous solution containing 4-NP and catalyst is heated to constant temperature in a constant temperature water bath or continuously irradiated for 1 minute under a specific wavelength and power of laser.
Characterization
The powder X-ray diffraction (XRD) pattern was measured on a Panaco X′ Pert PRO X-ray diffractometer with Cu Ka radiation (l = 0.15406 nm, the operation voltage: 45 kV, the operation current: 40 mA, scanning speed: 1° min−1, test range: 10° ≤ 2θ ≤ 70°). The transmission electron microscope (TEM) images were obtained on a FEI Talos F200S transmission electron microscope (the operation voltage: 200 kV). The Fine spectra of Fe and Pt were determined by Thermo ESCALAB 250XI X-ray photoelectron spectroscopy (XPS). The magnetometer hysteresis loops was measured on LakeShore 7404. The UV-vis absorption spectra were measured on Shimadzu UV-1780 UV-vis-NIR spectrophotometer. The absorbance at λ = 400 nm was monitored by a 722N visible spectrophotometer.
Results and discussion
Synthesis and characterization of 2-hydroxyethylamine stabilized Fe3O4@Pt
Fig. 1 shows the XRD results of Fe3O4@Pt and 2-hydroxyethylamine stabilized Fe3O4@Pt, the diffraction peak at about 40° is attributed to Pt nanoparticles, and the other diffraction peaks are consistent with the cubic phase of Fe3O4 (JCPDS: 75-0449). A series of weak diffraction peaks were observed in 35.76, 57.51 and 63.17, corresponding to (311), (511) and (440) crystal planes, respectively. When 2-hydroxyethylamine is added, 2-hydroxyethylamine adsorbs on the surface of the composite nanomaterial, so the diffraction peak of Fe3O4 weakens significantly. What's more, 2-hydroxyethylamine stabilized Fe3O4@Pt has the ability to be adsorbed by magnets and is easy to separate and recover. Its saturation magnetization, residual magnetic field strength and coercive force were 27.9 emu g−1, 5.9 emu g−1 and 138.7 G, respectively (Fig. S1†). The TEM images of Fe3O4@Pt (Fig. 2a and b) show that Pt clusters of approximately 2 nm uniformly dot the surface of Fe3O4, and the overall uniformity and dispersion are particularly good. But this structure is extremely unstable, and Pt and Fe3O4 will quickly separate and aggregate when stored in aqueous solution for 3 hours (Fig. 2c and d). Fig. 2e and f show the TEM images of 2-hydroxyethylamine stabilized Fe3O4@Pt. 2-Hydroxyethylamine can adsorb on the surface of composite nanomaterials to form a protective layer, preventing the separation and aggregation of Pt and Fe3O4, and the excess can spontaneously form a supporting membrane, thereby improving the stability of composite nanocatalysts. As shown in Fig. S2,† the addition of 2-hydroxyethylamine has no effect on the XPS fine spectra of Fe 2p and Pt 4f. The high-resolution XPS spectra of Fe 2p (Fig. 3a) indicated that 2-hydroxyethylamine stabilized Fe3O4@Pt were primarily composed of Fe2+ corresponded to the peaks at 710.2 eV and 723.3 eV, and the peaks at 711.7 and 725.1 eV were assigned to Fe3+. The two valence states of Fe element are consistent with XRD results, which once again confirms that the crystal phase of the prepared sample is Fe3O4. The peaks at 71.1 and 74.4 eV were originated from the presence of metallic Pt (Fig. 3b).
