DOI:
10.1039/D3RA04750D
(Paper)
RSC Adv., 2023,
13, 25660-25672
Ruthenium complexes of 1,4-diazabutadiene ligands with a cis-RuCl2 moiety for catalytic acceptorless dehydrogenation of alcohols: DFT evidence of chemically non-innocent ligand participation†
Received
15th July 2023
, Accepted 7th August 2023
First published on 29th August 2023
Abstract
The acceptorless dehydrogenative coupling (ADC) of primary alcohols to esters by diazabutadiene-coordinated ruthenium compounds is reported. Treatment of cis-Ru(dmso)4Cl2 in acetone at 56 °C with different 1,4-diazabutadienes [p-XC6H4N
C(H)(H)C
NC6H4X-p; X = H, CH3, OCH3, and Cl; abbreviated as DAB-X], gives trans-Ru[κ2-N,N-DAB-X]2Cl2 as the kinetic product of substitution. Heating these products in o-xylene at 144 °C gives the thermodynamically favored cis-Ru[κ2-N,N-DAB-X]2Cl2 isomers. Electronic structure calculations confirm the greater stability of the cis diastereomer. The molecular structures for each pair of geometric isomers have been determined by X-ray diffraction analyses. Cyclic voltammetry experiments on the complexes show an oxidative response and a reductive response within 0.50 to 0.93 V and −0.76 to −1.24 V vs. SCE respectively. The cis-Ru[κ2-N,N-DAB-X]2Cl2 complexes function as catalyst precursors for the acceptorless dehydrogenative coupling of primary alcohols to H2 and homo- and cross-coupled esters. When 1,4-butanediol and 1,5-pentanediol are employed as substrates, lactones and hydroxyaldehydes are produced as the major dehydrogenation products, while secondary alcohols afforded ketones in excellent yields. The mechanism for the dehydrogenation of benzyl alcohol to benzyl benzoate and H2 using cis-Ru[κ2-N,N-DAB-H]2Cl2 (cis-1) as a catalyst precursor was investigated by DFT calculations. The data support a catalytic cycle that involves the four-coordinate species Ru[κ2-N,N-DAB-H][κ1-N-DAB-H](κ1-OCH2Ph) whose protonated κ1-diazabutadiene moiety functions as a chemically non-innocent ligand that facilitates a β-hydrogen elimination from the κ1-O-benzoxide ligand to give the corresponding hydride HRu[κ2-N,N-DAB-H][κ1-N-DAB-H](κ2-O,C-benzaldehyde). H2 production follows a Noyori-type elimination to give (H2)Ru[κ2-N,N-DAB-H][κ1-N-DAB-H](κ1-O-benzaldehyde) as an intermediate in the catalytic cycle.
Introduction
Ruthenium-promoted chemistry continues to attract worldwide attention from both the academic and industrial sectors. From the catalysis of key commodity chemicals to their use as chemotherapeutic agents,1–4 ruthenium compounds play a pivotal role in helping to address some of the critical challenges faced by the consumer products industry. Of the many different types of ruthenium compounds known, those having a cis-RuCl2 moiety are of particular interest in the catalysis and biomedical fields. Interest in ruthenium(II) complexes with a cis-RuCl2 moiety remains high, with attention directed toward their use in the preparation of new complexes,2 as efficient DNA binding and anti-tumor agents,3 and as catalyst precursors for a variety of organic transformations.4 The vast majority of these applications rely on the enhanced lability of the Ru–Cl bond, which, in turn, is influenced by the nature of the other ligands in the primary coordination sphere of the ruthenium.5 The coordination of ruthenium by π-acid ligands is fundamental for the stabilization of the ruthenium(II) oxidation state, and such ligands also augment the lability of the Ru–Cl bonds in cis-Ru(α-diimine)2Cl2 and related complexes to furnish syn coordination sites in a well-defined molecular environment that synergistically act on organic substrates to effect site-selective activation and value-added functionalization.6,7 We maintain an interest in this genre of compounds and have published several papers on the synthesis and the catalytic activity of new nitrogen- and phosphine-coordinated ruthenium complexes possessing a reactive cis-RuCl2 moiety in transfer hydrogenation and acceptorless dehydrogenative coupling reactions.8
Wishing to branch out and to explore different ruthenium precursors as catalysts for the directed synthesis of complex organic molecules, we have initiated studies on a series of α-diimine-coordinated ruthenium compounds with the common formula cis-Ru[κ2-N,N-p-XC6H4N
C(H)(H)C
NC6H4X-p]2Cl2, where the p-XC6H4N
C(H)(H)C
NC6H4X-p ligand is based on the 1,4-diazabutadiene (DAB) platform (Scheme 1). These nitrogen donors are easily prepared from glyoxal and para-substituted anilines and they typically function as κ2-N,N-donors to give five-membered chelate rings.9 Numerous electrochemical and photophysical studies have demonstrated notable π-acidity of the κ2-bound p-XC6H4N
C(H)(H)C
NC6H4X-p ligands,10 a property that also promotes the stabilization of metals in low oxidation states; this latter feature is catalytically desirable and remains an important consideration in the design of late-metal catalysts for hydrogenation and C–C bond coupling reactions.11
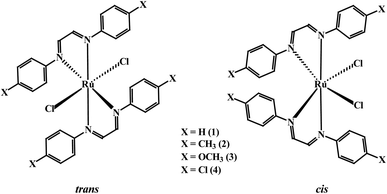 |
| Scheme 1 1,4-Diazabutadiene-coordinated ruthenium(II) compounds 1–4 investigated. | |
Interest in the catalytic conversion of primary alcohols to esters by acceptorless dehydrogenative coupling (ADC) remains high compared to traditional synthetic methods for ester formation from alcohols (eqn (1)).12 ADC catalysis is environmentally friendly, and it
|
 | (1) |
aligns itself nicely with our current emphasis on green catalysis, not to mention ADC chemistry is atom-economical in nature.
13,14 The coupling of two alcohols to furnish an ester in the absence of stoichiometric oxidizing reagents remains a priority in the field of catalysis. Herein we present our results on the synthesis and catalytic properties in the acceptorless dehydrogenative coupling (ADC) of alcohols to esters by new 1,4-diazabutadiene-coordinated compounds
cis-Ru[κ
2-
N,
N-p-XC
6H
4N
![[double bond, length as m-dash]](https://www.rsc.org/images/entities/char_e001.gif)
C(H)(H)C
![[double bond, length as m-dash]](https://www.rsc.org/images/entities/char_e001.gif)
NC
6H
4X-
p]
2Cl
2. The catalytic mechanism for the observed ADC reaction of benzyl alcohol to benzyl benzoate and H
2 was investigated by electronic structure calculations, and a mechanism involving Ru(0) intermediate and a chemically non-innocent κ
1-
N-p-XC
6H
4N
![[double bond, length as m-dash]](https://www.rsc.org/images/entities/char_e001.gif)
C(H)(H)C
![[double bond, length as m-dash]](https://www.rsc.org/images/entities/char_e001.gif)
N(H)C
6H
4X-
p ligand, which is formed by hydrogen transfer from the hydrido-alkoxide intermediate
cis-HRu[κ
2-
N,
N-p-XC
6H
4N
![[double bond, length as m-dash]](https://www.rsc.org/images/entities/char_e001.gif)
C(H)(H)C
![[double bond, length as m-dash]](https://www.rsc.org/images/entities/char_e001.gif)
N(H)C
6H
4X-
p]
2(κ
1-OCH
2Ph), is discussed.
