DOI:
10.1039/D3RA04766K
(Paper)
RSC Adv., 2023,
13, 29931-29943
An amplified electrochemical sensor employing one-step synthesized nickel–copper–zinc ferrite/carboxymethyl cellulose/graphene oxide nanosheets composite for sensitive analysis of omeprazole†
Received
15th July 2023
, Accepted 3rd October 2023
First published on 18th October 2023
Abstract
In this work, a signal amplification strategy was designed by the fabrication of a highly sensitive and selective electrochemical sensor based on nickel–copper–zinc ferrite (Ni0.4Cu0.2Zn0.4Fe2O4)/carboxymethyl cellulose (CMC)/graphene oxide nanosheets (GONs) composite modified glassy carbon electrode (GCE) for determination of omeprazole (OMP). The one-step synthesized Ni0.4Cu0.2Zn0.4Fe2O4/CMC/GONs nanocomposite was characterized by scanning electron microscopy, energy-dispersive X-ray spectroscopy, transmission electron microscopy and X-ray diffraction techniques. Then, the Ni0.4Cu0.2Zn0.4Fe2O4/CMC/GONs/GCE was applied to study the electrochemical behavior of the OMP. Electrochemical data show that the Ni0.4Cu0.2Zn0.4Fe2O4/CMC/GONs/GCE exhibits superior electrocatalytic performance on the oxidation of OMP compared with bare GCE, GONs/GCE, CMC/GONs/GCE and MFe2O4/GCE (M = Cu, Ni and Zn including single, double and triple of metals) which can be attributed to the synergistic effects of the nanocomposite components, outstanding electrical properties of Ni0.4Cu0.2Zn0.4Fe2O4 and high conductivity of CMC/GONs as well as the further electron transport action of the nanocomposite. Under optimal conditions, the Ni0.4Cu0.2Zn0.4Fe2O4/CMC/GONs/GCE offers a high performance toward the electrodetermination of OMP with the wide linear-range responses (0.24–5 and 5–75 μM), lower detection limit (0.22 ± 0.05 μM), high sensitivity (1.1543 μA μM−1 cm−2), long-term signal stability and reproducibility (RSD = 2.54%). It should be noted that the Ni0.4Cu0.2Zn0.4Fe2O4/CMC/GONs/GCE sensor could also be used for determination of OMP in drug and biological samples, indicating its feasibility for real analysis.
1. Introduction
Omeprazole (OMP), {5-methoxy-2-[(4-methoxy-3,5-dimethylpyridin-2-yl) methanesulfinyl]-1H-benzimidazole}, a substituted benzimidazole compound, is one of the first proton pump inhibitors to reduce gastric acid secretion and the prevention and treatment of gastric, duodenal ulcers or gastric reflux, as well as the treatment of infections caused by Helicobacter pylori (H. pylori).1–3 Also, a triple treatment regimen with OMP, amoxicillin, and clarithromycin has also been widely used to eradicate H. pylori.4–6 In fact, OMP is a pro-drug that is converted to an active sulfenamide intermediate at low pHs that binds to the thiol groups of the gastric wall proton pump cell (H+/K+-ATPase).3,7,8 Due to the importance of measuring OMP as well as the widespread commercialization of OMP-containing drug formulations, a wide range of analytical techniques including spectrophotometric,7,9–11 electrophoretic,8,12 chromatographic13–17 and electroanalytical methods18–23 have been reported to its evaluation. Spectroscopic and chromatographic methods to measure the OMP often require complicated and tedious sample preparation, pretreatment processes, expensive materials, and advanced instruments.24 While, the electroanalytical methods (electrochemical sensors) are simple, fast, cheap, accurate and minimal sample preparation, as well as powerful and practical techniques for analyzing with high selectivity, sensitivity, reproducibility, quick response time, and cheap instrumentation.25 Electrochemical sensors performances are limited due to slow electron transfer kinetics and the high required overpotential for the redox reaction of analyte molecules or ions. To illuminate these problems, the electrode surface should be modified with suitable materials to increase its electroactivity, sensitivity, and selectivity.26 Nowadays, nanomaterials as electrode materials are the most suitable materials for electrode modifiers, which have great potential for developing new devices and designing sensors with unique capabilities.27 Nanomaterial-based electrochemical sensors have great potential for enhancing electrical, physical, and sensing performance, especially in terms of selectivity and sensitivity.28 Hence, significantly and extensive researches on the fabrication of functional electrode materials, along with numerous electrochemical methods, are driving the widespread application of electrochemical devices. Compared to other nanoparticles, magnetic nanoparticles with the general formula MFe2O4-type spinel nano ferrites (M = is a divalent cation such as Fe, Co, Cu, Ni, etc.), a group of inorganic biomaterials, that are the most well-known compounds in analytical biochemistry, medicine, biotechnology and separation methods.29 Due to their good biocompatibility, good electrocatalytic properties, low toxicity, and easy preparation, these nanoparticles have received more attention in the preparation of sensors and biosensors.30–32 Ferrites are the most promising compounds among magnetic oxide complexes, which have complexes of iron oxides with different crystalline structures. There are currently several types of ferrites including spinel's ferrites, hexagonal ferrites, garnets, and ortho ferrites. Among these ferrites, spinel ferrites with hydrophilic nature are important magnetic materials due to their outstanding magnetic and electrical properties.33–35 One of the most essential spinel ferrites that has a mixed spinel structure is the Ni–Cu–Zn nano-crystalline ferrite. The nano-crystalline Ni–Cu–Zn ferrite is employed in the construction of multilayer chip inductors, information storage, biosensors, etc36. Ni–Cu–Zn ferrite is a soft material that has low magnetic power, good magnetic properties, and high electrical resistance.37 Recently, much research has been conducted to explore the properties of Ni–Cu–Zn ferrite and develop its applications as a new nanocomposite.38–42
The use of carbon nanomaterial composites such as graphene oxide, reduced graphene oxide, carbon nanotubes composites in the recent years have attracted much attention in electrode modification processes due to their suitable properties such as high surface area, conductivity and stability. Also, decrease of the required overpotential of the electrochemical reactions is one of the catalytic properties of hybrids of carbon nanomaterials.43–46 On the other hand, the application of graphene oxide and graphene-based metal oxide nanocomposites have been widely reported that improved the electrochemical performance.47 Compared with reduced graphene oxide, graphene oxide/metal oxide hybrid nanocomposites have concerned plentiful attention due to the simple and accessible synthesis method and also the synergistic effect between graphene oxide and metal oxide provide distinctive properties that make them suitable composites for various applications (such as photocatalysts, chemical sensors and biosensors, bioimaging, energy storage and conversion devices, lithium-ion batteries, and fuel cells).48 In addition to these, magnetic nanoparticles are appropriate materials with graphene oxide hybridization for utilization in electrochemical sensors.49,50 As mentioned in above, the utilization of magnetic nanoparticles as electrode surface modifiers has an increasing trend due to the high charge transfer capacity that significantly increases the electron transfer between the analyte and the electrode and consequently improves the sensitivity of electrochemical sensors.51–55 Despite these advantages, the accumulation of graphene oxide and magnetic nanostructures reduces their efficiency.56–58 Therefore, to solve this problem, these materials must be dispersed in a porous substrate. Cellulose has all the ideal properties required for sensors and biosensors due to its properties such as inertia, stability and mechanical strength. Cellulose and its derivatives such as carboxymethyl cellulose (CMC) can be used both as membranes and components of nanocomposites. It can also be used as a substrate for stabilizing graphene oxide and ferrite nanoparticles (to prevent them from accumulating on the electrode surface in electrochemical sensors). Hence CMC could act as an excellent option for the upgrade of magnetic nanoparticles composites.59–65
As far as we know, the utilization of nickel–copper–zinc ferrite (Ni0.4Cu0.2Zn0.4Fe2O4) nanocomposite for the determination of OMP (drug) has not been reported in the literature. In this research, the Ni0.4Cu0.2Zn0.4Fe2O4/CMC/graphene oxide nanosheets (GONs) modified glassy carbon electrode (GCE) as an innovative electrochemical sensor, Ni0.4Cu0.2Zn0.4Fe2O4/CMC/GONs/GCE, was designed to the determination of OMP. The physicochemical characterization of Ni0.4Cu0.2Zn0.4Fe2O4/CMC/GONs was examined using spectroscopic and electrochemical techniques [such as scanning electron microscopy (SEM), transmission electron microscopy (TEM), energy-dispersive X-ray spectroscopy (EDX), X-ray diffraction (XRD) and electrochemical impedance spectroscopy (EIS)]. Wonderfully, active sites and synergistic effects (between GONs, CMC and Ni0.4Cu0.2Zn0.4Fe2O4) of the nanocomposite demonstrated excellently outstanding electrocatalytic performance toward the oxidation of OMP with high sensitivity, long-term stability, desirable selectivity, wide concentration range, and low detection limit. Interestingly, the present developed sensor was able to monitor the OMP concentration in the real samples (pharmaceutical and biological samples).
2. Experimental
2.1. Materials and apparatus
A brief description of chemicals and reagents is presented in the ESI.† Electrochemical measurements were performed by a conventional three-electrode glass cell with a modified glassy carbon electrode (diameter: 3 mm), platinum wire (Pt) and Ag/AgCl (3 M KCl), respectively as the working, auxiliary and reference electrodes in a computer-controlled electrochemical workstation [Autolab, Eco Chemie, Utrecht, the Netherlands and data processing software (GPES, software version 4.7)] at room temperature (20–25 °C). Morphological, surface composition and compositional information of the Ni0.4Cu0.2Zn0.4Fe2O4/CMC/GONs/GCE were investigated by scanning electron microscopy equipped with X-ray energy dispersive spectroscopy (Phenom prox) and transmission electron microscopy (Carl Ziess AG-Zeiss EM900). X-ray powder diffraction analysis (XRD; D8 Advance Brucker AXS, Karlsruhe, Germany, CuKα radiation, λ = 1.5406 Å) was utilized to characterize the crystal structure of the prepared nanocomposites.