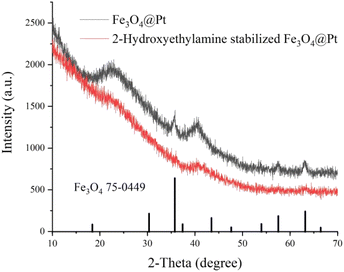 |
| Fig. 1 X-ray powder diffraction (XRD) patterns of Fe3O4@Pt and 2-hydroxyethylamine stabilized Fe3O4@Pt. | |
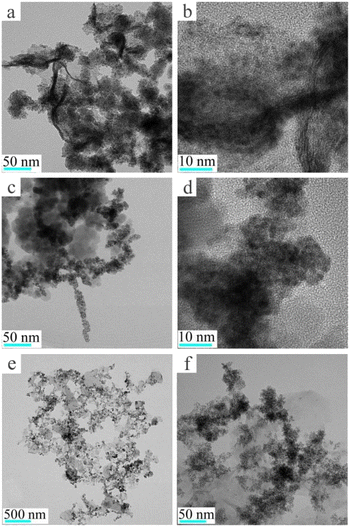 |
| Fig. 2 Transmission electron microscopy (TEM) images of (a and b) freshly prepared Fe3O4@Pt, (c and d) Fe3O4@Pt after 3 hours of storage, (e and f) 2-hydroxyethylamine stabilized Fe3O4@Pt. | |
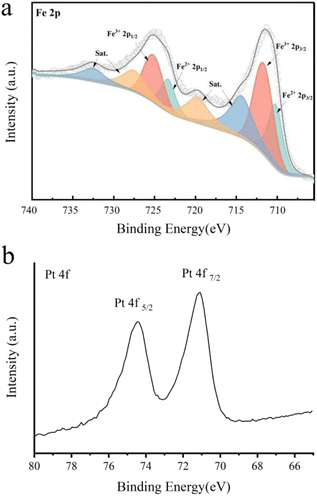 |
| Fig. 3 XPS high-resolution spectra of (a) Fe 2p and (b) Pt 4f of 2-hydroxyethylamine stabilized Fe3O4@Pt. | |
Catalytic reduction performance of 2-hydroxyethylamine stabilized Fe3O4@Pt for 4-NP
The catalytic reduction of 4-NP to 4-AP in aqueous phase has become an important research field. On the contrary, because of its simplicity and repeatability, the catalytic reduction of 4-NP with NaBH4 under aqueous phase has become a benchmark model reaction to quantify the catalytic activity of 2-hydroxyethylamine stabilized Fe3O4@Pt. As shown in Fig. S3,† the intensity of absorption peak at 400 nm belongs to the 4-NP, 20 μL, 1.9 mM 2-hydroxyethylamine stabilized Fe3O4@Pt can trigger the decrease of the absorption peak at 400 nm, and the formation of a new peak belongs to 4-AP at 300 nm. Therefore, the absorbance value at 400 nm could represent the concentration of 4-NP, and the reduction process can be easily monitored using a 722N spectrophotometer. Since the amount of NaBH4 is excess compared to 4-NP, the reaction kinetics follows the pseudofirst-order law, the apparent kinetic rate constant kapp can be described as eqn (1): |
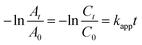 | (1) |
where C0 and A0 stand for the initial concentration and absorbance of 4-NP, while Ct and At correspond to time t.
