DOI:
10.1039/D3RA04852G
(Paper)
RSC Adv., 2023,
13, 26509-26515
Self-assembled molybdenum disulfide nanoflowers regulated by lithium sulfate for high performance supercapacitors†
Received
19th July 2023
, Accepted 31st August 2023
First published on 4th September 2023
Abstract
Recently, molybdenum disulfide (MoS2) has been extensively investigated as a promising pseudocapacitor electrode material. However, MoS2 usually exhibits inferior rate capability and cyclability, which restrain its practical application in energy storage. In this work, MoS2 nanoflowers regulated by Li2SO4 (L-MoS2) are successfully fabricated via intercalating solvated Li ions. Via appropriate intercalation of Li2SO4, MoS2 nanosheets could self-assemble to form L-MoS2 nanoflowers with an interlayer spacing of 0.65 nm. Due to the large specific surface area (23.7 m2 g−1) and high 1T phase content (77.5%), L-MoS2 as supercapacitor electrode delivers a maximum specific capacitance of 356.7 F g−1 at 1 A g−1 and maintains 49.8% of capacitance retention at 20 A g−1. Moreover, the assembled L-MoS2 symmetric supercapacitor (SSC) device displays an energy density of 6.5 W h kg−1 and 79.6% of capacitance retention after 3000 cycles.
1. Introduction
Molybdenum disulfide (MoS2), a typical two-dimensional (2D) transition metal chalcogenide, which is composed of S–Mo–S layers vertically stacked via weak van der Waals attraction.1 Owing to the characteristics of high theoretical capacity and electrochemical activity, relatively large and easily expanded interlayer distance, easy preparation, and low cost, MoS2 has been extensively investigated in the field of supercapacitors, batteries and electrocatalysts.2–7 However, MoS2 usually suffers from serious stacking and agglomeration problems during the preparation process, which leads to formation of many inaccessible active sites.8 As a pseudocapacitive electrode material of supercapacitors, the low conductivity and large volume variation during repeated charge/discharge cycles also give rise to inferior rate capability and cycling stability of MoS2, which restrains its practical application in energy storage.9 Therefore, much efforts are devoted to ameliorating the capacitive performances of MoS2.
To address the aforementioned drawbacks, endowing MoS2 with various kinds of nanostructures can improve its electrochemical performances. The nanostructure design can effectively avoid the stacking and agglomeration problems of MoS2 and expose more electroactive sites, which is beneficial to increasing the contact area with electrolyte ions. For instance, Kesavan et al. synthesized MoS2 nanosheets via topochemical sulfurization, which revealed a high capacitance of 119.38 F g−1 and good cyclability of 95.1% over 2000 cycles.10 Wei et al. fabricated MoS2 nanoflowers via adding sodium chloride, which displayed a high capacity of 1120 F g−1 at 0.5 A g−1 and 96% of capacitance retention after 2000 cycles.11 Broadening the interlayer spacing of MoS2 is also an effective method to enhance the rate capability and cycling stability, since large interlayer spacing can afford rapid diffusion transportation of electrolyte ions between MoS2 bilayers. Wang et al. prepared MoS2 micro flowers with an interlayer spacing of 0.94 nm by reactant conversion-intercalation strategy, which delivered a specific capacity of 246.8 F g−1 at 0.5 A g−1.12 Cai et al. fabricated PEDOT@MoS2 composite with an interlayer spacing of 1.02 nm via electrochemical co-deposition method, which exhibited a high specific capacity of 4418 mF cm−2 at 2 mA cm−2 and 100% of capacitance retention after 10
000 cycles.13 In addition, the usually synthesized MoS2 is stable semiconducting phase (2H-MoS2) with low conductivity, while metallic phase (1T-MoS2) is thermodynamically metastable but reveals better conductivity and hydrophilicity than 2H-MoS2.14–16 Therefore, developing 1T phase dominated hybrid phase MoS2 is the optimal strategy to ameliorate the electrochemical properties of MoS2. For example, Li et al. prepared high purity MoS2 nanosheets with 83.6% of 1T phase, which achieved a high capacitance of 392 F g−1 at 1 A g−1 and 83% of capacitance retention after 10
000 cycles.17 Although some progresses have been made in enhancing the electrochemical performances of MoS2-based electrodes, however, the preparation process of MoS2 usually requires a high hydrothermal reaction temperature (usually above 200 °C), and the specific capacity and rate capability of MoS2-based electrodes is still unsatisfactory, it is urgent to develop simple and low temperature strategies to ameliorate the specific capacity and rate capability of MoS2-based electrodes.