2.2. One-step synthesis of the Ni0.4Cu0.2Zn0.4Fe2O4/CMC/GONs nanocomposite
An appropriate amount of CMC/GONs composite66 (ESI†) was dispersed in deionized water and sonicated in an ultrasonic bath and then stirred with a magnetic stirrer for 12 hours. Then appropriate amounts of NiCl2·6H2O, CuCl2·6H2O, ZnCl2, and FeCl3·6H2O are weighed according to the desired stoichiometry and dissolved in distilled water (as the subsection of 2.2) and added to the dispersed CMC/GONs and sonicated for 1 hour.67 Then the solution of potassium hydroxide 1 mol L−1 was transferred drop by drop to a three-necked flask (in an ultrasonic bath (40 W) at a rate of 10 ml min−1 at room temperature) and the pH of the solution was adjusted to 10.5 with ammonia solution.21,68,69 When the co-precipitation reaction was completed, the sample was kept in a fixed place for 48 hours. The contents of the tube were then centrifuged at 3900 r min−1 for 5 minutes and repeated three times. The resulting product was then washed three times with distilled water and dried at 70 °C in an oven for 16 hours. Finally, the product was calcined for 2 hours at 800 °C and then sintered for 2 hours at 900 °C. Other structures of ferrite including single and double metals (M = Ni, Cu, and Zn); NiFe2O4, CuFe2O4, ZnFe2O4 or Cu0.5Ni0.5Fe2O4, Cu0.5Zn0.5Fe2O4 and Ni0.5Zn0.5Fe2O4 were synthesized with the same process by selecting of desired metal salts.
2.3. Preparation of real samples
To evaluate the performance of the Ni0.4Cu0.2Zn0.4Fe2O4/CMC/GONs/GCE for the electrodetermination of OMP in pharmaceutical tablets and biological samples; their preparation sample procedures are given in detail in the ESI.† Differential pulse voltammetry (DPV) technique and standard addition method were used to determine the OMP in real samples [three times assay of each sample (n = 3)].
3. Results and discussion
3.1. Physicochemical characterization of the nanocomposite
Since the morphological and compositional properties of materials play important roles in their physical and chemical properties, the synthesized nanocomposites and modified electrodes were characterized in detail by suitable methods. Typical morphology of nanomaterials were characterized by SEM and obtained results illustrated in Fig. 1 with two magnifications: [A (low), B (high)] GONs, [C (low), D (high)] CMC/GONs, [E (low), F (high)] Ni0.4Cu0.2Zn0.4Fe2O4 and [G (low), H (high)] Ni0.4Cu0.2Zn0.4Fe2O4/CMC/GONs. The captured images of A and B exhibit a folding laminar structure with thin sheets for the GONs. The morphology of CMC/GONs (images C and D) is flat and uneven with partially wrinkled structures. Images E and F illustrated the surface morphology of the Ni0.4Cu0.2Zn0.4Fe2O4. According to these pictures, it is observed that Ni0.4Cu0.2Zn0.4Fe2O4 nanoparticles are spherical and the particle size distribution is uniform. Also, images G and H in Fig. 1, represents the obtained images for Ni0.4Cu0.2Zn0.4Fe2O4/CMC/GONs in two magnifications. As can be seen from these images, the accumulated particles are almost regular in shape and the particle size distribution is relatively uniform. Furthermore, the EDX spectrum of Ni0.4Cu0.2Zn0.4Fe2O4/CMC/GONs clearly reveals the presence of Cu, Ni, Zn, Fe, C, O, and N elements in the sample (Fig. 1 spectrum I) which signifies the successful synthesis of the Ni0.4Cu0.2Zn0.4Fe2O4/CMC/GONs nanocomposite. The elemental maps were also recorded to obtain further information about the element distribution of the present atoms. The elemental mapping of the Ni0.4Cu0.2Zn0.4Fe2O4/CMC/GONs was carried out by EDX analysis and the results were revealed in Fig. 2 which confirms the existence of Fe, Cu, Zn, Ni, C and O atoms with uniform distribution in the synthesized nanocomposite.
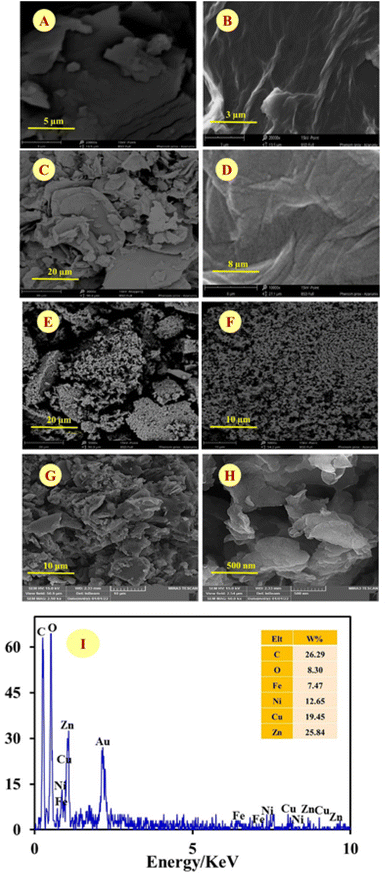 |
| Fig. 1 SEM images with two magnifications of synthesized materials: [A (low), B (high)] GONs, [C (low), D (high)] GONs/CMC, [E (low), F (high)] Ni0.4Cu0.2Zn0.4Fe2O4, [G (low), H (high)] Ni0.4Cu0.2Zn0.4Fe2O4/CMC/GONs and (I) the EDX spectrum of as-prepared Ni0.4Cu0.2Zn0.4Fe2O4/CMC/GONs. | |
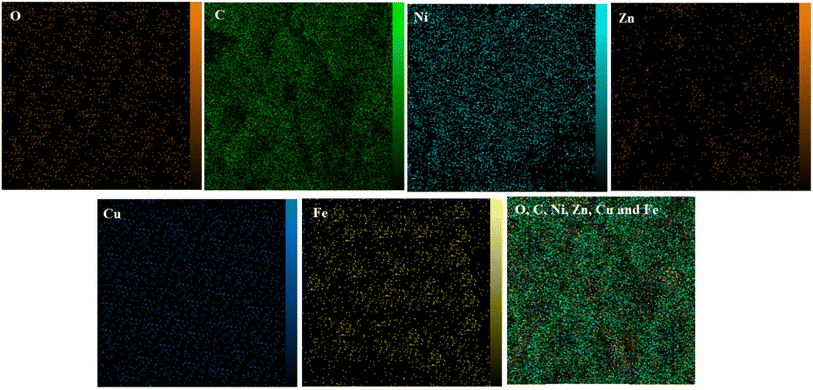 |
| Fig. 2 Elemental mapping of the each atom in the Ni0.4Cu0.2Zn0.4Fe2O4/CMC/GONs, last map is the distribution of all atoms. | |
Fig. 3 (left) displays the TEM image of the Ni0.4Cu0.2Zn0.4Fe2O4/CMC/GONs and reveals that particles are irregular, most likely cubic in shape, and agglomerated. As can be seen from Fig. 3, Ni0.4Cu0.2Zn0.4Fe2O4/CMC/GONs particle is polygonal, with a size of less than 50 nm, but there are also a few particles with larger size. It is considered that some particles grow abnormally due to incomplete drying process and small amount of water remaining, but most of the particles are normal in size, proving that the prepared ferrite powder is nanoparticle and nanocrystalline, Fig. 3 (right).