As a confirmatory experiment, the catalytic reduction performance of a series of 2-hydroxyethylamine (0, 0.1, 0.2, 0.3, 0.4 and 0.5 mL) stabilized Fe3O4@Pt for 4-NP were characterized. As can be seen in Fig. 4a, the catalytic effect of 2-hydroxyethylamine stabilized Fe3O4@Pt under different amounts of 2-hydroxyethylamine are all excellent, a small quantity of catalysts (20 μL, 1.9 mM) with different specifications can trigger the 100% conversion of 4-NP to 4-AP within merely 40 s (Fig. 4b). When the catalyst is stored in aqueous solution for 48 h, the catalytic effect of 2-hydroxyethylamine stabilized Fe3O4@Pt for 4-NP is much better than that without 2-hydroxyethylamine, and the conversion efficiency of 4-NP by 2-hydroxyethylamine stabilized Fe3O4@Pt was up to 100% in 200 s, which was significantly higher than that without 2-hydroxyethylamine (Fig. 4c and d). The catalytic reduction process of 4-NP with different specifications of catalysts under different storage times were shown in Fig. S4–S6.† As described in eqn (1), there is a linear relationship between −ln(At/A0) and t. The apparent kinetic rate constant kapp of different specifications of catalysts under different storage times was shown in Fig. 4e. Although kapp decreases with increasing storage time, Fe3O4@Pt protected with 0.2 mL 2-hydroxyethylamine has always been the optimal catalytic rate (0.1409 s−1 for newly prepared and 0.04615 s−1 for storage after 48 h) and the shortest reaction time tend (30 s for newly prepared and 110 s for storage after 48 h) (Fig. 4f). The author characterized the TEM image of 2-hydroxyethylamine stabilized Fe3O4@Pt under different amounts of 2-hydroxyethylamine (0.2 mL and 0.5 mL). As shown in Fig. S7,† the amount of 2-hydroxyethylamine does not change the particle size and textural properties, but excessive 2-hydroxyethylamine will completely adsorb on the surface of Fe3O4@Pt, even encapsulating Fe3O4@Pt, thus passivating the active surface and reducing its catalytic activity. Therefore, the experiment confirmed that 2-hydroxyethylamine indeed has a protective effect on the Fe3O4@Pt structure, and 0.2 mL is the optimal amount. For comparing our catalytic reduction effect for 4-NP with those reported catalysts, the turnover frequency TOF, defined as moles of reactant reduced through per mole of catalyst per second, was calculated to be 621 h−1 for newly prepared, and 166 h−1 for storage after 48 h. The TOF value is much higher than that of noble metal catalysts (Table 1), indicating that 2-hydroxyethylamine stabilized Fe3O4@Pt has excellent catalytic reduction activity for 4-NP. Finally, the reusability of 2-hydroxyethylamine stabilized Fe3O4@Pt was characterized. As shown in Fig. S8,† the catalytic performance remains good after 5 cycles, once again verifying the excellent catalytic stability of 2-hydroxyethylamine stabilized Fe3O4@Pt.