Inspired by the above literature, in this work, MoS2 nanoflowers regulated by Li2SO4 (L-MoS2) are successfully fabricated via intercalating solvated Li ions in a relatively low hydrothermal reaction temperature (180 °C). Under appropriate intercalation of Li2SO4, MoS2 nanosheets could self-assemble to form L-MoS2 nanoflowers with an interlayer spacing of 0.65 nm. Due to the large specific surface area (23.7 m2 g−1) and high 1T phase content (77.5%), L-MoS2 as supercapacitor electrode delivers a maximum specific capacitance of 356.7 F g−1 at 1 A g−1 and maintains 49.8% of initial capacity at 20 A g−1. Moreover, the assembled L-MoS2 symmetric supercapacitor (SSC) device displays an energy density of 6.5 W h kg−1 at 413 W kg−1 and 79.6% of capacitance retention after 3000 cycles.
2. Results and discussion
2.1. Characterization
In this work, L-MoS2 nanoflowers were self-assembled from MoS2 nanosheets by intercalating moderate amount of Li2SO4 and the detailed preparation process of L-MoS2 nanoflowers is shown in the ESI.† The morphologies of the obtained samples were first observed by scanning electron microscope (SEM). When Li2SO4·H2O was not added in the hydrothermal reaction, the obtained MoS2 is composed of intersecting nanosheets with thickness about 20 nm and reveals an obvious stacking/agglomeration phenomenon, as illustrated in Fig. S1a and b.† When 0.64 g Li2SO4·H2O was added in the hydrothermal reaction, the solvated Li ions carrying water molecules intercalated into the MoS2 bilayers, broadening the interlayer spacing of MoS2 and introducing negative charges on the MoS2 nanosheets.18 The strong electrostatic repulsive force between negative charges on adjacent MoS2 nanosheets affects the growth of MoS2 and induces the self-assembly of MoS2 nanosheets to form L-MoS2 nanoflowers. As present in Fig. 1, the SEM images of L-MoS2 exhibit perfect nanoflowers-like architecture without distinct agglomeration phenomenon. According to the four-probe tests, the conductivity of L-MoS2 powder is 0.15 S cm−1, which is higher than that of MoS2 powder (0.11 S cm−1). It is noteworthy that the amount of Li2SO4·H2O added manifests an obvious effect on the morphology of L-MoS2. When the amount of Li2SO4·H2O added was 0.32 g, the solvated Li ions intercalated into the MoS2 bilayers, introducing fewer negative charges on the MoS2 nanosheets. The relatively weak electrostatic repulsive force between negative charges on adjacent MoS2 nanosheets only induces partial self-assembly of MoS2 nanosheets to form nanoflowers, leading to the resultant L-MoS2-2.5 still presents stacking/agglomeration due to interlayer van der Waals attraction, as verified by the SEM images in Fig. S1c and d.† When the amount of Li2SO4·H2O added was 0.96 g, the solvated Li ions intercalated into the MoS2 bilayers, introducing more negative charges on the MoS2 nanosheets. The stronger electrostatic repulsive force between negative charges on adjacent MoS2 nanosheets induces the disorderly growth of MoS2 nanosheets, which inevitably leads to the local collapse and agglomeration of the obtained L-MoS2-7.5, as proved by the SEM images in Fig. S1e and f.†
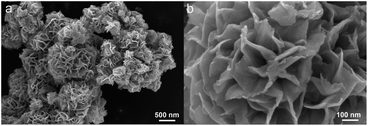 |
| Fig. 1 SEM images of L-MoS2 nanoflowers (a and b). | |
The micro morphology of the samples was further observed by transmission electron microscope (TEM) tests. The TEM image in Fig. 2a further affirms that L-MoS2 is self-assembled from thin and porous MoS2 nanosheets. The high-resolution TEM (HRTEM) image in Fig. 2b exhibits an interlayer spacing of 0.65 nm, assigning to the (002) plane of 2H-MoS2. Furthermore, the discontinuous lattice fringes marked within the dotted circles in Fig. 2b demonstrate the existence of abundant defects, which could be originated from the intercalation of Li ions. The TEM images of MoS2 are shown in Fig. S2.† It can be seen that the interlayer spacing of (002) plane for MoS2 is 0.64 nm. The high-angle annular dark-field scanning TEM (HAADF-STEM) image and the corresponding element mapping images in Fig. 2c confirm the homogenous distribution of Mo and S elements in L-MoS2.