Results and discussion
Syntheses and characterization
As delineated in the introduction, in order to explore the catalytic efficiency of cis-Ru(DAB-X)2Cl2 in acceptorless dehydrogenation of alcohols, we prepared a series of Ru(DAB-X)2Cl2 compounds 1–4 (Scheme 1). Treatment of the cis-Ru(dmso)4Cl2 with a slight excess of two equivalents of DAB-X in refluxing acetone afforded a green complex having general formula Ru(DAB-X)2Cl2 in good yield. Use of the relatively low boiling solvent (acetone) for the synthetic reaction was intended for retaining the cis-RuCl2 fragment of the cis-Ru(dmso)4Cl2 starting compound in the final bis-chelated product. The crystal structures of the green products authenticated the trans-geometry of the Ru(DAB-X)2Cl2 complexes. Structure of trans-Ru(DAB-OCH3)2Cl2 (trans-3) is displayed in Fig. 1. The structure clearly shows that the two DAB-OCH3 ligands are coordinated to ruthenium, and have constituted an equatorial plane with ruthenium at the center with two trans coordinated chlorides (Scheme 1). The molecular structures of the other three green products, viz. trans-1, trans-2 and trans-4, are similar to that of trans-3, and these are deposited as Fig. S1 (ESI†). 1H NMR data of the green Ru(DAB-X)2Cl2 complexes are also consistent with the trans-geometry.
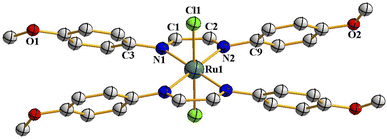 |
| Fig. 1 Molecular structures of trans-3. The thermal ellipsoids are drawn at the 50% probability level. Hydrogen atoms have been omitted for clarity. | |
As DAB-X ligands are symmetric in nature, the Ru(DAB-X)2Cl2 complexes can, in principle, exist in two geometrical isomeric forms, viz. cis and trans, in view of relative disposition of the two coordinated chlorides (Scheme 1). Formation of the trans-isomer from cis-Ru(dmso)4Cl2 in boiling acetone was quite intriguing, particularly as Ru(diimine)2Cl2 type complexes are well known to prefer the cis-geometry for better π-interaction.7,15 The difference in thermodynamic stability of the cis and trans isomers of Ru(DAB-X)2Cl2, complexes was investigated by DFT calculations. For each complex, the cis-isomer was found to be more stable than the trans-isomer (Fig. S2, (ESI†)). The fact, that in spite of the greater thermodynamic stability of the cis-isomer, the formation of trans-isomer in the refluxing acetone indicates that the trans-isomer is probably the kinetically controlled product. Conversion of trans-isomer to the more stable cis-isomer could not take place in refluxing acetone, presumably due to unavailability of adequate energy required for the isomerization.
Following the hint from the energy calculations, reaction of DAB-X with cis-[Ru(dmso)4Cl2] was next carried out in a relatively high-boiling solvent, viz. ortho-xylene, under refluxing condition, which afforded blue (X = H, Me, Cl) or bluish-green (X = OCH3) product having the same general formula Ru(DAB-X)2Cl2. Crystal structures of these complexes, as well as their 1H NMR spectra, confirmed the cis-geometry of them. Molecular structure of cis-3 is shown in Fig. 2 as a representative, and structures of the remaining three complexes (cis-1, cis-2 and cis-4) are shown in Fig. S3 (ESI†). In summary, heating the DAB-X and cis-[Ru(dmso)4Cl2] in acetone gives the trans isomer as the kinetically controlled product, while refluxing the starting reagents in o-xylene affords the thermodynamically favored cis isomer. The isolated trans-isomers are also found to be quantitatively convertible into the corresponding cis-isomers by simply heating the former in refluxing ortho-xylene.16 The solvent dependent formation of trans- and cis-isomers and conversion of trans → cis are summarized in Scheme 2. The observed trans → cis isomerization in each group of isomers proceeds by a unimolecular reaction that is believed to involve a Bailar-twist process through a trigonal prismatic transition state.17,18
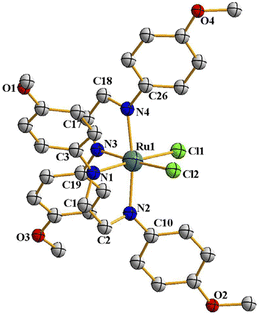 |
| Fig. 2 Molecular structures of cis-3. The thermal ellipsoids are drawn at the 50% probability level. Hydrogen atoms have been omitted for clarity. | |
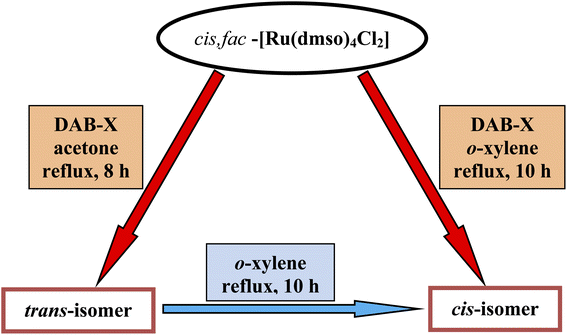 |
| Scheme 2 Formation of trans- and cis-isomers, and conversion of trans → cis. | |
The isomers of complexes 1–4 were characterized by elemental analysis and traditional spectroscopic methods, and these data are summarized in the Experimental section. Examination of the known structures on deposit with the Cambridge Crystallographic Data Centre (CSD version 5.40) reveals only three entries with the general formula Ru(DAB-X)2Cl2 of which two structures are polymorphs. The deposited structures are consistent with the formulated composition for each isomer and do not exhibit any significant differences in their structural parameters. Reports on the synthesis of isomers of compounds 2 and 3 by methods significantly different from ours exist in the literature.17,19
The structures depicted in Fig. 1, 2, S1 and S3 (ESI†) confirm that the two 1,4-diazabutadiene ligands chelate the ruthenium in the expected fashion (Scheme 1). The observed Ru–Cl and Ru–N distances in each isomer compare well with those found earlier.19–21 The ruthenium center is six-coordinate in each structure, and the subtended angles at the metal are consistent with the arrangement of the ligands in each isomer. The C–C and C–N bond lengths within the chelating DAB-X ligands in trans- and cis-complexes deviate significantly from localized C–C single bond and C
N double bond distances in keeping with an enhanced delocalization of the π-cloud and back-donation from the electron-rich, low-spin d6 ruthenium center.