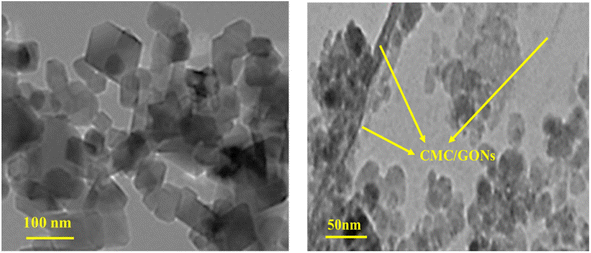 |
| Fig. 3 TEM images of the Ni0.4Cu0.2Zn0.4Fe2O4/CMC/GONs nanocomposite with different magnification [left (low) and right (high)]. | |
The XRD analysis was used to identify the crystal phase and structural information of freshly prepared GONs, CMC/GONs, Ni0.4Cu0.2Zn0.4Fe2O4, and Ni0.4Cu0.2Zn0.4Fe2O4/CMC/GONs nanocomposite (Fig. S1†). In the XRD pattern of GONs (pattern a), the peaks around 2θ equal to 11.09 and 26.4 for reflection (002) of GONs stacked with distances d equal to 6.76 Å and 3.39 Å, respectively, much larger than natural graphite (3.34 Å), which indicates the introduction of oxygen-containing groups on GONs.65 After loading the GONs with the CMC matrix66,67 (pattern b), the peak in the GONs almost disappeared on 2θ = 11.09, while other diffraction peaks were similar to the peaks of pure GONs.65 The Ni0.4Cu0.2Zn0.4Fe2O4 sample (pattern c) shows clear reflections, which can be assigned to (111), (220), (311), (222), (400), (422), (511), and (440) planes that emphasize the successful formation of Ni0.4Cu0.2Zn0.4Fe2O4 according to previous research;68,69 a single phase of Ni0.4Cu0.2Zn0.4Fe2O4 with cubic spinel structure.69–71 On the other hand, no extra lines corresponding to any other phases can be detected that indicates the resulting spinel ferrite is relatively pure. In addition, Fig. S1† pattern d depicted the XRD pattern of the Ni0.4Cu0.2Zn0.4Fe2O4/CMC/GONs. It can be seen that almost all diffraction peaks of Ni0.4Cu0.2Zn0.4Fe2O4 correspond to the standard pattern of Ni0.4Cu0.2Zn0.4Fe2O4 nanoparticles presented in the Ni0.4Cu0.2Zn0.4Fe2O4/CMC/GONs nanocomposite which indicates that the formation of nanocomposite and presence of CMC and GONs has no effect on the crystal structure of ferrite.72
Electrochemical characterization of the prepared modified electrode was followed as: electrochemical impedance spectroscopy (EIS) was used to evaluate the interface properties of the modified electrodes compared to the unmodified electrode towards a standard redox system; [Fe(CN)6]3−/[Fe(CN)6]4− (5.0 mM) in 0.1 M KCl solution. As shown in Fig. 4A (Nyquist plots), the charge transfer resistance (Rct) is estimated to be 309.83, 235.03, 192.45, 176.08, and 128.32 Ω.cm2 for GCE, GONs/GCE, CMC/GONs/GCE, Ni0.4Cu0.2Zn0.4Fe2O4/GCE and Ni0.4Cu0.2Zn0.4Fe2O4/CMC/GONs/GCE, respectively (as shown in Table 1). According to the results, it can be seen that the Ni0.4Cu0.2Zn0.4Fe2O4/CMC/GONs/GCE shows a smaller distinct semi-circular arc with a low Rct value compared to other step-by-step modified electrodes which indicates that the Ni0.4Cu0.2Zn0.4Fe2O4/CMC/GONs can improves the charge-transfer kinetics and implicates the excellent electrocatalytic activity of the designed nanocomposite. Furthermore, the inset of Fig. 4B displays the equivalent circuit model for Ni0.4Cu0.2Zn0.4Fe2O4/CMC/GONs/GCE, which is consistent with the results of the corresponding Nyquist plots and mirror electron shifting characteristics [Fe(CN)6]3−/[Fe(CN)6]4− redox probe.21
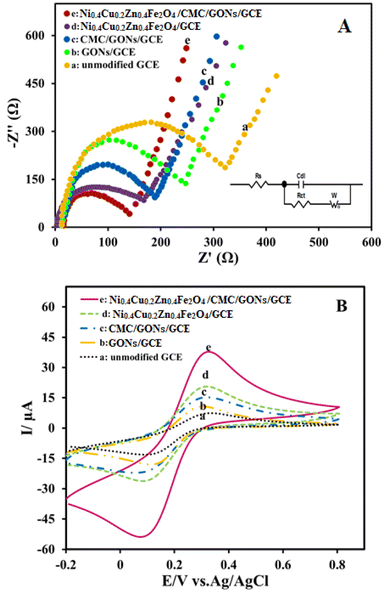 |
| Fig. 4 (A) Nyquist plots of the unmodified GCE (a), GONs/GCE (b), CMC/GONs/GCE (c), Ni0.4Cu0.2Zn0.4Fe2O4/GCE (d) and Ni0.4Cu0.2Zn0.4Fe2O4/CMC/GONs/GCE (e) in 0.1 M KCl containing 5 mM [Fe(CN)6]3−/[Fe(CN)6]4− as the redox probe. (B) CVs of the same electrodes in similar conditions. | |
Table 1 The Rct value of different electrodes
Electrode |
Rct |
Ni0.4Cu0.2Zn0.4Fe2O4/CMC/GONs/GCE |
128.32 |
Ni0.4Cu0.2Zn0.4Fe2O4/GCE |
176.08 |
CMC/GONs/GCE |
192.45 |
GONs/GCE |
235.03 |
GCE |
309.83 |
The electrochemical responses of the prepared electrodes in redox probe solution, Fe(CN)63−/Fe(CN)64− (5.0 mM) and KCl (0.1 M), were also recorded by the cyclic voltammetric method. Fig. 4B shows the obtained cyclic voltammograms for GCE (curve a), GONs/GCE (curve b), CMC/GONs/GCE (curve c), Ni0.4Cu0.2Zn0.4Fe2O4/GCE (curve d), and Ni0.4Cu0.2Zn0.4Fe2O4/CMC/GONs/GCE (curve e). From these results, the real surface area of the modified electrodes was determined based on the plots of the acodic peak current versus square root of scan rate (not shown here) and the Randles–Sevick formula (eqn (1)):73–76
|
Ip = 2.69 × 105n3/2AC0D1/2v1/2
| (1) |
In
eqn (1),
Ip,
n, A, C, D, and V are the anodic peak current, the number of electrons in the reaction (
n = 1), electrochemical real surface area, electroactive species concentration (5.0 mM), the diffusion coefficient of [Fe(CN)
6]
3−/[Fe(CN)
6]
4− (7.6 × 10
−6 cm
2 s
−1),
75,77 and the scan rate, respectively. Based on the calculation, the real surface areas were found as: 0.023, 0.048, 0.083, 0.284, and 0.686 cm
2 for GCE, GONs/GCE, CMC/GONs/GCE, Ni
0.4Cu
0.2Zn
0.4Fe
2O
4/GCE, and Ni
0.4Cu
0.2Zn
0.4Fe
2O
4/CMC/GONs/GCE, respectively.
3.2. Electrocatalysis performance of the Ni0.4Cu0.2Zn0.4Fe2O4/CMC/GONs/GCE towards OMP oxidation
In order to evaluate the electrocatalysis performance of the Ni0.4Cu0.2Zn0.4Fe2O4/CMC/GONs/GCE towards the oxidation of OMP, the cyclic voltammetric method was utilized in phosphate buffer solution (PBS, pH = 6.0) at the potential window from 300 to 1100 mV s−1 vs. Ag/AgCl in the presence of OMP (25 μM) with a fixed scan rate at 100 mVs −1. The obtained results in these condition at the unmodified GCE, GONs/GCE, CMC/GONs/GCE, Ni0.4Cu0.2Zn0.4Fe2O4/GCE and Ni0.4Cu0.2Zn0.4Fe2O4/CMC/GONs/GCE were shown in Fig. 5A. As shown, at the unmodified GCE (curve a), a very low redox current signal for OMP oxidation was obtained (Ip = 0.95 μA, Ep = 0.898 V). While others electrodes show high oxidation peaks: GONs/GCE (Ip = 4.15 μA, Ep = 0.844 V), CMC/GONs/GCE (Ip = 6.23 μA, Ep = 0.813 V), Ni0.4Cu0.2Zn0.4Fe2O4/GCE (Ip = 7.73 μA, Ep = 0.797 V), and Ni0.4Cu0.2Zn0.4Fe2O4/CMC/GONs/GCE (Ip = 11.24 μA, Ep = 0.787 V). As depicted in Fig. 5A, the Ni0.4Cu0.2Zn0.4Fe2O4/CMC/GONs/GCE (curve e) gives a well-defined peak for OMP oxidation with highest peak current and lower potential compared to all the modified GCEs.
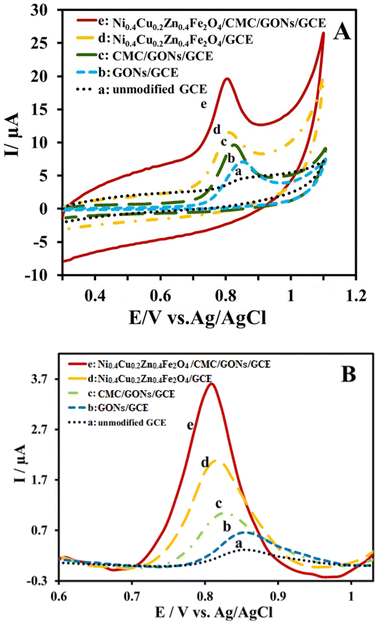 |
| Fig. 5 (A) CVs and (B) DPVs of the OMP at different electrodes: unmodified GCE (a), GONs/GCE (b), CMC/GONs/GCE (c), and Ni0.4Cu0.2Zn0.4Fe2O4/GCE (d), and Ni0.4Cu0.2Zn0.4Fe2O4/CMC/GONs/GCE (e). Cyclic voltammetric conditions: scan rate of 100 mVs−1 for 25 μM OMP in pH 6.0 PBS (0.1 M). DPV conditions: pulse amplitude 0.05 V, scan rate 0.025 V s−1 and pulse time 0.04 s for 4 μM OMP in pH 6.0 PBS phosphate buffer (0.1 M). | |
These results indicate that the electrooxidation process of OMP at the Ni0.4Cu0.2Zn0.4Fe2O4/CMC/GONs/GCE surface is significantly increases due to the simultaneous presence of the GONs, CMC and ferrite nanoparticles in the nanocomposite, which has good conductivity from that of GONs, high specific surface area from that of CMC and ferrite and synergistic contributions of CMC, GON and Ni0.4Cu0.2Zn0.4Fe2O4 which increase the electrocatalytic performances of the Ni0.4Cu0.2Zn0.4Fe2O4/CMC/GONs/GCE. On the other hand, high electrocatalysis activity of the Ni0.4Cu0.2Zn0.4Fe2O4/CMC/GONs/GCE may be attributable to the synergistic effects of these components, the effective interaction of Ni0.4Cu0.2Zn0.4Fe2O4 nanoparticles with OMP molecules78 on the electrode surface and the catalytic effect of the nanocomposite, Ni0.4Cu0.2Zn0.4Fe2O4/CMC/GONs/GCE (Fig. 3A curve a) in analogy with CMC/GONs/GCE (Fig. 5A curve c).21 Actually, the excellent increase in the anodic peak current and decrease in the peak potential of OMP oxidation implies that the modified Ni0.4Cu0.2Zn0.4Fe2O4/CMC/GONs/GCE accelerates the rate of electron transfer reaction. Finally, as can be seen, no cathodic peak is observed for OMP electroreaction during the reverse scan, it can be concluded that the electrochemical reaction of OMP at the Ni0.4Cu0.2Zn0.4Fe2O4/CMC/GONs/GCE is totally irreversible based on scientific findings.79
Also, the DPVs were recorded in 4 μM OMP on the surface of unmodified GCE, GONs/GCE, CMC/GONs/GCE, Ni0.4Cu0.2Zn0.4Fe2O4/GCE and Ni0.4Cu0.2Zn0.4Fe2O4/CMC/GONs/GCE and shown in Fig. 5B. As can be seen, at the surface of unmodified GCE, in the scanning potential window of 0.6 to 1.0 V, a very weak and broad anodic peak current (curve a) was observed for OMP oxidation due to slow electron transfer.21 In addition, the peak current of OMP electrooxidation improved at the GONs/GCE (curve b) and significantly increased at the surface of the CMC/GONs/GCE (curve c). The Ni0.4Cu0.2Zn0.4Fe2O4/GCE shows a noteworthy high current for OMP oxidation (curve d). While, at the Ni0.4Cu0.2Zn0.4Fe2O4/CMC/GONs/GCE (curve e) for OMP oxidation, a clear and sharp oxidation peak with the highest peak current is observed, which indicates an improvement in sensitivity in the determination process of OMP due to the excellent electronic conductivity of the nanocomposite and the high electroactive surface of the Ni0.4Cu0.2Zn0.4Fe2O4/CMC/GONs/GCE. As can be seen, the DPVs results are in good agreement with the results of the cyclic voltammetric method.