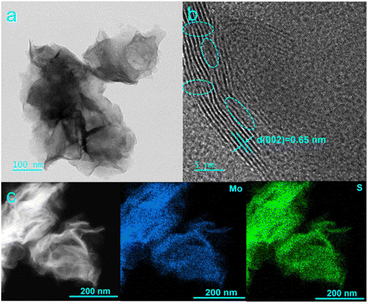 |
| Fig. 2 (a) TEM, (b) HRTEM, (c) HAADF-STEM and corresponding element mapping images of L-MoS2 nanoflowers. | |
The crystallinity of L-MoS2 and MoS2 was analyzed by X-ray diffractometer (XRD). In Fig. 3a, L-MoS2 and MoS2 display identical diffraction peaks around 2θ of 13.5°, 32.5°, 35.7° and 57.4°, which can be respectively corresponded to the (002), (100), (102) and (110) planes of 2H-MoS2 (JCPDS no. 37-1492),19 indicating that the intercalation of solvated Li ions has no distinct effect on the crystallinity of MoS2. According to the Bragg equation, the (002) plane interlayer spacing of L-MoS2 and MoS2 is respectively calculated to be 6.48 and 6.34 Å, which are in line with the HRTEM results. In addition, the XRD patterns in Fig. S3† reveal that the (002) plane interlayer spacing of L-MoS2-2.5 and L-MoS2-7.5 is calculated to be 6.42 and 6.53 Å, respectively, further indicating that the intercalation of MoS2 with Li2SO4 could broaden the interlayer spacing of MoS2. The large interlayer spacing of MoS2 can afford rapid electrolyte ions transportation in MoS2, contributing to improve the rate capability to a certain extent.
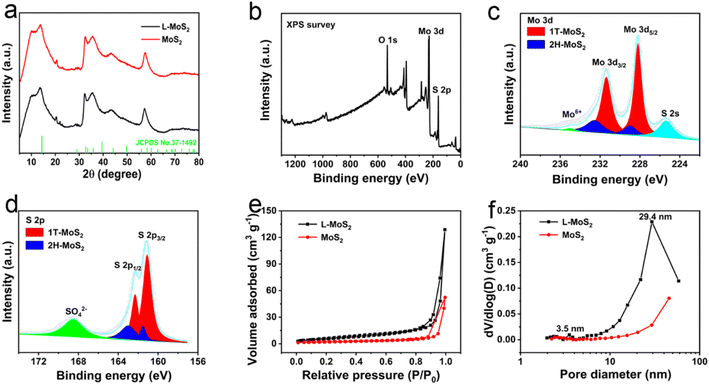 |
| Fig. 3 (a) XRD patterns of L-MoS2 and MoS2. (b) The XPS survey spectrum of L-MoS2. The high-resolution (c) Mo 3d, and (d) S 2p XPS spectrum of L-MoS2. (e) N2 adsorption–desorption isotherm curves of L-MoS2 and MoS2. (f) Pore size distribution curves of L-MoS2 and MoS2. | |
X-ray photoelectron spectroscopy (XPS) tests were carried out to identify the chemical states of the samples. The presence of O, Mo, and S elements in L-MoS2 is demonstrated by the XPS survey spectrum in Fig. 3b. Moreover, the trace amount of Li element in L-MoS2 can be identified by the high-resolution Li 1s XPS spectrum illustrated in Fig. S4.† The high-resolution Mo 3d spectrum in Fig. 3c displays two peaks at 229.1 eV and 232.7 eV referring to the Mo4+ 3d5/2 and Mo4+ 3d3/2 of 2H-MoS2, while the two peaks located at 228.4 eV and 231.6 eV corresponding to the Mo4+ 3d5/2 and Mo4+ 3d3/2 of 1T-MoS2.20 The weak peak at 235.1 eV demonstrates the presence of Mo6+ state, which is likely associated with the oxidation of MoS2.21 The S 2s peak at 225.6 eV is related to the Mo–S bond.22 Based on the integral area of the deconvoluted Mo 3d spectrum, the content of 1T phase in L-MoS2 is calculated to be 77.5%. The high-resolution S 2p spectrum in Fig. 3d reveals two peaks at 161.5 eV and 163.0 eV respectively assigns to S 2p3/2 and S 2p1/2 of 2H-MoS2, while the peak located at 161.1 eV and 162.3 eV could be respectively referred to S 2p3/2 and S 2p1/2 of 1T-MoS2.20,23 The strong SO42− peak at 168.5 eV could be possibly attributed to oxidation of MoS2.24 In addition, the two peaks of O 1s spectrum for L-MoS2 at 531.5 and 532.8 eV shown in Fig. S4† could be respectively associated with O–Mo bond and adsorbed water.25 The high-resolution Mo 3d and S 2p spectra of MoS2 are illustrated in Fig. S4† and the content of 1T phase in MoS2 is calculated to be 67.5%. It can be concluded that the intercalation of MoS2 with solvated Li ions is conducive to increasing the content of 1T phase in MoS2, which could be originated from that the negative charges on MoS2 nanosheets lead to lowered formation energy of 1T phase.18
The porous architecture of L-MoS2 and MoS2 was investigated by N2 absorption/desorption tests. In Fig. 3e, L-MoS2 and MoS2 exhibit a type-IV isotherm with an obvious hysteresis loop at high relative pressures, verifying the existence of mesopores.26 The specific surface area of L-MoS2 and MoS2 is calculated to be 23.7 and 7.5 m2 g−1, respectively. Compared with MoS2, the pore size distribution curve of L-MoS2 in Fig. 3f exhibits a bimodal pore size distribution. The narrow distribution centered at 3.5 nm might be related to the pores on the MoS2 nanosheets, whereas the wide distribution centered at 29.4 nm could be ascribed to the void space formed by the self-assemble of MoS2 nanosheets.6 Therefore, the large specific surface area and bimodal pore size distribution are beneficial to increasing the contact area with electrolyte ions and affording fast ions transportation, and then ameliorating the specific capacitance and rate capability of L-MoS2.20,27
2.2. Electrochemical performance