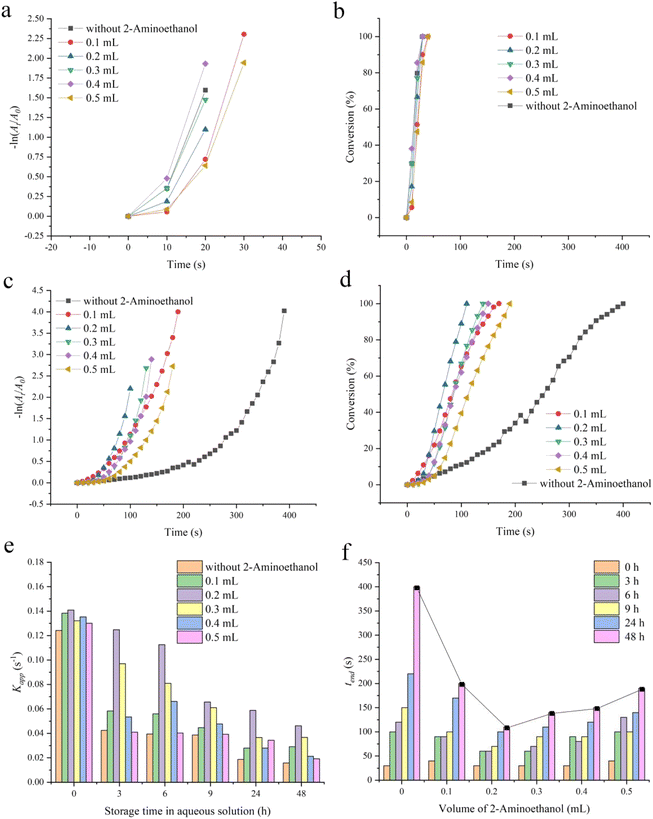 |
| Fig. 4 Plots of −ln(At/A0) and conversion versus the reaction time t for 4-NP catalyzed by 2-hydroxyethylamine stabilized Fe3O4@Pt under different conditions: (a and b) freshly prepared, (c and d) after 48 hours of storage. (e and f) Plots of reaction rate kapp and reaction end time tend versus the reaction time t under different amounts of 2-hydroxyethylamine and storage time. All reactions take place at room temperature, the catalyst concentration is 0.013 mM, and the molar ratio of NaBH4/4-NP is 100. | |
Table 1 Comparison of the kinetic parameter of 2-hydroxyethylamine stabilized Fe3O4@Pt for 4-NP with that of previous work. In this work, the catalyst concentration is measured by Pt concentration
Catalyst |
Reaction time |
4-NP concentration (mM) |
Catalyst concentration |
Rate constant (10−3 s−1) |
TOF/h−1 |
Ref. |
Pd/RGO/Fe3O4 |
60 s |
2.5 |
5 mg |
51 |
— |
43 |
Pd-GA/RGO |
5 min |
5 |
1–20 mg |
7 |
— |
44 |
Cu/Pd@graphitic carbon |
— |
0.05 |
0.083 mM |
80 |
108 |
45 |
AgNPs/SiNSs |
40 s |
0.12 |
0.051 mM |
80 |
200 |
46 |
PtAu-PDA/RGO |
3 min |
0.1 |
0.01 mM |
9.58 |
200 |
47 |
Sodium sesquicarbonate-supporting Fe2O3@Pt |
4 min |
0.067 |
0.013 mM |
13.98 |
78 |
36 |
2-Hydroxyethylamine stabilized Fe3O4@Pt |
30 s |
0.067 |
0.013 mM |
90.93 |
621 |
This work |
The effect of temperature on the catalytic reduction performance of 2-hydroxyethylamine stabilized Fe3O4@Pt for 4-NP
As we previously described, the catalytic rate of 20 μL, 0.013 mM 2-hydroxyethylamine stabilized Fe3O4@Pt for 4-NP was too high (the apparent kinetic rate constant Kapp = 0.1409 s−1) to analyze the effect of temperature on its catalytic performance. So the amount of catalyst (5, 10, 15 and 20 μL) was carefully investigated. As presented in Fig. 5a, 10 μL, 0.013 mM 2-hydroxyethylamine stabilized Fe3O4@Pt has a relatively moderate catalytic rate (Kapp = 0.03075 s−1) and is applied to explore the effect of temperature for 4-NP. As shown in Fig. 5b–d, the conversion efficiency and reaction rates Kapp increase with the increase of reaction temperature. When the reaction reaches 60 s, the conversion of the 60 °C system has reached 100%, while that of the room temperature system is only 54.14%. The temperature increased from 18 °C to 60 °C, kapp increased from 0.03075 to 0.08579 s−1, an increase of 2.8 times. The TOF also increased from 305.87 to 582.82 h−1, an increase of 1.9 times. The results as suggested by Arrhenius equation,37 the catalytic rate for 4-NP can be further increased by increasing the local temperature of the active site on the catalyst surface. Meanwhile, it has been proven that 2-hydroxyethylamine stabilized Fe3O4@Pt has good thermal stability.