3.3. Optimization of various experimental factors
Some important and key factors on the reaction rate and signal intensity including the amount and chemical structure of modifier, effect of pH, scan rate and accumulation time in the electrocatalysis process were studied and optimized.80,81 Since the amount of modifier plays an essential role in OMP electrocatalysis, different amounts of modifier were examined by linear sweep voltammetry (LSV) method in the electrode modification process (0.5 mg ml−1 to 3 mg ml−1) (Fig. 6A). The results (inset of Fig. 6A) show that the modified electrode with 1 mg ml−1 of modifier provides a significant high current signal compared to higher modifier values, while a further increase of the modifier reduces peak current. This behavior may be attributed to the fact that amounts higher than 1 mg ml−1 cause roughness on the surface, resulting in flattened noses and reduced sensitivity. Hence, the amount of 1 mg ml−1 was chosen as the optimal value for subsequent experimental tests in OMP electrocatalysis.
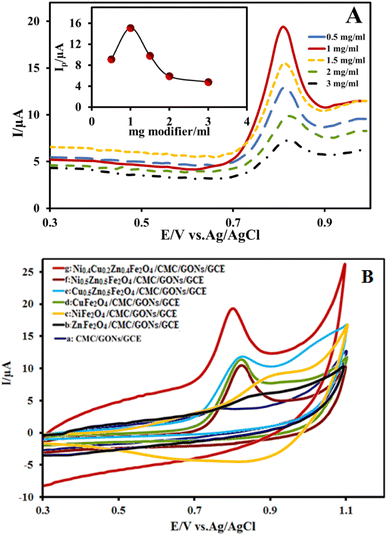 |
| Fig. 6 (A) LSVs of the Ni0.4Cu0.2Zn0.4Fe2O4/CMC/GONs/GCE at various amounts of modifier in PBS (0.1 M, pH 6.0) in a solution containing 25 μM OMP (inset is Ip vs. amount of modifier). (B) CVs of the OMP at different electrodes: CMC/GONs/GCE (a), ZnFe2O4/CMC/GONs/GCE (b), NiFe2O4/CMC/GONs/GCE (c), CuFe2O4/CMC/GONs/GCE (d) and Cu0.5Zn0.5Fe2O4/GCE (e), Ni0.5Zn0.5Fe2O4/GCE (f) and Ni0.5Cu0.5Fe2O4/CMC/GONs/GCE (e). Cyclic voltammetric conditions: scan rate of 100 mV s−1 for 25 μM OMP in pH 6.0 PBS (0.1 M). | |
The chemical structure of synthesized ferrite nanoparticles as a part of nanocomposite modifier can also affect the signal of OMP electrooxidation due to the synergistic contribution of metals. Therefore, in order to study of effect of each metal in the ferrite structure on the electrocatalysis activity, different structures of ferrite including single, double, and triple metals (M = Ni, Cu and Zn) involved in ferrite components were synthesized and used as modifier in the OMP oxidation. The obtained cyclic voltammograms related to different nanocomposite modifiers are shown in Fig. 6B. As seen before (Fig. 5 curve c), the voltammogram related to CMC/GONs/GCE shows a very weak electrocatalysis response to the OMP oxidation with lowest peak current value. Comparing this voltammogram with the voltammograms of Fig. 6B shows that by adding the ferrite nanoparticles to the CMC/GONs system, the peak current value of the OMP oxidation increases, which indicates its positive effect on the anodic peak current. The plot of ZnFe2O4/CMC/GONs/GCE gave approximately 2 times higher peak current values than the CMC/GONs/GCE at the same concentration of OMP. It was also observed that the modifier containing NiFe2O4/CMC/GONs/GCE showed approximately 3 times the peak current values, while the nanocomposite containing CuFe2O4/CMC/GONs/GCE showed approximately 4 times the peak current values. Also, the voltammograms of double ferrites; Cu0.5Zn0.5Fe2O4/CMC/GONs/GCE and Ni0.5Zn0.5Fe2O4/CMC/GONs/GCE were examined. Of these two the Cu0.5Zn0.5Fe2O4/CMC/GONs/GCE shows high electrocatalysis activity. Finally, the triple metal ferrites; Ni0.4Cu0.2Zn0.4Fe2O4, show the highest peak current in the OMP oxidation due to the synergistic contribution of metals.
Another effective parameter that must be optimized is the pH of the solution in the electrooxidation of OMP. For this purpose, the effect of pH on the oxidation of OMP at the Ni0.4Cu0.2Zn0.4Fe2O4/CMC/GONs/GCE was studied by CV technique for 10 ml 0.1 M PBS containing 25 μM OMP with different pH values in the range of 3.0 to 9.0 (Fig. S2†) at the scan rate of 100 mV s−1. The results illustrated that the pH value affects both the peak current and peak potential of OMP oxidation. As can be seen, the anodic peak current increased with the pH change (inset A) from 3.0 to 6.0 and then decreased (in pH from pH 7.0 to 9.0). Also, the oxidation peak potential of OMP shifts to a negative potential with increasing pH under a regression equation of Epa(V) = −0.0529 pH + 1.1388 (R2 = 0.9923). The peak potential diagram in terms of pH can be seen in inset B of Fig. S2.† As shown, the slope of the peak potential changes is 0.0529 V pH −1 (obtained as a function of the pH of the solution), which is close to the slope of the Nernst equation.21,82 Therefore, we can deduce that the number of electrons and protons involved in the oxidation reaction are equal. Based on the obtained results and according to the literature reported for OMP oxidation, the suggested electrooxidation mechanism of OMP can be expressed as Scheme S2,† in which the number of electrons and protons is 1.21,22,83,84 From the chemical point, OMP is a weak lipophilic base with pKa1 = 4.2 and pKa2 = 9 and decomposes unless protected against acidic conditions.85,86 Based on the values of pKa for OMP, at the pHs lower than the pKa1, due to the protonation of OMP molecule, its oxidation process may be more difficult and therefore the current should increase with increasing pH around its pKa1. At pH values above 9.0, the slope changes and the peak potential is independent of pH. Due to the propinquity of the intersection point of the curve to the pKa2 value of benzimidazole moiety present in the OMP molecule (about 8.6), it is assumed that the oxidation of OMP is related to the removal of a proton from the imidazole part of the OMP molecule in the oxidation of this molecule.87 This result is consistent with the electrochemical oxidation mechanism of OMP that shown in Scheme S2†.83,84 On the other hand, the electrochemical oxidation mechanism of OMP involves the removal of an electron and the formation of an intermediate cation radical. Then deprotonation occurs, after which the radical cation reacts with water molecules and finally leads to the production of an irreversible hydroxylated product.88,89 Due to the excellent response of the obtained sensor in buffer solution at pH = 6.0, OMP oxidation has the best and highest signal at pH = 6.0, this pH was used in subsequent experiments which is favorable for determining the OMP in real biological conditions.
To obtain more detailed information about the kinetic and the nature of the OMP electrooxidation process at the Ni0.4Cu0.2Zn0.4Fe2O4/CMC/GONs/GCE, the effect of the potential sweep rate by employing the several scan rates from 10–120 mVs−1 on the peak current response was evaluated by CV analysis in the 0.1 M PBS (pH = 6.0) and 25 μM of OMP (Fig. S3†). As can be seen from Fig. S3,† the analysis of anodic peak current in terms of scan rate (inset A) shows a linear relationship between peak current (IP) and scan rate (v) with linear equation; I (μA) = 0.9789 + 0.1134v (v in mV s−1), (R2 = 0.9903), which indicates that the electrode reaction process is an adsorption-controlled process.21,90 Also, by plotting the log peak current (Ip) in terms of the log of scan rate (inset B), a linear graph was obtained with a slope of 0.8919 [log
Ip (μA) = 0.8919
log
v (mV s−1) − 0.687 (R2 = 0.9955)]. The obtained value is theoretically close to 1.0 for reactions that are classified as adsorption-controlled processes.22
Furthermore, based on the theory of Laviron for an irreversible electrode process and the E-Log ν plot (Fig. S4†), and following equation (eqn (2)),91 the catalytic rate constant (ks) and the values of electron transfer coefficients (αn) of OMP electrooxidation were calculated at the Ni0.4Cu0.2Zn0.4Fe2O4/CMC/GONs/GCE.
|
 | (2) |
where
n,
F,
R,
T and other symbols have their conventional and usual meanings. By using the Laviron equation, the
αn and
Ks (catalytic constant) were calculated to be about 0.47 and 1.31 s
−1, respectively.