The electrochemical performances of L-MoS2 and MoS2 as supercapacitor electrodes were firstly studied in three-electrode system using 2 M KOH as electrolyte. In this work, nickel foam was used as a current collector due to its high conductivity and porosity. After applying L-MoS2 powder and MoS2 powder to nickel foam, the conductivity of obtained L-MoS2 and MoS2 electrode is 0.96 kS cm−1 and 0.82 kS cm−1, respectively. The cyclic voltammetry (CV) and galvanostatic charge–discharge (GCD) curves of nickel foam alone are illustrated in Fig. S5.† According to the CV and GCD test results, the contribution of foam nickel to capacitance could be negligible. The CV curves of L-MoS2 and MoS2 are compared at a scan rate of 20 mV s−1, as present in Fig. 4a. It can be observed that both L-MoS2 and MoS2 reveal a anodic peak at 0.52 V and cathodic peak at 0.28 V, manifesting the pseudocapacitive nature of L-MoS2 and MoS2.28 Moreover, L-MoS2 exhibits a larger integral area of CV curve than that of MoS2 at the same scan rate, signifying the higher specific capacitance of L-MoS2. Fig. 4b shows the CV curves of L-MoS2 at different scan rates. Obviously, all the CV curves display a pair of distinct redox peaks and the anodic peaks and cathodic peaks gradually move away mutually as the scan rate increases, which may be associated with the polarization phenomenon and internal resistance in L-MoS2 electrode.29 The GCD curves of L-MoS2 and MoS2 at a current density of 1 A g−1 are compared in Fig. 4c. The L-MoS2 displays longer discharge time than that of MoS2, further verifying the higher specific capacitance of L-MoS2 after intercalating moderate amount of Li2SO4. All GCD curves in Fig. 4d are nearly symmetric, indicating that L-MoS2 possesses a highly electrochemical reversibility.30 The CV and GCD curves of MoS2 reveal similar variation tendency with L-MoS2, as illustrated in Fig. S6.† The specific capacitances of L-MoS2 and MoS2 calculated from the GCD curves are provided in Fig. 4e. It can be found that all the specific capacitances decrease with increasing the current density, which might be related to the limitation of K ions transportation at high current density.31 At a current density of 1 A g−1, L-MoS2 achieves a specific capacitance of 356.7 F g−1, which is larger than that of MoS2 (282.8 F g−1). When the current density is 20 A g−1, L-MoS2 still maintains a specific capacitance of 177.7 F g−1, exhibiting a favorable rate capability with 49.8% of capacitance retention, which is better than that of MoS2 (35.6%). In addition, the intercalation amount of Li2SO4 has a significant effect on the electrochemical properties of L-MoS2. As present in Fig. S7,† all the specific capacitances of L-MoS2-2.5 and L-MoS2-7.5 are inferior than that of L-MoS2 under the same current density, which could be associated with the morphology and interlayer spacing of L-MoS2. Compared with L-MoS2, L-MoS2-2.5 exhibits smaller layer spacing with obvious stacking/agglomeration, which is adverse for the transportation of electrolyte ions into L-MoS2-2.5, resulting in lower specific capacitance than L-MoS2. Although the interlayer spacing of L-MoS2-7.5 is greater than that of L-MoS2, the local collapse structure of L-MoS2-7.5 and excessive Li ions between the L-MoS2-7.5 bilayers can hinder the rapid transportation of electrolyte ions within it, leading to significantly lower specific capacitance than L-MoS2. Electrochemical impedance spectroscopy (EIS) measurements were conducted to study the kinetics of L-MoS2 and MoS2. In Nyquist plot, the intercept value on the horizontal axis at high-frequency region corresponds to the equivalent series resistance (Rs) and the diameter of the semicircle represents the charge transfer resistance (Rct).32,33 In Fig. 4f, the Rs value of L-MoS2 (0.73 Ω) is less than that of MoS2 (0.85 Ω), implying that the intercalation of solvated Li ions contributes to the transportation of electrolyte ions in L-MoS2. Moreover, no obvious semicircle can be found in Nyquist plots, indicating that Rct is negligible and there is a fast electronic transfer during the redox reactions.34 The cycling stability tests of L-MoS2 and MoS2 were carried out at a current density of 10 A g−1. As present in Fig. S8,† L-MoS2 maintains 76.5% of capacitance retention after 3000 cycles, which is higher than that of MoS2 (48.8%), demonstrating a good cycling stability. The SEM images of L-MoS2 electrode and MoS2 electrode after 3000 cycles are illustrated in Fig. S9.† The MoS2 electrode reveals a dense structure and appears several cracks. Moreover, MoS2 losses its nanosheet structure and completely turns into nanoparticles after repeated charge/discharge cycles. In comparison, L-MoS2 electrode still exhibits a loose and porous architecture after cycling, which is conducive to generating a good cycling stability. We summarize the electrochemical performances (specific capacitance, rate capability and cycling stability) of MoS2-based electrodes in literature reports with our work. As shown in Table S1,† the electrochemical properties of L-MoS2 are comparable to the previously reported literature.