Spectral and electrochemical properties
The 1H NMR spectral data of the complexes are presented in the Experimental section. Number of signals observed in each of the trans isomers is consistent with the presence of two C2 axes. For example, only one signal near 8.6 ppm is observed for the four azomethine protons. In each of the cis isomers, two azomethine proton signals were found within 8.1–8.6 ppm, indicating existence of only one C2 axis.
Electronic spectra of the complexes were recorded in dichloromethane solutions, and the spectral data are given in the Experimental section. Each complex shows two intense absorptions in the visible region, which are tentatively attributed to metal-to-ligand (DAB-X) charge-transfer transitions. The intense absorptions in the ultraviolet region are assignable to transitions within the DAB-X ligand orbitals.
Redox activity of the trans- and cis-Ru(DAB-X)2Cl2 complexes was examined in acetonitrile solution (0.1 M TBHP) by cyclic voltammetry. Each complex shows a reversible oxidation within 0.5 to 1.0 V and an irreversible reduction within −0.7 to −1.3 V (all potentials are referenced to SCE). Voltammetric data are presented in Table S9 (ESI†), and a representative CV is shown in Fig. S4 (ESI†). The oxidative response is assigned to Ru(II)–Ru(III) oxidation. In the trans-isomers the Ru(II)–Ru(III) oxidation occurs at a much lower (ca. 300 mV) potential compared to that in the corresponding cis isomers, which is attributed to difference in stereochemical preference of Ru(II) and Ru(III).7,15,22 In cis-Ru(bpy)2Cl2, an exhaustively studied complex, the Ru(II)–Ru(III) oxidation takes place at 0.29 V,23 while in the cis-Ru(DAB-X)2Cl2 complexes the same oxidation occurs at ca. 0.8 V. The observed positive shift of about 500 mV indicates that these 1,4-diazabutadiene ligands are better π-acids than bpy.
The Ru(II)–Ru(III) oxidation potential in the trans- and cis-Ru(DAB-X)2Cl2 complexes is found to be sensitive to the nature of substituent X in the DAB-X ligand. The potential increases with increasing electron-withdrawing character of X. The plot of oxidation potentials vs. Hammett constant of the substituents (4σ; the σ values being:24 OCH3 = −0.27, CH3 = −0.17, H = 0.00 and Cl = 0.23) is linear for both trans-[Ru(DAB-X)2Cl2] and cis-[Ru(DAB-X)2Cl2] complexes (Fig. S5 (ESI†)). The observed slope (slope = reaction constant (ρ) of this redox couple25) is 0.09 V for the trans-complexes and 0.12 V for the cis-complexes. From these linear correlations with reasonable slopes it is clear that the substituents on the 1,4-diazabutadiene ligands, which are six bonds away from the electroactive metal center, can influence the redox potential in a predictable manner. The irreversible reductive response is attributed to reduction of the α-diimine fragment in the 1,4-diazabutadiene ligands. Potential of this reduction does not show any systematic variation with the nature of substituent X.
Catalytic acceptorless dehydrogenation of alcohols
As outlined in the introduction, the other major objective of this study was to explore the catalytic activity of a series of cis-Ru(DAB-X)2Cl2 complexes in acceptorless dehydrogenation of alcohols. The high efficiency of catalyst precursors with a cis-RuCl2 moiety [or cis-Ru(H)Cl or cis-Ru(H)2 moieties derived from a cis-RuCl2 unit] in fundamental bond-activation reactions is well-documented in the literature.12,13 We began our study by examining the dehydrogenation of benzyl alcohol using 0.1 mol% cis-Ru(DAB-X)2Cl2 as the catalyst precursor in toluene as a solvent. These data are summarized in Table S10 (ESI†), and surprisingly, instead of observing benzaldehyde as the dominant product of dehydrogenation, benzyl benzoate was obtained in relatively good yield [entry 1, Table S10 (ESI†)].26 The presence of H2 was verified by 1H NMR spectroscopy [Fig. S6 (ESI†)].27 The coupling of benzyl alcohol to furnish benzyl benzoate in the absence of an added oxidant or sacrificial hydrogen acceptor is, though precedent,14g,i quite interesting. The formation of benzyl benzoate and H2 as reaction products proceeds by an initial dehydrogenation of benzyl alcohol, followed by a dehydrogenative C–O bond coupling involving the parent alcohol and benzaldehyde (eqn (1)). Having established the ADC reaction with benzyl alcohol using cis-1, we next investigated the catalytic activity of the other cis isomers based on compounds 2–4; these data are found in entries 13–15 in Table S10 (ESI†). The three other catalyst precursors also give benzyl benzoate as the major product in yields that range from 66% (cis-4) to 43% (cis-3). The presence of small amounts of benzaldehyde (<15%) was confirmed in each of these reactions. Qualitatively, the para substituent in the four catalysts investigated mildly influences the overall yield of product ester, with the electron-donating Me and MeO groups in cis-2 and cis-3 affording lower yields of benzyl benzoate relative to the phenyl and para-chloro derivatives. Of the four different catalyst precursors examined, cis-1 was the most active, and the optimal reaction conditions using cis-1 were ultimately finalized as listed in entry 2 of Table S10 (ESI†). Accordingly, we selected cis-1 for more detailed screening studies with other alcohol substrates, the results of which are presented below.
The scope of the dehydrogenation using different primary alcohols and cis-1 as a catalyst was next investigated using the optimized conditions from the benzyl alcohol reaction [entry 2; Table S10 (ESI†)]. Five additional primary alcohols were examined as substrates, and the catalysis results are reported in Table 1. All of the primary alcohols examined furnished the corresponding ester as the major dehydrogenated product. The yield of ester yield ranged from good (51% for 1-butanol) to excellent (90% for isobutyl alcohol), with the corresponding aldehyde present in yields that ranged from a high of 18% (1-butanol) to a low of 1% (isobutyl alcohol). Though relatively less common, several reports concerning the ruthenium-catalyzed formation of esters via ADC of alcohols exist.14a,e,g–i
Table 1 Dehydrogenation of primary alcoholsa
We next explored the efficacy of cis-1 in the cross-coupling of two different alcohols to furnish mixed-ester products (eqn (2)) since transition-metal catalyzed dehydrogenative cross-coupling reactions are few in number. A recent report by Milstein and coworkers confirms the successful cross-coupling of primary alcohols to form cross-esters using a manganese pincer complex.28 Table 2 summarizes our ester cross-coupling results. We employed the standard reaction conditions adopted for the benzyl alcohol cross-coupling [entry 2, Table S10 (ESI†)] and here we used a 1
:
1 mixture of primary alcohols. In principle, four different esters may be
|
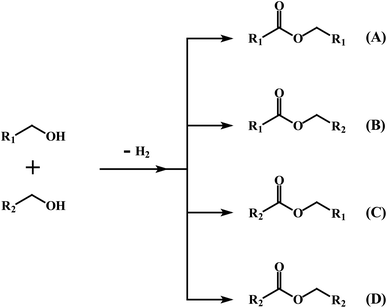 | (2) |
produced, as illustrated by the reaction depicted in the header of
Table 2. However, only the reaction using ethanol and benzyl alcohol afforded all four possible ester products (entry 5). The remaining reactions gave their respective homo-esters and only one of the two cross-coupled esters as observed products (entries 1–4). The observed variation in product distribution is, presumably, due to difference in bulk of the alkyl/aryl moieties (R and R′) in the alcohols, which, in turn, influences approach of these substrates to the metal center in the catalyst, and hence the reaction kinetics. We also examined the ADC reaction using the terminal diols 1,4-butanediol and 1,5-pentanediol and found the cyclized products γ-butyrolactone, and δ-valerolactone, respectively, as the major product. Accompanying each lactone was the corresponding hydroxyaldehyde. The data from the diol reactions support a scheme that involves initial oxidation to give a hydroxyaldehyde whose intramolecular cyclization is slightly faster (∼2×) compared to the dissociation of the initial oxidation product from the κ
1-aldehyde intermediate. The observed lactone products are in keeping with the Ru-catalyzed lactone formation from terminal diols reported earlier by the groups of Szymczak
14g and Hartwig.