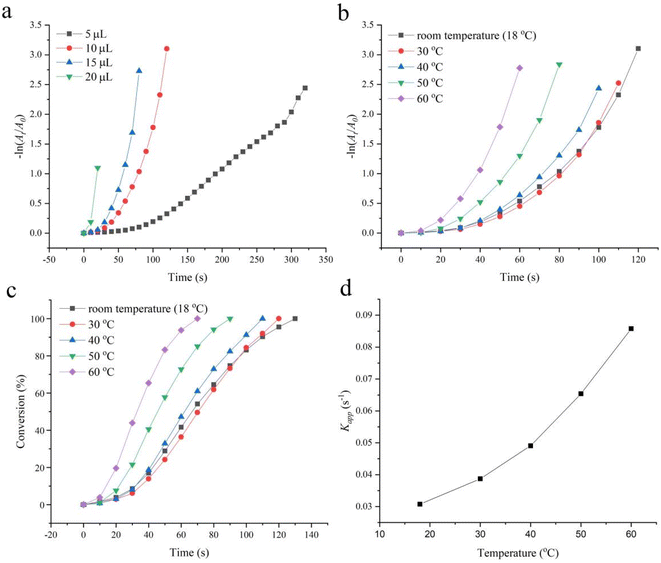 |
| Fig. 5 Plots of −ln(At/A0) versus the reaction time t for 4-NP under (a) different dosages of catalysts, (b) different reaction temperatures. (c) Time dependent conversion of 4-NP at different temperatures and (d) the trend of reaction rate kapp changing with temperature. | |
The effect of light on the catalytic reduction performance of 2-hydroxyethylamine stabilized Fe3O4@Pt for 4-NP
Another method of adjusting reaction kinetics is to change the temperature of the catalyst surface through photothermal transformation. However, most photothermal research is based on converting light energy into thermal energy through photothermal materials to increase the temperature of the entire solution and explore changes in catalytic performance.38–42 This manuscript explores the effect of light radiation on catalytic efficiency without increasing the entire solution temperature. As shown in Fig. S9,† 2-hydroxyethylamine stabilized Fe3O4@Pt has absorption throughout the entire UV visible and near-infrared regions. Therefore, three types of monochromatic light with good penetrability (650 nm, 808 nm and 980 nm) were selected to explore the impact of light radiation on catalytic performance. Fig. S10† shows the temperature changes of aqueous solutions caused by light radiation at different radiation wavelengths and powers. 650 nm monochromatic light with power of 1 w, 2 w, and 3 w continuously radiated for 60 s, causing little thermal effect and no change in solution temperature. The 808 nm radiation caused minimal thermal effect, leading to an increase in solution temperature of 1 °C for 1 w and 2 °C for 2–3 w. However, 980 nm radiation causes significant thermal effects, 3 °C for 1 w, 7 °C for 2 w and 10 °C for 3 w. The effect of light on the catalytic reduction performance of 2-hydroxyethylamine stabilized Fe3O4@Pt for 4-NP is shown in Fig. 6. Under different wavelengths and power radiation, it causes a decrease in catalytic rate and an extension of catalytic reaction end time tend. For 650 nm and 808 nm monochromatic light, the thermal effect caused by power changes is not significant, so the catalytic rate does not change significantly at different powers (Fig. 6a and b). However, for 980 nm monochromatic light, the thermal effect caused by the increase in power is significant, so the catalytic rate gradually increases (Fig. 6c). Fig. 7 compares the effect of different wavelengths of radiation light at 1 w on the catalytic reduction for 4-NP. The comparison results clearly indicate that as the wavelength increases, the conversion rate and catalytic rate both significantly