Optimization of accumulation time is very important in conditions where the proposed reaction mechanism is the adsorption process. Because, this factor can affect the amount of OMP accumulated on the modified electrode surface. Fig. S5† depicts the diagram of the anodic peak current in terms of the accumulation time for the 4 μM OMP solution under optimal conditions in the range of 10–70 s by DPV technique, where a linear relationship between anodic peak current and time was observed. The Ipa value increased rapidly with increasing pre-concentration time and reached an uppermost value at 50 s, while the peak current remained constant with a further increase in accumulation time. Therefore, in the following experiments, the optimal accumulation time was selected as 50 s.
3.4. Electrochemical determination of OMP
Due to various advantages; higher current sensitivity, lower background currents, better performance and more acceptable peak resolutions, the DPV technique was used to quantitate the OMP by the proposed electrode; Ni0.4Cu0.2Zn0.4Fe2O4/CMC/GONs/GCE. To achieve the working range of newly designed sensor; Ni0.4Cu0.2Zn0.4Fe2O4/CMC/GONs/GCE, the corresponding DPV profiles at different concentrations of OMP were recorded under optimized conditions (0.1 M PBS at pH = 6.0). Fig. 7 shows DPVs grams of the OMP in different concentration ranges.
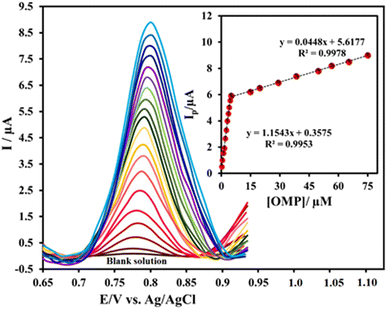 |
| Fig. 7 DPVs of Ni0.4Cu0.2Zn0.4Fe2O4/CMC/GONs/GCE in the absence (blank solution) and presence of 0.24–75 μM OMP in 0.1 M PBS phosphate buffer (pH = 6.0). Inset: the related linear relationships between the Ip and OMP concentrations (calibration curve) in the ranges of 0.24–5 and 5–75 μM. | |
The linear response were obtained for the OMP in the ranges of 0.24–5 μM and 5–75 μM with linear equations: Ip = 1.1543 [OMP] + 0.3575 and Ip = 0.0448 [OMP] + 5.6177 and correlation coefficient of R2 = 0.9953 and R2 = 0.9978 (inset), respectively. The calculated limit of detection (LOD) and limit of quantitation (LOQ) (LOD = 3S m−1 and LOQ = 10S m−1, where S = standard deviation and m = slope of the calibration curve)92 are 0.22 ± 0.05 μM and 0.73 ± 0.05 μM, respectively, suggesting that the proposed sensor can detect the low concentrations of OMP. In summary, the comparison of the results obtained from the present designed sensor with other OMP electrochemical sensors reported in the literature shows that the strategy applied in this study in terms of detection limit and dynamic range is well comparable with previous reports or better than the provided methods (as clearly shown in Table 2). The results show the high and acceptable performance of the present sensor for the determination of OMP by the DPV method.
Table 2 Comparison of analytical parameters of OMP determination at the Ni0.4Cu0.2Zn0.4Fe2O4/CMC/GONs/GCE with other modified electrodesa
Electrode |
Method |
Linear range (μM) |
LOD (μM) |
Sample |
Ref. |
LOD: detection of limit; DPV: differential pulse voltammetry; SWV: square wave voltammetry; DPP: differential pulse polarography; AP: amperometry; LSV: linear sweep voltammograms; GCE: glass carbon electrode; CPE: carbon paste electrode; BDDE: boron-doped diamond electrode; DME: dropping mercury electrode; EPG: edge-plane pyrolytic graphite electrode; NiOxNPs: nickel oxide nanoparticles. |
Mercury electrode |
DPV |
0.07–300 |
0.02 |
Drug, serum |
13 |
Ni0.5Zn0.5Fe2O4/Gr/GCE |
DPV |
0.02–1.4 |
0.13 |
Drug, serum |
22 |
BDDE |
DPV |
2.3–14 |
0.91 |
Urine, drug |
23 |
Mercapto-NP-F-CPE |
DPV |
0.00025–25.0 |
0.095 |
Urine, plasma, water, drug |
83 |
EPG |
SWV |
0.11–100 |
0.019 |
Drug, serum |
87 |
CuO NPs/CPE |
ALSV |
0.1–2 |
0.013 |
Drug, serum |
91 |
CPE |
SWV |
0.2–50 |
0.025 |
Drug |
93 |
GCE |
DPV |
2.9–58 |
0.55 |
Drug |
94 |
S-MWCNTs-Fe3O4/PPDA/GCE |
LSV |
0.05–9.0 |
0.015 |
Urine, plasma, water, drug |
95 |
NiOxNPs/GCE |
AP |
4.5–120 |
0.4 |
Drug |
96 |
Poly (alizarin)/GCE |
DPV |
400–1000 |
0.715 |
Drug |
97 |
DME |
DPP |
0.1–1 |
0.7 |
Drug, serum |
98 |
Chit-S@gC3N4/GCE |
DPV |
0.6–260 |
0.02 |
Drug, serum, urine |
99 |
Ni0.4Cu0.2Zn0.4Fe2O4/CMC/GONs/GCE |
DPV |
0.24–5 5–75 |
0.22 |
Drug, serum |
This work |
3.5. Interference studies
Anti-interference capability is one of the important indicators to evaluate the practical application of a sensor. In interference study, the interferer species are usually selected that have oxidation or reduction behavior near to the main analyte or presented in the analyte matrix (real samples). Therefore, the responses of the Ni0.4Cu0.2Zn0.4Fe2O4/CMC/GONs/GCE was investigated for the some selected interferences in determination of 4 μM OMP on behalf of common mineral ions (K+, Na+, Zn2+, Mg2+, Ca2+, Fe2+ and Cu2+) and also biological compounds (glucose, sucrose, ascorbic acid, citric acid, and uric acid) with a 10-folds concentrations higher than OMP under optimal experimental conditions (0.1 M PBS, pH = 6) and measured peak currents in DPV technique shown in Fig. S6.† According to the results, it is obvious that there is no noticeable and significant change in the OMP signal, which indicates that the proposed sensor is selective and suitable for determining OMP in complicated matrices.
3.6. Analytical applications of the Ni0.4Cu0.2Zn0.4Fe2O4/CMC/GONs/GCE in real samples
To evaluate the analytical and actual application of the newly designed sensor, OMP was determined in real samples (tablet and human serum) under optimal conditions by DPV technique, and the obtained results are listed in Table 3. The sample preparation of the real samples was described in the experimental section (ESI†). The obtained recovery range values of the human blood serum (98.80–100.50%) and tablet samples (98.24–100.31%) with low RSDs (2.88% for human blood serum and 2.65% for tablet samples) are acceptable. Therefore, it can be concluded that the proposed nanocomposite-modified electrode has significant potential that can be used to detect the OMP in real samples with a good recovery range.
Table 3 Results of recovery test of OMP in real samples (n = 3)
No. |
Added (μM) |
Found (μM) |
Recovery (%) |
RSD% |
Human serum |
0.00 |
Not detected |
— |
— |
2 |
2.01 |
100.50 |
2.88 |
5 |
4.98 |
99.60 |
3.15 |
10 |
9.88 |
98.80 |
3.36 |
Omeprazol tablet |
0.00 |
19.36 |
— |
3.05 |
2 |
21.16 |
99.06 |
3.42 |
3 |
22.43 |
100.31 |
2.65 |
4 |
22.95 |
98.24 |
3.13 |
4. Conclusion
This work summarizes the synthesis of Ni0.4Cu0.2Zn0.4Fe2O4/CMC/GONs nanocomposite through a one-step, sustainable and rapid method. The synthesized nanocomposite was characterized by different suitable techniques including SEM, TEM, XRD, EDX and EIS. In the next step, the GCE was modified by the obtained nanocomposite, Ni0.4Cu0.2Zn0.4Fe2O4/CMC/GONs/GCE, and then was used to the electrooxidation of OMP. The results show that the obtained modified electrode has excellent electrocatalytic activity for OMP oxidation in low positive potential (0.797 V) due to more active sites, large surface area, high conductivity, synergistic effects and fast electron transfer. The experimentally results display that the newly designed sensor has excellent analytical performance for OMP detection; wide working range (0.24–5 and 5–75 μM), low LOD (0.22 ± 0.05 μM) and LOQ (0.73 ± 0.05 μM), good sensitivity (1.1543 μA μM−1 cm−2), long-term stability, acceptable selectivity, and high reproducibility. The use of the designed sensor to determination of OMP in real samples such as human blood serum and OMP tablets demonstrates that its appreciable sensing potential in complex matrices. So, the prepared sensor could certainly be a promising candidate for a wide range of electrochemical and bioassay applications.
Conflicts of interest
There are no conflicts to declare.
Acknowledgements
The authors gratefully acknowledge the Research Council of Azarbaijan Shahid Madani University for financial support.
References
- K. Jana, T. Bandyopadhyay and B. Ganguly, Stereoselective Metabolism of omeprazole by cytochrome P450 2C19 and 3A4: Mechanistic Insights from DFT Study, J. Phys. Chem. B, 2018, 122(22), 5765–5775 CrossRef CAS PubMed.
- A. O. El-Nezhawy, A. R. Biuomy, F. S. Hassan, A. K. Ismaiel and H. A. Omar, Design, synthesis and pharmacological evaluation of omeprazole-like agents with anti-inflammatory activity, Bioorg. Med. Chem., 2013, 21(7), 1661–1670 CrossRef CAS PubMed.
- E. Cheng, Proton Pump Inhibitors for Eosinophilic Esophagitis, Curr. Opin. Gastroenterol., 2013, 29(4), 416–420 CrossRef CAS PubMed.
- D. Lopes-de-Campos, C. Pereira-Leite, P. Fontaine, A. Coutinho, M. Prieto, B. Sarmento, S. Jakobtorweihen, C. Nunes and S. Reis, Interface-Mediated Mechanism of Action-The Root of the Cytoprotective Effect of Immediate-Release Omeprazole, J. Med. Chem., 2021, 64(8), 5171–5184 CrossRef CAS PubMed.