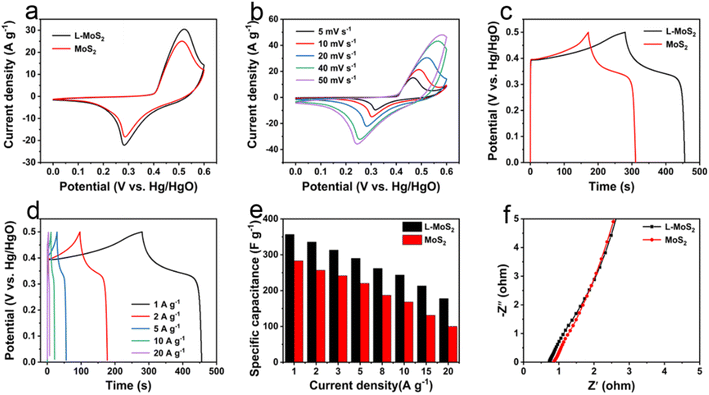 |
| Fig. 4 Electrochemical performances of L-MoS2 and MoS2 electrodes in three-electrode system. (a) Comparative CV curves at a scan rate of 20 mV s−1. (b) CV curves of L-MoS2 electrode at different scan rates. (c) Comparative GCD curves at a current density of 1 A g−1. (d) GCD curves of L-MoS2 electrode at different current densities. (e) Specific capacitance versus current density curves. (f) Nyquist plots. | |
Compared with MoS2, L-MoS2 displays superior specific capacitance, rate capability and cycling stability, which could be attributed to the following aspects. First, the self-assembled L-MoS2 exhibits nanoflowers-like architecture with a large specific surface area of 23.7 m2 g−1, which can expose more electroactive sites and increase the contact area with electrolyte ions. Second, L-MoS2 possesses an interlayer spacing of 0.65 nm, contributing to rapid transportation of electrolyte ions between L-MoS2 bilayers. Finally, the high 1T phase content (77.5%) in L-MoS2 is conducive to improving the conductivity and hydrophilicity, offering fast electronic transfer and large contact area with electrolyte ions.
In general, there are two charge storage mechanisms for MoS2 electrode in KOH electrolyte, one is a diffusion-controlled process for intercalation/deintercalation of K+ into/from the MoS2 interlayers during the faradaic reaction, the other one is a capacitive process based on the surface adsorption/desorption of K+ at the MoS2 electrode/electrolyte interface, as shown in eqn (1) and (2):9,27
|
MoS2 + K+ + e− ↔ MoS-SK+
| (1) |
|
(MoS2)surface + K+ + e− ↔ (MoS2-K+)surface
| (2) |
The CV curves of L-MoS2 and MoS2 were analyzed by virtue of the method described in the ESI.† The calculated results shown in Fig. S10† manifest that the charge storage in L-MoS2 comprises both diffusion-controlled process and capacitive process.35 At low scan rate of 5 mV s−1, the capacitive charge storage contribution ratio of L-MoS2 is 39.0%, which is higher than that of MoS2 (34.7%). Moreover, all the capacitive charge storage contribution ratios of L-MoS2 are larger than MoS2 at the same scan rate, further verifying the superiority of L-MoS2. At 50 mV s−1, the capacitive charge storage contribution ratio of L-MoS2 accounts for 86.2%. Such a high capacitive charge storage contribution ratio is in favor of obtaining superior rate capability.36
To further evaluate the practical application of L-MoS2 in supercapacitor, the SSC device was fabricated using two identical L-MoS2 electrodes in 2 M KOH electrolyte. The CV curves of the SSC device in Fig. 5a reveal quasi-rectangular shape and keep the shape unchanged even at the high scan rate of 100 mV s−1, demonstrating the superior capacitive behavior of the SSC device.12 The GCD curves of the SSC device are shown in Fig. 5b. The quasi-triangular characteristic of GCD curves confirms a highly electrochemical reversibility of the ASC device. The specific capacitance of the SSC device is calculated from the GCD curves and illustrated in Fig. 5c. The SSC device could deliver a specific capacitance of 47.3 F g−1 at 0.8 A g−1 and 13.0 F g−1 at 5 A g−1. Fig. 5d depicts the Nyquist plot of the SSC device, which displays a distinct semicircle at high frequency region. According to the equivalent circuit model shown in inset of Fig. 5d, the SSC device possesses a small Rs of 0.48 Ω and Rct of 0.81 Ω, manifesting a low resistance and rapid charge transfer in the SSC device.37 In Fig. 5e, the SSC device reveals 79.6% of capacitance retention and over 94% of coulombic efficiency