decrease (the kapp without light radiation, at 650 nm, 808 nm, and 980 nm radiation are 0.03075, 0.02832, 0.01781, and 0.01441 s−1, respectively). Therefore, it can be concluded that monochromatic light radiation has an inhibitory effect on 2-hydroxyethylamine stabilized Fe3O4@Pt catalytic reduction of 4-NP, and the longer the wavelength, the more obvious the inhibitory effect. The inhibition effect caused by monochromatic radiation may be attributed to the aggregation of Pt clusters driven by photogenerated electrons and their transfer to surface Pt atoms.48
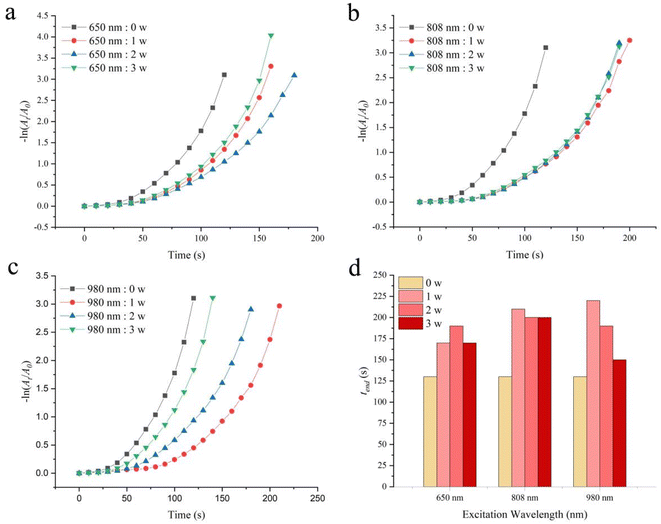 |
| Fig. 6 Plots of −ln(At/A0) versus the reaction time t for 4-NP under different wavelengths and power light radiation: (a) 650 nm, (b) 808 nm, (c) 980 nm. (d) The relationship between reaction end time tend and radiation wavelength and radiation power. | |
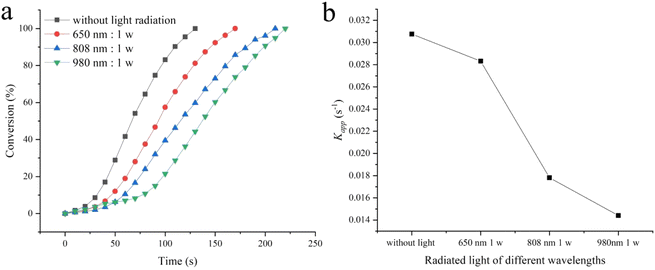 |
| Fig. 7 (a) The conversion versus the reaction time t for 4-NP under different wavelengths of radiation light at 1 w, (b) the trend of reaction rate kapp changing with radiation wavelength at 1 w. | |
Conclusions
In summary, 2-hydroxyethylamine stabilized Fe3O4@Pt composite nanocatalyst is developed via a one-pot route. The composite nanocatalyst exhibits a stable and enhanced catalytic performance for 4-NP reduction reaction. The value of the reaction rate kapp and TOF are also higher than many other reported catalysts. Moreover, the dynamic adjustment experiment shows that increasing temperature can promote the catalytic rate, while monochromatic light radiation (650 nm, 808 nm and 980 nm) inhibits the catalytic rate. In conclusion, we believe that 2-hydroxyethylamine stabilized Fe3O4@Pt composite nanocatalyst will have wide application attraction in 4-NP reduction reaction.
Conflicts of interest
There are no conflicts to declare.
Acknowledgements
The authors are grateful for the financial aid from the National Natural Science Foundation of China (Grant No. 21961001) and the institution of higher education of Gansu Province Foundation for Young doctor (2022QB-079).
Notes and references
- Y. Wang, Y. Xie, H. Sun, J. Xiao, H. Cao and S. Wang, Catal. Sci. Technol., 2016, 6, 2918 RSC.
- W. J. Liu, K. Tian, H. Jiang and H. Q. Yu, Green Chem., 2014, 16, 4198 RSC.
- Y. Liu, Y. Fan, Y. Yuan, Y. Chen, F. Cheng and S. C. Jiang, J. Mater. Chem., 2012, 22, 21173 RSC.
- F. Wang, S. Y. Song, K. Li, J. Q. Li, J. Pan, S. Yao, X. Ge, J. Feng, X. Wang and H. J. Zhang, Adv. Mater., 2016, 28, 10679 CrossRef CAS PubMed.
- Y. Q. Jiang, J. J. Zhang and R. Balasubramanian, J. Environ. Chem. Eng., 2022, 10, 107215 CrossRef.