- D. S. Strand, D. Kim and D. A. Peura, 25 years of proton pump inhibitors: a comprehensive review, Gut Liver, 2017, 11(1), 27–37 CrossRef CAS PubMed.
- D. Šahnić, E. Meštrović, T. Jednačak, I. Habinovec, J. Parlov Vuković and P. Novak, Monitoring and quantification of omeprazole synthesis reaction by in-line Raman spectroscopy and characterization of the reaction components, Org. Process Res. Dev., 2016, 20(12), 2092–2099 CrossRef.
- M. S. Elmasry, W. S. Hassan, M. Y. El-Mammli and M. Badrawy, Earth friendly spectrophotometric methods based on different manipulation approaches for simultaneous determination of aspirin and omeprazole in binary mixture and pharmaceutical dosage form: Comparative statistical study, Spectrochim. Acta, Part A, 2022, 266, 120436 CrossRef CAS PubMed.
- J. J. B. Nevado, G. C. Peñalvo, R. M. R. Dorado and V. R. Robledo, Simultaneous determination of omeprazole and their main metabolites in human urine samples by capillary electrophoresis using electrospray ionization-mass spectrometry detection, J. Pharm. Biomed. Anal., 2014, 92, 211–219 CrossRef CAS PubMed.
- H. M. Lotfy, M. A. Hegazy, S. Mowaka and E. H. Mohamed, Validated spectrophotometric methods for simultaneous determination of Omeprazole, Tinidazole and Doxycycline in their ternary mixture, Spectrochim. Acta, Part A, 2016, 153, 321–332 CrossRef CAS PubMed.
- M. S. Elmasry, A. Serag, W. S. Hassan, Ma. Y. El-Mammli and M. Badrawy, Spectrophotometric determination of aspirin and omeprazole in the presence of salicylic acid as a degradation product: a comparative evaluation of different univariate/multivariate post processing algorithms, J. AOAC Int., 2022, 105(1), 309–316 CrossRef PubMed.
- I. A. Alamin and A. A. Elbashir, A new study on Omeprazole spectrophotometric determination using 9- Fluorenyl methyl chloroformate as derivatizating agent, J. anal. pharm. res., 2019, 8(2), 38–43 CrossRef.
- G. Hancu, L. A. Papp and A. Rusu, Chiral Separation of the Enantiomers of Omeprazole and Pantoprazole by Capillary Electrophoresis, Chromatographia, 2015, 78, 279–284 CrossRef CAS.
- S. Beheshti, H. Ahmad Panahi and A. Feizbakhsh, Development of Thermo-Sensitive and Magnetic Molecularly Imprinted Polymer for Extraction of Omeprazole in Biological and Pharmaceutical Samples Coupled by High Performance Liquid Chromatography, ChemistrySelect, 2023, 8(10), e202203237 CrossRef CAS.
- M. Rambla-Alegre, J. Esteve-Romero and S. Carda-Broch, Analysis of omeprazole and its main metabolites by liquid chromatography using hybrid micellar mobile phases, Anal. Chim. Acta, 2009, 633(2), 250–256 CrossRef CAS PubMed.
- G. Yenduri and S. Navuluri, Analytical high performance liquid chromatography method for estimating the combination of aspirin and omeprazole in bulk and tablet dosage form, Marmara Pharm. J., 2018, 22(4), 502–510 CAS.
- E. Johansson and A. Karlsson, Jufang Wu Ludvigsson Ultra high performance liquid chromatography method development for separation of omeprazole and related substances on core-shell columns using a Quality by Design approach, J. Sep. Sci., 2020, 43(4), 696–707 CrossRef CAS PubMed.
- A. M. Kashid and O. H. Kolhe, Simultaneous Densitometric Determination of Aspirin and Omeprazole by High-Performance Thin-Layer Chromatography, J. Planar Chromatogr. - Mod. TLC, 2019, 32(6), 501–504 CrossRef CAS.
- B. Mostafiz, L. Fotouhi and P. Seyed Dorraji, An electrochemical sensor based on an Eriochrome Black T polymer and deep eutectic solvent for the simultaneous determination of omeprazole and lansoprazole, Anal. Methods, 2020, 12, 4072–4079 RSC.
- K. Pandian, J. Kalayarasi and S. C. Gopinath, Metal-free Sulfur-doped graphitic carbon nitride-modified GCE-based electrocatalyst for the enhanced electrochemical determination of omeprazole in Drug formulations and Biological Samples, Biotechnol. Appl. Biochem., 2022, 69, 2766–2779 CrossRef CAS PubMed.
- J. S. Stefano, T. F. Tormin, J. P. da Silva, E. M. Richter and R. A. Munoz, Amperometric determination of omeprazole on screen-printed electrodes using batch injection analysis, Microchem. J., 2017, 133, 398–403 CrossRef CAS.
- A. Afkhami, A. Bahiraei and T. Madrakian, Application of nickel zinc ferrite/graphene nanocomposite as a modifier for fabrication of a sensitive electrochemical sensor for determination of omeprazole in real samples, J. Colloid Interface Sci., 2017, 495, 1–8 CrossRef CAS PubMed.
- P. Karolia, D. Tiwari and R. Jain, Electrocatalytic sensing of omeprazole, Ionics, 2015, 21(8), 2355–2362 CrossRef CAS.
- Z. Chomisteková, E. Culková, R. Bellová, D. Melicherčíková, J. Durdiak, J. Timko, M. Rievaj and P. Tomčík, Oxidation and reduction of omeprazole on boron-doped diamond electrode: Mechanistic, kinetic and sensing performance studies, Sens. Actuators, B, 2017, 241, 1194–1202 CrossRef.
- E. I. El-Kimary and A. A. Marwa Ragab, Recent Analytical Methodologies for the Determination of Omeprazole and/or Its Active Isomer Esomeprazole in Different Matrices: A Critical Review, Crit. Rev. Anal. Chem., 2022, 52(1), 106–130 CrossRef CAS PubMed.
- W. Jiang, L. Huang, D. Zhang, Y. Wang and G. Pan, Graphene-Based Composites for Electrochemical Sensor Fabrication and Their Application for Drug Detection, Int. J. Electrochem. Sci., 2021, 16, 21037 CrossRef CAS.
- A. Afkhami, A. Bahiraei and T. Madrakian, Gold nanoparticle/multi-walled carbon nanotube modified glassy carbon electrode as a sensitive voltammetric sensor for the determination of diclofenac sodium, Mater. Sci. Eng. Carbon, 2016, 59, 168–176 CrossRef CAS PubMed.
- N. Baig, M. Sajid and T. A. Saleh, Recent trends in nanomaterial-modified electrodes for electroanalytical applications, TrAC, Trends Anal. Chem., 2019, 111, 47–61 CrossRef CAS.
- M. Ahmadi, A. Ghoorchian, K. Dashtian, M. Kamalabadi, T. Madrakian and A. Afkhami, Application of magnetic nanomaterials in electroanalytical methods: A review, Talanta, 2021, 225, 121974 CrossRef CAS PubMed.
- J. M. Gonçalves, L. V. de Faria, A. B. Nascimento, R. L. Germscheidt, S. Patra, L. P. Hernández-Saravia, J. A. Bonacin, R. A. A. Munoz and L. Angnes, Sensing performances of spinel ferrites MFe2O4 (M = Mg, Ni, Co, Mn, Cu and Zn) based electrochemical sensors: A review, Anal. Chim. Acta, 2022, 1233, 340362 CrossRef PubMed.
- P. T. K. Thu, N. D. Trinh and N. T. V. Hoan, et al., Synthesis of cobalt ferrite and simultaneous determination of ascorbic acid, acetaminophen and caffeine by voltammetric method using cobalt ferrite modified electrode, J. Mater. Sci.: Mater. Electron., 2019, 30, 17245–17261 CrossRef CAS.
- N. Q. Man, N. T. Thanh Tu, N. T. Vuong Hoan, H. X. Anh Vu, L. L. Son, N. D. Vu Quyen, D. N. Nhiem, N. H. Phong, V. T. Nguyen, T. N. Tuyen and D. Q. Khieu, Electrochemical determination of clenbuterol with nickel-ferrite/reduced-graphene-oxide-modified electrode, J. Nanopart. Res., 2023, 25, 31 CrossRef CAS.
- N. Tavakkoli, N. Soltani, F. Shahdost-fard, M. Ramezani, H. Salavati and M. R. Jalali, Simultaneous voltammetric sensing of acetaminophen, epinephrine and melatonin using a carbon paste electrode modified with zinc ferrite nanoparticles, Microchim. Acta, 2018, 185, 479–499 CrossRef PubMed.
- H. J. Kardile1, A. A. Pandit and K. M. Jadav, Hydrophilic nature of nickel ferrite thin film deposition by spray pyrolysis technique, Int. J. Res. Biosci. Agric. Techn., 2021, 17, 617–620 Search PubMed.
- A. Anosov, O. Koplak, E. Smirnova, E. Borisova, E. Korepanova and A. Derunets, Effect of Cobalt Ferrite Nanoparticles in a Hydrophilic Shell on the Conductance of Bilayer Lipid Membrane, Membranes, 2022, 12, 1106 CrossRef CAS PubMed.
- A. Vedrtnam, K. Kalauni, S. Dubey and A. Kumar, A comprehensive study on structure, properties, synthesis and characterization of ferrites, AIMS Mater. Sci., 2020, 7, 800–835 CAS.
- M. F. Huq, D. K. Saha, R. Ahmed and Z. H. Mahmood, Ni–Cu–Zn ferrite research: A brief review, J. Sci. Res., 2013, 5, 215–234 CrossRef CAS.