after 3000 cycles at 2 A g−1. The low coulombic efficiency could be due to the side reaction originated from the creation of transition metal and sulfur-containing nanocomposites with low reversibility.19 The Ragone plot of the SSC device is shown in Fig. 5f. The SSC device exhibits a maximum energy density of 6.5 W h kg−1 at a power density of 413 W kg−1, and keeps 1.8 W h kg−1 even at 3.2 kW kg−1. The energy density and power density of L-MoS2 SSC device are comparable to MoS2-based SSCs in previously reported literature, such as 1T-MoS2/Ti3C2 MXene (6.95 W h kg−1 at 239.48 W kg−1),16 MoS2 nanosheets (6.56 W h kg−1 at 250 W kg−1),10 Cu doped MoS2 (5.58 W h kg−1 at 299 W kg−1),38 and MoS2–Ti3C2Tx (5.1 W h kg−1 at 298 W kg−1).19 If the masses of nickel foam collector and polypropylene diaphragm are added, the actual energy of the SSC device is only 0.028 W h kg−1 at a power density of 1.8 W kg−1. Therefore, MoS2-based free-standing electrodes need to be developed to enhance the actual energy of the SSC device. Self-discharge and leakage current are extremely important parameters to evaluate the practical application of supercapacitors. The self-discharge and leakage current curves of the SSC device are illustrated in Fig. S11.† As shown in Fig. S11a,† the self-discharge of the SSC device could be ascribed to the following aspects. (i) The internal resistance (IR) drop that appears at the initial stage of self-discharge, which is associated with the charging current density and the electrolyte type.39 (ii) The irreversible parasitic faradaic reaction could be originated from the surface oxygen-containing functional groups of L-MoS2 electrode.40 (iii) The charge redistribution caused by the inequality of charge distribution between the surface and inside of L-MoS2 electrode.41 According to the previously reported literature,42 the studied self-discharge time of the SSC device is 10 h. The self-discharge rate could be measured by the open circuit voltage attenuation per unit time (OCV) and the OCV of the SSC device is 63.7 mV h−1. In Fig. S11b,† as the current is stabilized, the leakage current of the SSC device is 46.5 μA (8.5 mA g−1). If we do not consider the internal short circuit that may occur in assembling the SSC device, the leakage current of the SSC device is mainly derived from the parasitic Faraday reaction near the interfaces of L-MoS2 electrode and electrolyte caused by the surface oxygen-containing functional groups.42
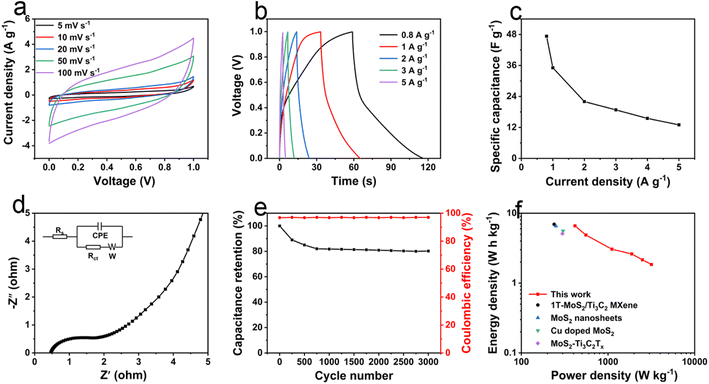 |
| Fig. 5 Electrochemical performances of L-MoS2 SSC in two-electrode system. (a) CV curves at different scan rates. (b) GCD curves under various current densities. (c) Specific capacitance versus current density curves. (d) Nyquist plot. Inset: the equivalent circuit model. (e) Cycling stability and coulombic efficiency after 3000 cycles at a current density of 2 A g−1. (f) Ragone plot. | |
3. Conclusions
In summary, L-MoS2 nanoflowers with an interlayer spacing of 0.65 nm are successfully self-assembled from MoS2 nanosheets under appropriate intercalation of Li2SO4. Due to the large specific surface area and high content of 1T-MoS2, L-MoS2 electrode delivers a maximum specific capacitance of 356.7 F g−1 at 1 A g−1 and keeps 49.8% of capacitance retention at 20 A g−1. Moreover, the fabricated L-MoS2 SSC device displays a highest energy density of 6.5 W h kg−1 and 79.6% of capacitance retention after 3000 cycles. It is believed that the intercalation of solvated Li ions could also be applicable to develop other 2D materials as advanced electrodes for supercapacitors.