- Y. X. He, N. Cheshomi, S. M. Lawson, A. K. Itta, F. Reazei, S. Kapila and A. A. Rownaghi, Chem. Eng. J., 2021, 410, 128326 CrossRef CAS.
- K. P. Mishra and P. R. Gogate, Ultrason. Sonochem., 2011, 18, 739 CrossRef CAS PubMed.
- F. Coccia, L. Tonucci, D. Bosco, M. Bressan and N. d'Alessandro, Green Chem., 2012, 14, 1073 RSC.
- C. Li, L. Zhu, L. Song, G. Zhu, Q. Zhang, Y. Zhao, Q. Gong, C. Sun, Y. Liu, K. Sasakid and J. Xie, J. Mater. Chem. A, 2023, 11, 7756 RSC.
- W. Li, F. Wang, Y. Shi and L. Yu, Chin. Chem. Lett., 2023, 34, 107505 CrossRef CAS.
- H. Wan, C. Qin and A. Lu, J. Mater. Chem. A, 2022, 10, 17279 RSC.
- Z. Zeng, Y. Chen, X. Zhu and L. Yu, Chin. Chem. Lett., 2023, 34, 107728 CrossRef CAS.
- P. Zhao, X. Feng, D. Huang, G. Yang and D. Astruc, Coord. Chem. Rev., 2015, 287, 114 CrossRef CAS.
- P. Zhang, C. Shao, Z. Zhang, M. Zhang, J. Mu, Z. Guo and Y. Liu, Nanoscale, 2011, 3, 3357 RSC.
- Q. Wang, W. Jia, B. Liu, A. Dong, X. Gong, C. Li, P. Jing, Y. Li, G. Xua and J. Zhang, J. Mater. Chem. A, 2013, 1, 12732 RSC.
- S. K. Ghosh, M. Mandal, S. Kundu, S. Nath and T. Pal, Appl. Catal., A, 2004, 268, 61 CrossRef CAS.
- Z. Dong, X. Le, C. Dong, W. Zhang, X. Li and J. Ma, Appl. Catal., B, 2015, 162, 372 CrossRef CAS.
- M. Nasrollahzadeh, S. M. Sajadi, A. Rostami-Vartooni and M. Bagherzadeh, J. Colloid Interface Sci., 2015, 448, 106 CrossRef CAS PubMed.
- X. F. Tan, J. Qin, Y. Li, Y. T. Zeng, G. X. Zheng, F. Feng and H. Li, J. Hazard. Mater., 2020, 397, 122786 CrossRef CAS PubMed.
- J. Qin, X. F. Tan, F. Feng and H. Li, Appl. Surf. Sci., 2021, 561, 150024 CrossRef CAS.
- M. Nasrollahzadeh and A. Banaei, Tetrahedron Lett., 2015, 56, 500 CrossRef CAS.
- A. T. Ezhil Vilian, S. R. Choe, K. Giribabu, S. C. Jang, C. Roh, Y. S. Huh and Y. K. Han, J. Hazard. Mater., 2017, 333, 54 CrossRef PubMed.
- W. Ye, J. Yu, Y. Zhou, D. Gao, D. Wang, C. Wang and D. Xue, Appl. Catal., B, 2016, 181, 371 CrossRef CAS.
- Z. Dong, X. Le, Y. Liu, C. Dong and J. Ma, J. Mater. Chem. A, 2014, 2, 18775 RSC.
- C. H. Liu, J. Liu, Y. Y. Zhou, X. L. Cai, Y. Lu, X. Gao and S. D. Wang, Carbon, 2015, 94, 295 CrossRef CAS.
- Q. Ding, Z. Kang, L. Cao, M. Lin, H. Lin and D. P. Yang, Appl. Surf. Sci., 2020, 510, 145526 CrossRef CAS.
- F. Saira, N. Firdous, R. Qureshi and A. Ihsan, Turk. J. Chem., 2020, 44, 448 CrossRef CAS PubMed.