14j
Table 2 Dehydrogenative cross-coupling of two primary alcoholsa
Dehydrogenation of terminal diols |
|
Diol |
Product-Ib |
Product-IIb |
6 |
 |
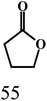 |
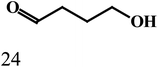 |
7 |
 |
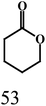 |
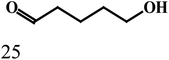 |
Finally, the dehydrogenation of selected secondary alcohols was investigated using cis-1 (eqn (3)). Similar experimental conditions, as in the case of primary alcohols, were used,
|
 | (3) |
except that the catalyst loading had to be increased to 0.2 mol% for a better yield. The yield of ketone product was lower by a factor of two with a 0.1 mol% catalyst loading.
Table 3 shows the results of our dehydrogenation of secondary alcohols. The expected ketone was obtained in excellent yield in each case, and the evolution of H
2 was again verified by
1H NMR studies (Fig. S7 (ESI
†)). Control experiments, carried out under an argon atmosphere, afforded the same ketone in comparable yield (
cf.: entries 1 and 6), serving to eliminate the participation molecular oxygen in the alcohol oxidation. As expected, no ester product was found in any of these reactions that employed a secondary alcohol as the substrate. The observed catalytic efficiency of
cis-
1 towards acceptorless dehydrogenation of alcohols is better than that of many other Ru-catalysts,
14b–d,f,g,i,j and comparable to one reported Ru-complex.
14a
Table 3 Dehydrogenation of secondary alcoholsa
Mechanism for the acceptorless dehydrogenative coupling reaction
The ruthenium-mediated formation of benzyl benzoate requires, at a minimum, two successive catalytic steps involving the dehydrogenation of benzyl alcohol to benzaldehyde, followed by C–O coupling with additional alcohol. A likely contributor to the catalytic cycle is the unsaturated ruthenium(0) intermediate Ru(DAB-H)2, whose formation may be traced to an initial reduction process with the alcohol substrate serving as the reducing agent in the Ru(II) → Ru(0) process. Generation of ruthenium(0) species bearing soft ligands is precedent in literature.29 Scheme 3 shows a sequence of events that serves to convert cis-1 to the κ1-O-benzaldehyde complex E. The activation of cis-1 by the alcohol substrate was experimentally examined using ethanol at room temperature. Treatment of cis-1 with ethanol led to a slow increase in the solution conductivity (Λ = 12.0 S cm2 mol−1) over the course of several hours in support of the ion pair [Ru(DAB-H)2(EtOH)Cl][Cl].30 While not examined, deprotonation of the ionic intermediate produced from benzyl alcohol would give the corresponding chloro-alkoxide species C, which upon loss of additional HCl would furnish the κ1-O-benzaldehyde complex E. Dissociation of the aldehyde from the latter species or its reaction with additional alcohol to give the homocoupled-ester product would ultimately furnish the key catalytic intermediate Ru(DAB-H)2. The proposed reduction pathway depicted in Scheme 3 is strengthened by reports on glycol reduction of related ruthenium(II) precursors to active ruthenium(0) species used in C–H and O–H bond activation reactions.31
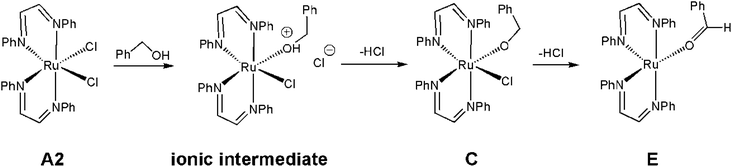 |
| Scheme 3 Proposed path for the alcohol-promoted Ru(II) → Ru(0) process starting from cis-1. | |
Before addressing the mechanism of the acceptorless dehydrogenative C–O coupling reaction involving cis-1 and benzyl alcohol, we wished to establish the structure of the unsaturated intermediate Ru(DAB-H)2 by DFT calculations. As a formal d8-ML4 species, Ru(DAB-H)2 can exist as either a singlet or triplet ground state with a structure that can range from a square planar to a distorted tetrahedral geometry.32,33 Unrestricted optimization of Ru(DAB-H)2 confirmed the triplet species 3G to be 11.0 kcal mol−1 more stable (ΔH) than the corresponding singlet species 1G. The optimized structure of 3G (Fig. 3) exhibits a deformed square-planar structure that possesses idealized D2 symmetry due to a slight twist in the DAB rings. The origin of the observed twist is attributed to unfavorable van der Waals interactions between the phenyl groups on the adjacent chelate rings that effectively prohibit the core RuN4 atoms from adopting a planar arrangement. The N–Ru–N bite angle in each chelate ring is 77.8° while the mean intermolecular angle for the cis and trans N–Ru–N linkages between the rings is 103.9° and 166.0°, respectively. The related 16e complex Ru(dmpe)2 [where dmpe = 1,2-bis(dimethylphosphino)ethane] has been reported to be a singlet based on UV-vis data with a structure based on a square-planar geometry. The singlet state proposed for this d8-ML4 species was reproduced computationally using the model compound Ru(PH3)4.33c That Ru(dmpe)2 is a singlet is reinforced by the near diffusion limit for the capture of CO and H2.34 The optimization of 3G as a triplet as opposed to a singlet in the case of Ru(dmpe)2 likely reflects subtle differences in the ordering of the frontier orbitals as influenced by the nature of the donor ligands (N vs. P) and the backbone of the chelating ligands.