Author contributions
Yunan Li: conceptualization, data curation, writing – original draft, writing – review & editing. Yang Sun: investigation, data curation. Sen Zhang: investigation, data curation. Xueling Wu: data curation. Meng Song: project administration. Mingli Jiao: funding acquisition. Qi Qin: writing – review & editing. Liwei Mi: funding acquisition, writing – review & editing.
Conflicts of interest
There are no conflicts to declare.
Acknowledgements
This work was supported by the National Natural Science Foundation of China (No. 21671205), the Program for Innovative Research Team (in Science and Technology) in University of Henan Province (No. 23IRTSTHN019), the Training Program for Young Backbone Teachers in Henan Colleges and Universities (No. 2021GGJS110), the Natural Science Foundation of Zhongyuan University of Technology (No. K2022MS005) and the Strength Enhancement Plan of Superior Disciplines of Zhongyuan University of Technology (No. SD202203). The authors would like to thank Gang Li and Jiaxiu Zhou from Shiyanjia Lab (https://www.shiyanjia.com/) for the SEM and TEM tests.
References
- R. Hu, Y. Liao, H. Qiao, J. Li, K. Wang, Z. Huang and X. Qi, Ceram. Int., 2022, 48, 23498–23503 CrossRef CAS.
- S. W. Bokhari, A. V. Ellis, M. Uceda, S. Wei, M. Pope, S. Zhu, W. Gao and P. C. Sherrell, J. Energy Storage, 2022, 56, 105935 CrossRef.
- W. Jia, H. Wu, Y. Zheng, Z. Liu, G. Cai, J. Wen, G. Hu, T. Tang, X. Li, L. Jiang, Z. Wang, M. Li and H. Huang, ACS Appl. Energy Mater., 2023, 6, 2570–2581 CrossRef CAS.
- S. Li, C. Huang, L. Gao, Q. Shen, P. Li, X. Qu, L. Jiao and Y. Liu, Angew. Chem., Int. Ed., 2022, 61, e202211478 CrossRef CAS PubMed.
- J. Tian, C. Yang, R. Hao, F. Li, Z. Liu, W. Chen, Y. Lv and C. Lin, Int. J. Hydrogen Energy, 2022, 47, 17871–17878 CrossRef CAS.
- X. Zhu, F. Xia, D. Liu, X. Xiang, J. Wu, J. Lei, J. Li, D. Qu and J. Liu, Adv. Funct. Mater., 2023, 33, 2207548 CrossRef CAS.
- M. K. Francis, K. Rajesh, P. B. Bhargav and N. Ahmed, J. Mater. Sci., 2023, 58, 4054–4069 CrossRef CAS.
- Z. Deng, H. Jiang and C. Li, Small, 2018, 14, 1800148 CrossRef PubMed.
- M. G. Fayed, S. Y. Attia, Y. F. Barakat, E. E. El-Shereafy, M. M. Rashad and S. G. Mohamed, Sustainable Mater. Technol., 2021, 29, e00306 CrossRef CAS.
- D. Kesavan, V. K. Mariappan, P. Pazhamalai, K. Krishnamoorthy and S. J. Kim, J. Colloid Interface Sci., 2021, 584, 714–722 CrossRef CAS PubMed.
- S. Wei, R. Zhou and G. Wang, ACS Omega, 2019, 4, 15780–15788 CrossRef CAS PubMed.
- J. Wang, X. Zheng, Y. Dong, L. Chen, L. Chen and W. He, Dalton Trans., 2023, 52, 4537–4547 RSC.
- Y. Cai, H. Kang, F. Jiang, L. Xu, Y. He, J. Xu, X. Duan, W. Zhou, X. Lu and Q. Xu, Appl. Surf. Sci., 2021, 546, 149088 CrossRef CAS.
- Z. Lei, J. Zhan, L. Tang, Y. Zhang and Y. Wang, Adv. Energy Mater., 2018, 8, 1703482 CrossRef.
- F. Wan, X. Wang, C. Tang, C. Jiang, W. Wang, B. Li, Y. Zhang and X. Zhu, J. Mater. Chem. A, 2022, 10, 12258–12268 RSC.
- X. Wang, H. Li, H. Li, S. Lin, W. Ding, X. Zhu, Z. Sheng, H. Wang, X. Zhu and Y. Sun, Adv. Funct. Mater., 2020, 30, 0190302 CrossRef CAS.
- H. Li, H. Li, Z. Wu, L. Zhu, C. Li, S. Lin, X. Zhu and Y. Sun, J. Mater. Sci. Technol., 2022, 123, 34–40 CrossRef CAS.
- Z. Bo, X. Cheng, H. Yang, X. Guo, J. Yan, K. Cen, Z. Han and L. Dai, Adv. Energy Mater., 2022, 12, 2103394 CrossRef CAS.
- W. Hou, Y. Sun, Y. Zhang, T. Wang, L. Wu, Y. Du and W. Zhong, J. Alloys Compd., 2021, 859, 157797 CrossRef CAS.