- M. Hashim, S. E. Shirsath, S. Kumar, R. Kumar, A. S. Roy, J. Shah and R. K. Kotnala, Preparation and characterization chemistry of nano-crystalline Ni–Cu–Zn ferrite, J. Alloys Compd., 2013, 549, 348–357 CrossRef CAS.
- A. D. Patil, R. A. Pawar, S. M. Patange, S. S. Jadhav, S. K. Gore, S. E. Shirsath and S. S. Meena, TiO2-doped Ni0.4Cu0.3Zn0.3Fe2O4 nanoparticles for enhanced structural and magnetic properties, ACS Omega, 2021, 6(28), 17931–17940 CrossRef CAS PubMed.
- M. N. Akhtar, M. A. Khan, M. Raza, M. Ahmad, G. Murtaza, R. Raza, S. Shaukat, M. Asif, M. Saleem and M. Nazir, Structural, morphological, dielectric and magnetic characterizations of Ni0.6Cu0.2Zn0.2Fe2O4 (NCZF/MWCNTs/PVDF) nanocomposites for multilayer chip inductor (MLCI) applications, Ceram. Int., 2014, 40(10), 15821–15829 CrossRef CAS.
- S. Zeki Bas, N. Yuncu, K. Atacan and M. Ozmen, A comparison study of MFe2O4 (M: Ni, Cu, Zn)-reduced graphene oxide nanocomposite for electrochemical detection of bisphenol A, Electrochim. Acta, 2021, 386, 138519 CrossRef.
- S. Sehrawat, M. Saini, A. Bhankhar and R. Shukla, A Comparative Analysis of Structural, Optical and Electrical Properties of Polyaniline/Ferrite (Co, Ni, Cu, Zn) Composites, ECS J. Solid State Sci. Technol., 2022, 11, 113005 CrossRef.
- J. Han, L. Sun, E. Cao, W. Hao, Y. Zhang and L. Ju, Structural, magnetic, and dielectric properties of Ni–Zn ferrite and Bi2O3 nanocomposites prepared by the sol-gel method, Chin. Phys. B, 2021, 30, 096102 CrossRef CAS.
- S. Tajik, Y. Orooji, Z. Ghazanfari, F. Karimi, H. Beitollahi, R. S. Varma, H. W. Jang and M. Shokouhimehr, Nanomaterials modified electrodes for electrochemical detection of Sudan I in food, J. Food Meas. Charact., 2021, 15(4), 3837–3852 CrossRef.
- M. H. Omar, K. A. Razak, M. N. Ab Wahab and H. H. Hamzah, Recent progress of conductive 3D-printed electrodes based upon polymers/carbon nanomaterials using a fused deposition modelling (FDM) method as emerging electrochemical sensing devices, RSC Adv., 2021, 11(27), 16557–16571 RSC.
- J. Li, S. Zhang, L. Zhang, Y. Zhang, H. Zhang, C. Zhang, X. Xuan, M. Wang, J. Zhang and Y. Yuan, A novel graphene-based nanomaterial modified electrochemical sensor for the detection of cardiac troponin I, Front. Chem., 2021, 9, 1–8 Search PubMed.
- S. Fu, Y. Zhu, Y. Zhang, M. Zhang, Y. Zhang, L. Qiao, N. Yin, K. Song, M. Liu and D. Wang, Recent advances in carbon nanomaterials-based electrochemical sensors for phenolic compounds detection, Microchem. J., 2021, 171, 106776 CrossRef CAS.
- M. Khan, M. N. Tahir, S. F. Adil, H. U. Khan, M. R. H. Siddiqui, A. A. Al-Warthan and W. Tremel, Graphene based metal and metal oxide nanocomposites: synthesis, properties and
their applications, J. Mater. Chem. A, 2015, 3, 18753–18808 RSC.
- S. K. Kandasamy, Graphene oxide, in Graphene, Nanotubes and Quantum Dots-Based Nanotechnology, Woodhead Publishing, 2022, pp. 155–172 Search PubMed.
- H. Beitollahi, M. Safaei, M. R. Shishehbore and S. Tajik, Application of Fe3O4@SiO2/GO nanocomposite for sensitive and selective electrochemical sensing of tryptophan, J. Electrochem. Sci. Eng., 2019, 9(1), 45–53 CrossRef CAS.
- S. Amani, N. Sohrabi, R. Mohammadi and I. Ahadzadeh, Synthesis and investigation of CoMnFeO4/reduced graphene oxide as ecofriendly electrode material for supercapacitor and its electrochemical performances, J. Alloys Compd., 2023, 937, 168020 CrossRef CAS.
- B. C. Behera, S. N. Sarangi, N. K. Sahoo, S. P. Dash and S. K. Tripathy, Magnetic Nanoparticles-Based Novel Sensors for Select Biomedical/Biological Science Applications, Biomaterials-Based Sensors, 2023, Vol. 1, pp. 325–348 Search PubMed.
- Y. Poo-arporn, S. Pakapongpan, N. Chanlek and R. P. Poo-arporn, The development of disposable electrochemical sensor based on Fe3O4-doped reduced graphene oxide modified magnetic screen-printed electrode for ractopamine determination in pork sample, Sens. Actuators, B, 2019, 284, 164–171 CrossRef CAS.
- C. McKeever, S. Callan, S. Warren and E. Dempsey, Magnetic nanoparticle modified electrodes for voltammetric determination of propellant stabiliser diphenylamine, Talanta, 2022, 238, 123039 CrossRef CAS PubMed.
- M. Vahidifar and Z. Es’haghi, Magnetic Nanoparticle-Reinforced Dual-Template Molecularly Imprinted Polymer for the Simultaneous Determination of Oxazepam and Diazepam Using an Electrochemical Approach, J. Anal. Chem., 2022, 77(5), 625–639 CrossRef.
- M. Valian, A. Khoobi and M. Salavati-Niasari, Synthesis, characterization and electrochemical sensors application of Tb2Ti2O7 nanoparticle modified carbon paste electrode for the sensing of mefenamic acid drug in biological samples and pharmaceutical industry wastewater, Talanta, 2022, 123593 CrossRef CAS PubMed.
- Y. Wang, H. Zhu, Y. Chen, X. Wu, W. Zhang, C. Luo and J. Li, Design of hollow ZnFe2O4 microspheres@graphene decorated with TiO2 nanosheets as a high-performance low frequency absorber, Mater. Chem. Phys., 2017, 202, 184–189 CrossRef CAS.
- S. Kumar, R. Walia, A. Kumar and V. Verma, Hybrid structure of MWCNT/ferrite and GO incorporated composites for microwave shielding properties and their practical applications, RSC Adv., 2021, 11(17), 9775–9787 RSC.
- Y. Li, D. Li, J. Yang, H. Luo, F. Chen, X. Wang and R. Gong, Enhanced microwave absorption and surface wave attenuation properties of Co0.5Ni0.5Fe2O4 fibers/reduced graphene oxide composites, Materials, 2018, 11(4), 508–518 CrossRef PubMed.
- Y. O. Al-Ghamdi, M. Jabli, M. H. Alhalafi, A. Khan and K. A. Alamry, Alamry Hybridized sulfated-carboxymethyl cellulose/MWNT nanocomposite as highly selective electrochemical probe for trace detection of arsenic in real environmental samples, RSC Adv., 2023, 13, 18382–18395 RSC.
- S. Javanbakht, M. Pooresmaeil and H. Namazi, Green one-pot synthesis of carboxymethylcellulose/Zn-based metal-organic framework/graphene oxide bio-nanocomposite as a nanocarrier for drug delivery system, Carbohydr. Polym., 2019, 208, 294–301 CrossRef CAS PubMed.
- M. Pooresmaeil, H. Namazi and R. Salehi, Simple method for fabrication of metal-organic framework within a carboxymethylcellulose/graphene quantum dots matrix as a carrier for anticancer drug, Int. J. Biol. Macromol., 2020, 164, 2301–2311 CrossRef CAS PubMed.
- B. Borisova, A. Sánchez, S. Jiménez-Falcao, M. Martín, P. Salazar, C. Parrado, J. M. Pingarrón and R. Villalonga, Reduced graphene oxide-carboxymethylcellulose layered with platinum nanoparticles/PAMAM dendrimer/magnetic nanoparticles hybrids. Application to the preparation of enzyme electrochemical biosensors, Sens. Actuators, B, 2016, 232, 84–90 CrossRef CAS.
- K. Juengchareonpoon, P. Wanichpongpan and V. Boonamnuayvitaya, Trimethoprim adsorption using graphene oxide-carboxymethylcellulose film coated on polyethylene terephthalate as a supporter, Chem. Eng. Process., 2021, 169, 108641 CrossRef CAS.
- C. Arenas, E. Sánchez-Tirado, I. Ojeda, C. Gómez-Suárez, A. González-Cortés, R. Villalonga, P. Yáñez-Sedeño and J. Pingarrón, An electrochemical immunosensor for adiponectin using reduced graphene oxide–carboxymethylcellulose hybrid as electrode scaffold, Sens. Actuators, B, 2016, 223, 89–94 CrossRef CAS.
- A. Pendashteh, M. F. Mousavi and M. S. Rahmanifar, Fabrication of anchored copper oxide nanoparticles on graphene oxide nanosheets via an electrostatic coprecipitation and its application as supercapacitor, Electrochim. Acta, 2013, 88, 347–357 CrossRef CAS.
- E. Sohouli, E. M. Khosrowshahi, P. Radi, E. Naghian, M. Rahimi-Nasrabadi and F. Ahmadi, Electrochemical sensor based on modified methylcellulose by graphene oxide and Fe3O4 nanoparticles: Application in the analysis of uric acid content in urine, J. Electroanal. Chem., 2020, 877, 114503–114513 CrossRef CAS.
- M. Yadav, K. Rhee, I. Jung and S. Park, Eco-friendly synthesis, characterization and properties of a sodium carboxymethyl cellulose/graphene oxide nanocomposite film, Cellulose, 2013, 20(2), 687–698 CrossRef CAS.