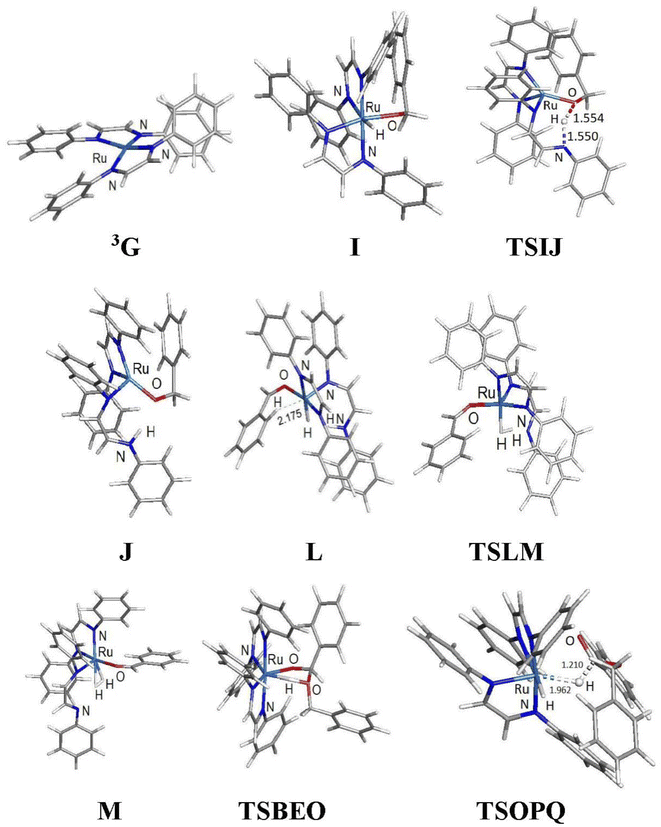 |
| Fig. 3 DFT-optimized structures for selected participants in the ADC reaction mechanism depicted in Fig. 4. Displayed bond distances for species TSIJ, L, and TSOPQ are in Å. | |
Fig. 4 shows the computed catalytic cycle starting from species 3G that is produced from species E on completion of the dehydrogenative C–O coupling with additional benzyl alcohol (B) or through dissociation of benzaldehyde (F) from species E. The unsaturated intermediate 3G is integral as an entry point into the catalytic cycle. The potential energy profile for the reaction under consideration is depicted in Fig. 5. Benzyl alcohol (B) addition to 3G affords the five-coordinate compound H which serves as the precursor to the octahedral hydridoalkoxide species I. Transfer of the hydride (as a proton) to an adjacent nitrogen atom of a DAB ligand proceeds via TSIJ and yields a protonated DAB ligand that undergoes a κ2-N,N → κ1-N transformation in the process to give species J that contains a κ1-N-C6H5N
C(H)(H)C
N(H)C6H5 ligand. The latter species, which lies 6.8 kcal mol−1 above I, is of interest because its formation underscores the chemically non-innocent role played by the ancillary DAB ligand in the catalytic cycle. Related to our results are the computational data reported by Li and Hall on the conversion of methanol to H2 and CO2 by the ruthenium complex [HRu(trop2dad)][K(dme)2] whose ancillary 1,4-bis(5H-dibenzo[a,d]cycloheptenyl-5-yl)-1,4-diazabuta-1,3-diene ligand (trop2dad) also functions as a chemically non-innocent ligand that exclusively controls the formation of the reported products.35 Here the ruthenium metal functions as a template for ligand binding and remains as a spectator throughout the catalytic cycle.
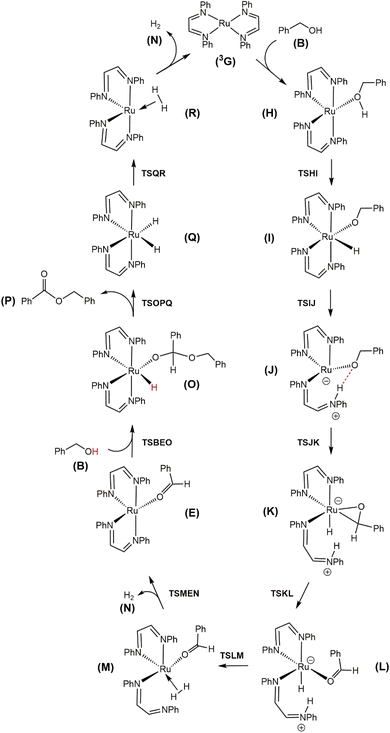 |
| Fig. 4 DFT-computed acceptorless dehydrogenative coupling mechanism from Ru(DAB-H)2 (3G) and benzyl alcohol (B) to give H2 (N) and benzyl benzoate (P). | |
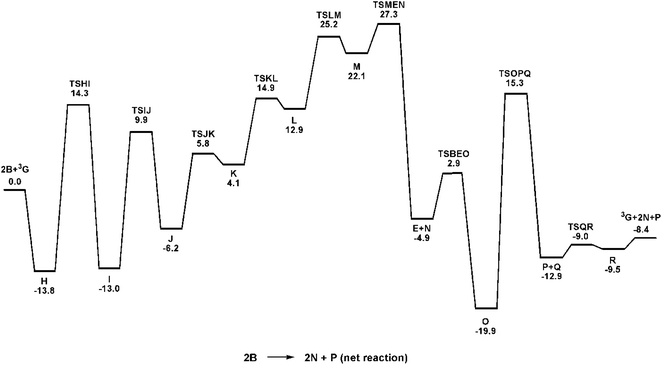 |
| Fig. 5 Potential energy profile for the reaction of Ru(DAB-H)2 (3G) and benzyl alcohol (B) to give H2 (N) and benzyl benzoate (P). Relative energies (ΔH) in kcal mol−1 with respect to 2B + 3G. | |
β-Hydrogen elimination from a benzylic site in J gives the hydrido species K that contains a κ2-O,C-benzaldehyde moiety and an intact and protonated κ1-N-DAB ligand. Transformation of the coordinated benzaldehyde moiety in K to the oxygen-bound κ1 species in L facilitates the formation of the molecular H2 complex M. The H2 ligand in M derives from a Noyori-type elimination involving the protonated “imine-like” N–H group on the κ1-N-DAB moiety and the terminal hydride ligand. The elimination process that gives M proceeds via TSLM with a barrier of 12.3 kcal mol−1. Dissociative loss of H2 from M is coupled with concomitant chelation of the pendant DAB ligand, leading to the aldehyde complex E which completes the first phase of the dehydrogenation reaction.