- H. Niu, Z. Zou, Q. Wang, K. Zhu, K. Ye, G. Wang, D. Cao and J. Yan, Chem. Eng. J., 2020, 399, 125672 CrossRef CAS.
- Z. Wang, J. Wang, F. Wang, X. Zhang, X. He, S. Liu, Z. Zhang, Z. Zhang and X. Wang, J. Alloys Compd., 2023, 947, 169505 CrossRef CAS.
- P. Kour, Deeksha and K. Yadav, J. Alloys Compd., 2022, 922, 166194 CrossRef CAS.
- S. Li, Y. Liu, X. Zhao, K. Cui, Q. Shen, P. Li, X. Qu and L. Jiao, Angew. Chem., Int. Ed., 2021, 60, 20286–20293 CrossRef CAS PubMed.
- Q. Zhang, Y. Song, B. Chen, X. Hu, W. Peng, Y. Li, F. Zhang and X. Fan, Chem. Eng. J., 2023, 454, 140330 CrossRef CAS.
- X. Gu, L. Zhang, X. Ma, J. Wang, X. Shang, Z. Wang, M. Kandawa-Schulz, W. Song and Y. Wang, Ionics, 2022, 28, 2337–2347 CrossRef CAS.
- I. T. Bello, K. O. Otun, G. Nyongombe, O. Adedokun, G. L. Kabongo and M. S. Dhlamini, Int. J. Energy Res., 2022, 46, 8908–8918 CrossRef CAS.
- K. B. Pisal, B. M. Babar, S. H. Mujawar, S. S. Mali, C. K. Hong, S. D. Sartale and L. D. Kadam, Int. J. Energy Res., 2022, 46, 18312–18327 CrossRef CAS.
- N. Joseph, P. Muhammed Shafi and A. Chandra Bose, New J. Chem., 2018, 42, 12082–12090 RSC.
- Y. Yao, J. Wang, X. Ban, C. Chen, Q. Wang, K. Zhu, K. Ye, G. Wang, D. Cao and J. Yan, EcoMat, 2023, 5, e12287 CrossRef CAS.
- S. Liu, Y. Yin, M. Wu, K. S. Hui, K. N. Hui, C.-Y. Ouyang and S. C. Jun, Small, 2019, 15, 1803984 CrossRef PubMed.
- K. Le, X. Zhang, Q. Zhao, Y. Liu, P. Yi, S. Xu and W. Liu, ACS Appl. Mater. Interfaces, 2021, 13, 44427–44439 CrossRef CAS PubMed.
- M. Rashidi and F. Ghasemi, Electrochim. Acta, 2022, 435, 141379 CrossRef CAS.
- H. Wu, Z. Guo, M. Li, G. Hu, T. Tang, J. Wen, X. Li and H. Huang, Electrochim. Acta, 2021, 370, 137758 CrossRef CAS.
- S. Liu, Y. Yin, D. Ni, K. S. Hui, K. N. Hui, S. Lee, C.-Y. Ouyang and S. C. Jun, Energy Storage Mater., 2019, 19, 186–196 CrossRef.
- M. S. Javed, X. Zhang, S. Ali, A. Mateen, M. Idrees, M. Sajjad, S. Batool, A. Ahmad, M. Imran, T. Najam and W. Han, Nano Energy, 2022, 101, 107624 CrossRef CAS.
- Z. Tang, J. Dai, W. Wei, Z. Gao, Z. Liang, C. Wu, B. Zeng, Y. Xu, G. Chen, W. Luo, C. Yuan and L. Dai, Adv. Sci., 2022, 9, 2201685 CrossRef CAS PubMed.
- X. Sun, Y. a. Pang, S. Li, Y. Yu, X. Ding, L. Wang and Q. Zhang, Ceram. Int., 2022, 48, 21317–21326 CrossRef.
- D. Vikraman, S. Hussain, K. Karuppasamy, A. Kathalingam, E.-B. Jo, A. Sanmugam, J. Jung and H.-S. Kim, J. Alloys Compd., 2022, 893, 162271 CrossRef CAS.
- S. Pu, Z. Wang, Y. Xie, J. Fan, Z. Xu, Y. Wang, H. He, X. Zhang, W. Yang and H. Zhang, Adv. Funct. Mater., 2022, 33, 2208715 CrossRef.
- M. Haque, Q. Li, A. D. Smith, V. Kuzmenko, P. Rudquist, P. Lundgren and P. Enoksson, J. Power Sources, 2020, 453, 227897 CrossRef CAS.
- W. Shang, W. Yu, X. Xiao, Y. Ma, Y. He, Z. Zhao and P. Tan, Adv. Powder Mater., 2023, 2, 100075 CrossRef.
- W. Li, X. Yang, Z. Chen, T. Lv, X. Wang and J. Qiu, Carbon, 2022, 196, 136–145 CrossRef CAS.
|
This journal is © The Royal Society of Chemistry 2023 |
Click here to see how this site uses Cookies. View our privacy policy here.