- Y. Peng, C. Xia, M. Cui, Z. Yao and X. Yi, Effect of reaction condition on microstructure and properties of (NiCuZn)Fe2O4 nanoparticles synthesized via co-precipitation with ultrasonic irradiation, Ultrason. Sonochem., 2021, 71, 105369–105379 CrossRef CAS PubMed.
- H. Harzali, F. Saida, A. Marzouki, A. Megriche, F. Baillon, F. Espitalier and A. Mgaidi, Structural and magnetic properties of nano-sized NiCuZn ferrites synthesized by co-precipitation method with ultrasound irradiation, J. Magn. Magn. Mater., 2016, 419, 50–56 CrossRef CAS.
- F. Saida, H. Harzali, A. Marzouki, A. Mgaidi and A. Megriche, Effect of cobalt substitution on the structural and magnetic properties of nanopowders Ni0.4Cu0.2Zn0.4Fe2O4 by hydrothermal method, Chem. Afr., 2017, 19, 26–33 CAS.
- Y. He, C. Lei, Q. Lin, J. Dong, Y. Yu and L. Wang, Mössbauer and Structural Properties of La-Substituted Ni0.4Cu0.2Zn0.4Fe2O4 Nanocrystalline Ferrite, Sci. Adv. Mater., 2015, 7, 1809–1815 CrossRef CAS.
- B. Liu, W. Wang, J. Wang, Y. Zhang, K. Xu and F. Zhao, Preparation and catalytic activities of CuFe2O4 nanoparticles assembled with graphene oxide for RDX thermal decomposition, J. Nanopart. Res., 2019, 21, 48 CrossRef.
- A. Afkhami, H. Khoshsafar, H. Bagheri and T. Madrakian, Preparation of NiFe2O4/graphene nanocomposite and its application as a modifier for the fabrication of an electrochemical sensor for the simultaneous determination of tramadol and acetaminophen, Anal. Chim. Acta, 2014, 831, 50–59 CrossRef CAS PubMed.
- B. Habibi, S. Pashazadeh, L. A. Saghatforoush and A. Pashazadeh, Direct electrochemical synthesis of the copper based metal-organic framework on/in the heteroatoms doped graphene/pencil graphite electrode: Highly sensitive and selective electrochemical sensor for sertraline hydrochloride, J. Electroanal. Chem., 2021, 888, 115210–115222 CrossRef CAS.
- A. J. Bard and L. R. Faulkner, Fundamentals and applications: electrochemical methods, Electrochem. Methods, 2001, 2(482), 580–632 Search PubMed.
- M. K. Bojdi, M. Behbahani, M. H. Mashhadizadeh, A. Bagheri, S. S. H. Davarani and A. Farahani, Mercapto-ordered carbohydrate-derived porous carbon electrode as a novel electrochemical sensor for simple and sensitive ultra-trace detection of omeprazole in biological samples, Mater. Sci. Eng. Carbon, 2015, 48, 213–219 CrossRef PubMed.
- C. Ferrag, M. Noroozifar and K. Kerman, Thiol functionalized carbon ceramic electrode modified with multi-walled carbon nanotubes and gold nanoparticles for simultaneous determination of purine derivatives, Mater. Sci. Eng. Carbon, 2020, 110, 110568 CrossRef CAS PubMed.
- G. G. Mohamed, F. A. N. El-Dieny, S. M. Khalilz and A. S. El-Mohammad, Metal complexes of omeprazole. Preparation, spectroscopic and thermal characterization and biological activity, J. Coord. Chem., 2009, 62, 645–654 CrossRef CAS.
- C. Ferrag, M. Noroozifar and K. Kerman, Ultralight 3D Graphene Oxide Aerogel Decorated with Pd–Fe Nanoparticles for the Simultaneous Detection of Eight Biomolecules, ACS Appl. Mater. Interfaces, 2023, 15, 27502–27514 CrossRef CAS PubMed.
- M. Nemakal, S. Aralekallu, I. Mohammed, M. Pari, K. V. Reddy and L. K. Sannegowda, Nanomolar detection of 4-aminophenol using amperometric sensor based on a novel phthalocyanine, Electrochim. Acta, 2019, 318, 342–353 CrossRef CAS.
- S. Aralekallu, M. Palanna, S. Hadimani, K. P. CP, V. A. Sajjan, M. O. Thotiyl and L. K. Sannegowda, Biologically inspired catalyst for electrochemical reduction of hazardous hexavalent chromium, Dalton Trans., 2020, 49(42), 15061–15071 RSC.
- H. M. Abumelha, A. Q. Alorabi, H. Alessa, N. A. Alamrani, A. Alharbi, A. A. Keshk and N. M. El-Metwaly, Novel Iron Oxide Nanoparticle-Fortified Carbon Paste Electrode for the Sensitive Voltammetric Determination of Atomoxetine, ACS Omega, 2023, 8, 19006–19015 CrossRef CAS PubMed.
- S. Shahrokhian, M. Ghalkhani, M. Bayat and F. Ghorbani-Bidkorbeh, Voltammetric behavior and determination of trace amounts of omeprazole using an edge-plane pyrolytic graphite electrode, Iran. J. Pharm. Res., 2015, 14(2), 465–471 CAS.
- V. Gallardo, M. López-Viota, J. Sierra and M. Ruiz, Spectrophotometric and chromatographic determination of omeprazole in pharmaceutical formulations, Pharm. Dev. Technol., 2009, 14(5), 516–523 CrossRef CAS PubMed.
- I. Al-Salloum and B. Al-Sabti, Stability study of esomeprazole in different packaging material using stability indicating validating HPLC method, World J. Pharm. Pharm. Sci., 2019, 8, 20–25 CAS.
- P. Gupta and R. N. Goyal, A sensitive pyrolytic graphite sensor for determination of omeprazole in human blood plasma and pharmaceuticals, J. Electrochem. Soc., 2014, 161(4), H255–H259 CrossRef CAS.
- A. M. Qaisi, M. F. Tutunji and L. F. Tutunji, Acid decomposition of omeprazole in the absence of thiol: a differential pulse polarographic study at the static mercury drop electrode (SMDE), J. Pharm. Sci., 2006, 95(2), 384–391 CrossRef CAS PubMed.
- A. Afkhami, S. Sayari, F. Soltani-Felehgari and T. Madrakian, Ni0.5Zn0. 5Fe2O4 nanocomposite modified carbon paste electrode for highly sensitive and selective simultaneous electrochemical determination of trace amounts of mercury (II) and cadmium (II), J. Iran. Chem. Soc., 2015, 12(2), 257–265 CrossRef CAS.
- B. Habibi, S. Pashazadeh, L. A. Saghatforoush and A. Pashazadeh, A thioridazine hydrochloride electrochemical sensor based on zeolitic imidazolate framework-67-functionalized bio-mobile crystalline material-41 carbon quantum dots, New J. Chem., 2021, 45(32), 14739–14750 RSC.
- M. Altuntaş, D. B. Altuntaş, S. Aslan, E. Yılmaz and E. Nalbant, Determination of Exogenous Adrenaline Levels in Patients Undergoing Cardiopulmonary Resuscitation, ACS Omega, 2023, 8, 19425–19432 CrossRef PubMed.
- H. F. Assaf, H. Salah, N. Hashem, M. Khodari and A. Toghan, Fabrication of an electrochemical sensor based on copper waste wire recycling and its application, Sens. Actuators, A, 2021, 331, 112962 CrossRef CAS.
- N. El-Enany, F. Belal and M. Rizk, The alternating current polarographic behavior and determination of lansoprazole and omeprazole in dosage forms and biological fluids, J. Biochem. Biophys. Methods, 2008, 70(6), 889–896 CrossRef CAS PubMed.
- A. Radi, Anodic voltammetric assay of lansoprazole and omeprazole on a carbon paste electrode, J. Pharm. Biomed. Anal., 2003, 31(5), 1007–1012 CrossRef CAS PubMed.
- J.-L. Yan, Electrochemical behavior and the determination of omeprazole using glassy carbon electrode, J. Appl. Sci., 2006, 6(7), 1625–1627 CrossRef CAS.
- K. Deng, X. Liu, C. Li, Z. Hou and H. Huang, An electrochemical omeprazole sensor based on shortened multi-walled carbon nanotubes-Fe3O4 nanoparticles and poly (2, 6-pyridinedicarboxylic acid), Sens. Actuators, B, 2017, 253, 1–9 CrossRef CAS.
- A. Salimi, Z. Enferadi, A. Noorbakhash and K. Rashidi, Sensitive amperometric detection of omeprazole and pantoperazole at electrodeposited nickel oxide nanoparticles modified glassy carbon electrode, J. Solid State Electrochem., 2012, 16(4), 1369–1375 CrossRef CAS.
- K. R. Mahanthesha, B. E. K. Swamy and K. V. Pai, Poly (alizarin) Modified glassy carbon electrode for the electrochemical investigation of omeprazole: A voltammetric study, Anal. Bioanal. Electrochem., 2014, 6(2), 234–244 Search PubMed.
- S. McClean, E. O'kane, V. Ramachandran and W. Smyth, Differential pulse polarographic study of the degradation of H+/K+ ATPase inhibitors SK&F 95601 and omeprazole in acidic media and the subsequent reactions with thiols, Anal. Chim. Acta, 1994, 292(1–2), 81–89 CrossRef CAS.
- K. Pandian, J. Kalayarasi and S. C. Gopinath, Metal-free Sulfur-doped graphitic carbon nitride-modified GCE-based electrocatalyst for the enhanced electrochemical determination of Omeprazole in Drug formulations and Biological Samples, Biotechnol. Appl. Biochem., 2022, 69(6), 2766–2779 CrossRef CAS PubMed.
|
This journal is © The Royal Society of Chemistry 2023 |
Click here to see how this site uses Cookies. View our privacy policy here.