The C–O bond coupling portion of the ADC reaction is initiated by TSBEO. The second equivalent of benzyl alcohol (B) adds to the vacant site in E that is syn to the coordinated benzaldehyde to furnish the hydridoalkoxide O. The H–O moiety of the incoming alcohol adds synchronously to the metal center and aldehyde carbon in E, respectively, to form a five-membered ring not unlike that reported for other substrate activation processes that display bifunctional behavior.14g,36 This addition step exhibits a barrier of 7.8 kcal mol−1 and proceeds through TSBEO. Transfer of the hydride (as a proton) in O to an adjacent nitrogen atom was modeled as was done for I → J, but all attempts to model this process were unfavorable, giving a κ2-O,O-coordinated alkoxyether species and other unreasonable structures that proved resistant to β-hydrogen elimination and release of the product ester. We were able to successfully model the release of the benzyl benzoate product (P) through a benzylic β-hydrogen elimination through TSOPQ that is overall rate-limiting and proceeds with a barrier of 35.2 kcal mol−1. Activation of the C–H bond in this step is accompanied by the release of the ester P and formation of the dihydride Q. The penultimate step involves the reductive elimination of the hydrides to give the H2 complex R, which on the release of H2 (N) furnishes 3G to complete the ADC reaction. Finally, the overall reaction (eqn (1)) is computed to be exothermic by 8.4 kcal mol−1. It is relevant to mention here that utilization of imine-coordinated ruthenium(0) species in catalysis is precedent in the literature.29a,37
Conclusion
We have prepared a series of ruthenium compounds with the general formula cis-Ru(DAB-X)2Cl2 [where DAB = p-XC6H4N
C(H)(H)C
NC6H4X-p; X= H, CH3, OCH3, and Cl] and have investigated their reactivity in the dehydrogenation of primary and secondary alcohols. We confirm that the cis-Ru(DAB-X)2Cl2 complexes are efficient catalyst precursors for acceptorless dehydrogenation of primary alcohols, which is followed by dehydrogenative C–O coupling of the resulting aldehyde and the additional alcohol to yield esters and H2. The dehydrogenation reaction with secondary alcohols proceeds similarly and stops at the ketone product. DFT calculations support the coordination of the alcohol substrate by the unsaturated species Ru(κ2-N,N-DAB)2 to give the κ1-O alcohol compound H. Transfer of the hydroxy hydrogen in H to the metal affords the octahedral intermediate cis-HRu(κ2-N,N-DAB)2(κ1-OCH2Ph) (I), whose hydride is transferred to an adjacent DAB ligand to give the protonated ligand complex Ru[κ2-N,N-C6H5N
C(H)(H)C
NC6H5][κ1-N-C6H5N
C(H)(H)C
N(H)C6H5](κ1-OCH2Ph) (J). The ability of the chemically non-innocent DAB ligand to function as a proton acceptor, coupled with its participation in the bifunctional release of H2 from the hydride species HRu[κ2-N,N-C6H5N
C(H)(H)C
NC6H5][κ1-N-C6H5N
C(H)(H)C
N(H)C6H5](κ1-O-benzaldehyde) (L) through an outer-sphere elimination is computationally supported. Our current efforts center on the study of other nitrogen-based donors that can function in a capacity similar to that exhibited by the DAB ligands here. The catalytic results from these studies will be disseminated in due course.
Experimental section
Materials
Ruthenium trichloride was purchased from Arora Matthey, Kolkata, India. cis,fac-Ru(dmso)4Cl2 was prepared by following a reported procedure.38 Glyoxal was obtained from SD Fine Chem, Mumbai, India, and the 4-X-anilines (X = OCH3, CH3, H, and Cl) were procured from Merck, India. The 1,4-diazabutadiene ligands were prepared by condensation of glyoxal with the 4-X-anilines in hot methanol. All other chemicals and solvents were reagent grade commercial materials and were used as received.
Physical measurements
Microanalyses (C, H, and N) were performed using a Heraeus Carlo Erba 1108 elemental analyzer, and magnetic susceptibilities were measured using a Sherwood MK-1 balance. 1H NMR spectra were recorded in CDCl3 solution at 300 MHz on a Bruker Avance DPX 300 NMR spectrometer. IR spectra were recorded on a PerkinElmer Spectrum Two IR spectrometer, with samples prepared as KBr pellets. Electronic spectra were recorded on a JASCO V-630 spectrophotometer. Solution electrical conductivities were measured using an Elico CM 180 conductivity meter with a solute concentration of ca. 10−3 M. GC-MS analyses were performed using a PerkinElmer CLARUS 680 instrument.
Compound preparation
The synthetic details associated with the preparation of the isomeric compounds trans-Ru[κ2-N,N-p-XC6H4N
C(H)(H)C
NC6H4X-p]2Cl2 (trans-1) and cis-Ru[κ2-N,N-p-XC6H4N
C(H)(H)C
NC6H4X-p]2Cl2 (cis-1) are described below. The remaining six compounds were synthesized similarly and we report only the yield and physical data for each derivative of 2–4.
trans-1. To a solution of DAB-H (0.11 g, 0.51 mmol) in warm acetone (40 mL) was added cis-Ru(dmso)4Cl2 (0.10 g, 0.21 mmol), and the mixture was heated at reflux for 8 h. The resulting dark solution was allowed to stand overnight, whereby trans-1 separated as a dark crystalline precipitate, which was collected by filtration, washed with cold acetone, and dried in air. Additional trans-1 was obtained from the filtrate following evaporation and chromatographic purification of the residue on a silica plate using 1
:
1 dichloromethane–chloroform as the eluent. Yield: 0.10 g (80%). Anal. calc. for C28H24N4Cl2Ru: C, 57.13; H, 4.08; N, 9.52. Found: C, 57.18; H, 4.11; N, 9.49. 1H NMR (300 MHz, CDCl3): δ 6.90 (t, 8H, J = 7.5); 7.08 (t, 4H, J = 7.5); 7.22 (d, 8H, J = 7.5); 8.68 (s, 4H). IR (KBr pellet, cm−1): 521, 585, 604, 623, 696, 759, 836, 852, 881, 916, 1002, 1028, 1071, 1175, 1208, 1300, 1312, 1342, 1447, 1483, 1584, 1628. UV-vis in CH2Cl2 [λmax/nm (ε/M−1 cm−1)]: 706 (8700), 411 (7900), 307 (8100), 269 (11
800).
cis-1. To a solution of DAB-H (0.11 g, 0.51 mmol) in o-xylene (40 mL) was added cis-Ru(dmso)4Cl2 (0.10 g, 0.21 mmol), after which the mixture was heated at reflux for 10 h. The resulting bluish-green solution was allowed to stand overnight, whereby cis-1 separated as a dark crystalline solid. The desired product was collected by filtration, washed with ether, and dried in air. Additional cis-1 was obtained from the filtrate following evaporation and chromatographic purification of the residue on a silica plate using 1
:
1 dichloromethane–chloroform as the eluent. Yield: 0.09 g (77%). Anal. calc. for C28H24N4Cl2Ru: C, 57.13; H, 4.08; N, 9.52. Found: C, 57.18; H, 4.11; N, 9.49. 1H NMR (300 MHz, CDCl3): δ 6.78 (d, 4H, J = 7.5); 7.01–7.32 (m, 12H)*; 7.37 (d, 4H, J = 7.2); 8.32 (s, 2H); 8.50 (s, 2H). IR (KBr pellet, cm−1): 524, 613, 694, 759, 846, 954, 1024, 1075, 1208, 1275, 1345, 1447, 1485, 1536, 1591, 1625. UV-vis in CH2Cl2 [λmax/nm (ε/M−1 cm−1)]: 572 (7500), 473 (2300), 350 (10
000).