- Q. Fu, H. Saltsburg and M. Flytzani-Stephanopoulos, Science, 2003, 301, 935 CrossRef CAS PubMed.
- M. Valden, X. Lai and D. W. Goodman, Science, 1998, 281, 1647 CrossRef CAS PubMed.
- M. Cargnello, V. V. T. Doan-Nguyen, T. R. Gordon, R. E. Diaz, E. A. Stach, R. J. Gorte, P. Fornasiero and C. B. Murray, Science, 2013, 341, 771 CrossRef CAS PubMed.
- X. Y. Liu, M. H. Liu, Y. C. Luo, C. Y. Mou, S. D. Lin, H. K. Cheng, J. M. Chen, J. F. Lee and T. S. Lin, J. Am. Chem. Soc., 2012, 134, 10251 CrossRef CAS PubMed.
- J. L. Shi, Chem. Rev., 2013, 113, 2139 CrossRef CAS PubMed.
- S. Saha, A. Pal, S. Kundu, S. Basu and T. Pal, Langmuir, 2010, 26, 2885 CrossRef CAS PubMed.
- W. C. Elias, R. Eising, T. R. Silva, B. L. Albuquerque, E. Martendal, L. Meier and J. B. Domingos, J. Phys. Chem. C, 2014, 118, 12962 CrossRef CAS.
- S. M. Ansar and C. L. Kitchens, ACS Catal., 2016, 6, 5553 CrossRef CAS.
- X. Xu, M. Li, L. Yang and B. Hu, RSC Adv., 2023, 13, 13556 RSC.
- K. J. Laidler, J. Chem. Educ., 1984, 61, 494 CrossRef CAS.
- K. Wang, X. Zhu, Y. Yang, D. Ye, R. Chen and Q. Liao, J. Environ. Chem. Eng., 2022, 10, 108253 CrossRef CAS.
- M. Kang and Y. Kim, J. Ind. Eng. Chem., 2020, 86, 61 CrossRef CAS.
- J. H. Kim, B. W. Lavin, B. W. Boote and J. A. Pham, J. Nanopart. Res., 2012, 14, 995 CrossRef.
- Y. Gu, Y. Jiao, X. Zhou, A. Wu, B. Buhe and H. Fu, Nano Res., 2018, 11, 126 CrossRef CAS.
- Z. Wang, W. Wang, M. Wamsley, D. Zhang and H. Wang, ACS Appl. Mater. Interfaces, 2022, 14, 17560 CrossRef CAS PubMed.
- M. Atarod, M. Nasrollahzadeh and S. M. Sajadi, J. Colloid Interface Sci., 2016, 465, 249 CrossRef CAS PubMed.
- A. Vilian, S. R. Choe, K. Giribabu, S. Jang, C. Roh, Y. S. Huh and Y. Han, J. Hazard. Mater., 2017, 333, 54 CrossRef CAS PubMed.
- M. Morales, M. Rocha, C. Freire, E. Asedegbega-Nieto, E. Gallegos-Suarez, I. Rodríguez-Ramos and A. Guerrero-Ruiz, Carbon, 2017, 111, 150 CrossRef CAS.
- Z. Yan, L. Fu, X. Zuo and H. Yang, Appl. Catal., B, 2018, 226, 23 CrossRef CAS.
- W. Ye, J. Yu, Y. Zhou, D. Gao, D. Wang, C. Wang and D. Xue, Appl. Catal., B, 2016, 181, 371 CrossRef CAS.
- N. Denisov, S. Qin, J. Will, B. N. Vasiljevic, N. V. Skorodumova, I. A. Pašti, B. B. Sarma, B. Osuagwu, T. Yokosawa, J. Voss, J. Wirth, E. Spiecker and P. Schmuki, Adv. Mater., 2023, 35, 2206569 CrossRef CAS PubMed.
|
This journal is © The Royal Society of Chemistry 2023 |