trans-2. Yield: 0.11 g (81%). Anal. calc. for C32H32N4Cl2Ru: C, 59.63; H, 4.97; N, 8.70. Found: C, 59.91; H, 4.95; N, 8.73. 1H NMR (300 MHz, CDCl3): δ 2.27 (s, 12H, CH3); 6.69 (d, 8H, J = 8.1); 7.09 (d, 8H, J = 8.1); 8.64 (s, 4H). IR (KBr pellet, cm−1): 502, 559, 645, 763, 807, 847, 867, 1020, 1039, 1062, 1103, 1170, 1210, 1278, 1347, 1380, 1466, 1499, 1634. UV-vis in CH2Cl2 [λmax/nm (ε/M−1 cm−1)]: 707 (8000), 434 (9400), 313 (7800), 267 (12
000).
cis-2. Yield: 0.10 g (78%). Anal. calc. for C32H32N4Cl2Ru: C, 59.63; H, 4.97; N, 8.70. Found: C, 60.28; H, 4.98; N, 8.79. 1H NMR (300 MHz, CDCl3): δ 2.33 (s, 6H, CH3); 2.40 (s, 6H, CH3); 6.68 (d, 4H, J = 8.5); 6.79 (d, 4H, J = 8.5); 7.04 (d, 4H, J = 8.5); 7.13 (d, 4H, J = 8.5); 8.25 (s, 2H); 8.41 (s, 2H). IR (KBr pellet, cm−1): 569, 770, 815, 956, 1021, 1069, 1108, 1215, 1345, 1361, 1454, 1501, 1623. UV-vis in CH2Cl2 [λmax/nm (ε/M−1 cm−1)]: 576 (8300), 479 (2900), 372 (14
000).
trans-3. Yield: 0.13 g (91%). Anal. calc. for C32H32N4O4Cl2Ru: C, 54.24; H, 4.52; N, 7.91. Found: C, 54.33; H, 4.53; N, 7.93. 1H NMR (300 MHz, CDCl3): δ 3.78 (s, 12H, OCH3), 6.43 (d, 8H, J = 8.9), 7.18 (d, 8H, J = 8.9), 8.67 (s, 4H). IR (KBr pellet, cm−1): 461, 505, 556, 573, 642, 645, 661, 760, 798, 828, 846, 867, 925, 938, 1034, 1069, 1113, 1169, 1212, 1254, 1298, 1349, 1456, 1502, 1550, 1601. UV-vis in CH2Cl2 [λmax/nm (ε/M−1 cm−1)]: 708 (10
600), 472 (15
500), 343 (10
000), 238 (35
900).
cis-3. Yield: 0.12 g (82%). Anal. calc. for C32H32N4O4Cl2Ru: C, 54.24; H, 4.52; N, 7.91. Found: C, 54.72; H, 4.49; N, 7.96. 1H NMR (300 MHz, CDCl3): δ 3.83 (s, 6H, OCH3); 3.84 (s, 6H, OCH3); 6.76 (d, 4H, J = 8.7); 6.80 (d, 4H, J = 8.7); 7.11 (d, 4H, J = 8.8); 7.36 (d, 4H, J = 8.8); 8.19 (s, 2H); 8.34 (s, 2H). IR (KBr pellet, cm−1): 569, 658, 756, 798, 824, 1031, 1107, 1168, 1212, 1254, 1301, 1342, 1502, 1580, 1602. UV-vis in CH2Cl2 [λmax/nm (ε/M−1 cm−1)]: 586 (7800), 407 (17
400), 241 (33
200).
trans-4. Yield: 0.11 g (75%). Anal. calc. for C28H20N4Cl6Ru: C, 46.27; H, 2.75; N, 7.71. Found: C, 46.44; H, 2.73; N, 7.71. 1H NMR (300 MHz, CDCl3): δ 6.98 (d, 8H, J = 8.6); 7.21 (d, 8H, J = 8.6); 8.65 (s, 4H). IR (KBr pellet, cm−1): 535, 677, 715, 752, 819, 833, 874, 1014, 1091, 1167, 1208, 1345, 1412, 1456, 1482, 1590, 1635. UV-vis in CH2Cl2 [λmax/nm (ε/M−1 cm−1)]: 714 (6300), 421 (7300), 309 (7600), 281 (10
000).
cis-4. Yield: 0.11 g (75%). Anal. calc. for C28H20N4Cl6Ru: C, 46.27; H, 2.75; N, 7.71. Found: C, 46.52; H, 2.79; N, 7.68. 1H NMR (300 MHz, CDCl3): δ 6.43 (d, 4H, J = 8.6); 6.72 (d, 4H, J = 8.6); 7.10 (d, 4H, J = 8.5); 7.18 (d, 4H, J = 8.5); 8.33 (s, 2H); 8.50 (s, 2H). IR (KBr pellet, cm−1): 540, 597, 683, 715, 750, 823, 1013, 1091, 1170, 1211, 1285, 1345, 1405, 1450, 1480, 1539, 1588, 1635. UV-vis in CH2Cl2 [λmax/nm (ε/M−1 cm−1)]: 578 (10
400), 479 (3100), 364 (17
100).
X-ray crystallography
Methods for growing single crystals of the complexes: trans-3 and trans-1: slow evaporation of solvents from solutions of the complexes in 1
:
1 acetonitrile–dichloromethane; trans-2: slow diffusion of toluene into a solution of the complex in dichloromethane; trans-4: slow evaporation of solvents from a solution of the complex in 1
:
1 acetonitrile–chloroform; cis-3, cis-2 and cis-1: diffusion of toluene (for cis-3 and cis-1) or benzene (for cis-2) into a solution of the complex in dichloromethane (for cis-3 and cis-2), or chloroform (for cis-1); cis-4: slow evaporation of solvent from a solution of the complex in toluene.
The molecular structure of each compound was established by X-ray crystallography. Tables S1–S4 (ESI†) contain the pertinent crystallographic data collection and processing parameters for the four pairs of isomers. The X-ray data for trans-3 were collected on a CrysAlis Pro, Super Nova (Agilent Technologies) diffractometer using Cu Kα radiation (λ = 1.54184 Å), while the data on the remaining compounds were collected on a Bruker SMART CCD diffractometer using Mo Kα radiation (λ = 0.71073 Å). X-ray data reduction, structure solution, and refinement were done using the SHELXS-97 and SHELXL-97 packages, and Olex2.39 The structures were solved by direct methods. Tables S5–S8 (ESI†) report selected bond distances and angles for the isomeric pairs of compounds.
General procedure for acceptorless dehydrogenation of alcohols
In a typical run, an oven-dried 10 mL round-bottomed flask was charged with the alcohol substrate (1.0 mmol), KOtBu (1.0 mol%), and the desired catalyst precursor (0.1–0.2 mol%), followed the addition of 5 mL of a binary solvent mixture composed of 1
:
4 dichloromethane–toluene. The flask was attached to a condenser fitted with a rubber septum at the end. A needle was pierced through the septum to allow release of uncondensed volatiles. The whole setup was placed in a preheated oil bath for refluxing. After the specified time, the flask was removed from the oil bath and water (20 mL) added, followed by extraction with diethyl ether (4 × 10 mL). The combined organic layers were washed with water (3 × 10 mL), dried over anhydrous Na2SO4, and filtered. The solvent was removed under vacuum, after which the residue was dissolved in hexane and analyzed by GCMS.
For verification of H2 evolution, the open end of the needle was sealed with a rubber block. After about 30 min, the rubber block was removed and the tip of the needle was inserted into ice-cold CDCl3, kept in a NMR tube, for 1 min. The resulting solution was examined for presence of H2 by 1H NMR spectroscopy.
Computational modeling details
The reported DFT calculations were performed with the hybrid meta exchange–correlation functional M06,40 as implemented by the Gaussian 09 program package.41 The Ru atoms were described by Stuttgart–Dresden effective core potentials (ECP28MWB) and an SDD basis set,42 while a 6-31G(d′) basis set was employed for the remaining atoms.43
The reported geometries (gas-phase at 298.15 K) represent fully optimized ground states (positive eigenvalues) and transition states (one imaginary eigenvalue) as verified from the analytical Hessian. The computed frequencies were used to make zero-point and thermal corrections to the electronic energies; the reported enthalpies (ΔH) are quoted in kcal mol−1 relative to the specified standard. The geometry-optimized structures have been drawn with the JIMP2 molecular visualization and manipulation program.44
Energy differences between cis and trans geometries of complexes 1–4 were done using density functional theory (DFT) with the B3LYP exchange–correlation functional,45 as implemented in Gaussian 09 program.41 The lanl2dz basis set was used for Ru,46 and 6-31G(d) was employed for the other elements.47
Conflicts of interest
The authors declare no conflict of interest.
Acknowledgements
SB acknowledges financial assistance received from the Department of Science and Technology (DST-SERB), New Delhi [Reference No. CRG/2022/003382], while MGR thanks the Robert A. Welch Foundation (grant B-1093) for continued financial support. UNT computational resources provided through CASCaM, which is an NSF-supported facility (CHE-1531468) is gratefully acknowledged. AM thanks for her fellowship [grant number 09/096(0962)/2019-EMR-I]. The authors thank Prof. Saurabh Das of JU for his help in recording the UV-vis spectra of the new complexes.
References
-
(a) O. M. Ogba, N. C. Warner, D. J. O'Leary and R. H. Grubbs, Chem. Soc. Rev., 2018, 47, 4510–4544 RSC;
(b) D. A. Hey, R. M. Reich, W. Baratta and F. E. Kühn, Coord. Chem. Rev., 2018, 374, 114–132 CrossRef CAS;
(c) J. A. Leitch and C. G. Frost, Chem. Soc. Rev., 2017, 46, 7145–7153 RSC;
(d) L. Zeng, P. Gupta, Y. Chen, E. Wang, L. Ji, H. Chao and Z.-S. Chen, Chem. Soc. Rev., 2017, 46, 5771–5804 RSC;
(e) G. Chelucci, Coord. Chem. Rev., 2017, 331, 1–36 CrossRef CAS;
(f) S. Chanthamath and S. Iwasa, Acc. Chem. Res., 2016, 49, 2080–2090 CrossRef CAS PubMed;
(g) M. Hirano and S. Komiya, Coord. Chem. Rev., 2016, 314, 182–200 CrossRef CAS;
(h) L. Duan, L. Wang, F. Li, F. Li and L. Sun, Acc. Chem. Res., 2015, 48, 2084–2096 CrossRef CAS PubMed;
(i) F. Li, J. G. Collins and F. R. Keene, Chem. Soc. Rev., 2015, 44, 2529–2542 RSC;
(j) A. K. Singh, D. S. Pandey, Q. Xu and P. Braunstein, Coord. Chem. Rev., 2014, 270–271, 31–56 CrossRef CAS;
(k) C. Gunanathan and D. Milstein, Chem. Rev., 2014, 114, 12024–12087 CrossRef CAS PubMed;
(l) S. Manzini, J. A. Fernández-Salas and S. P. Nolan, Acc. Chem. Res., 2014, 47, 3089–3101 CrossRef CAS PubMed;
(m) P. B. Arockiam, C. Bruneau and P. H. Dixneuf, Chem. Rev., 2012, 112, 5879–5918 CrossRef CAS PubMed.
-
(a) S. G. Eaves, D. S. Yufit, B. W. Skelton, J. M. Lynam and P. J. Low, Dalton Trans., 2015, 44, 21016–22102 RSC;
(b) K. B. Ghiassi, D. T. Walters, M. M. Aristov, N. D. Loewen, L. A. Berben, M. Rivera, M. M. Olmstead and A. L. Balch, Inorg. Chem., 2015, 54, 4565–4573 CrossRef CAS PubMed;
(c) H. Zhao, T. Zhang, T. Yan and M. Cai, J. Org. Chem., 2015, 80, 8849–8855 CrossRef CAS PubMed;
(d) E. S. F. Ma, D. C. Mudalige, B. O. Patrick and B. R. James, Dalton Trans., 2013, 42, 7614–7621 RSC;
(e) D. H. Nguyen, J.-C. Daran, S. Mallet-Ladeira, T. Davin, L. Maron, M. Urrutigoïty, P. Kalck and M. Gouygou, Dalton Trans., 2013, 42, 75–81 RSC;
(f) R. Gilbert-Wilson, L. D. Field and M. M. Bhadbhade, Inorg. Chem., 2012, 51, 3239–3246 CrossRef CAS PubMed.
-
(a) Y. Kong, F. Chen, Z. Su, Y. Qian, F. Wang, X. Wang, J. Zhao, Z.-W. Mao and H.-K. Liu, J. Inorg. Biochem., 2018, 182, 194–199 CrossRef CAS PubMed;
(b) L. Zeng, P. Gupta, Y. Chen, E. Wang, L. Ji, H. Chao and Z.-S. Chen, Chem. Soc. Rev., 2017, 46, 5771–5804 RSC;
(c) K. Jeyalakshmi, J. Haribabu, N. S. P. Bhuvanesh and R. Karvembu, Dalton Trans., 2016, 45, 12518–12531 RSC;
(d) R. Pettinari, F. Marchetti, F. Condello, C. Pettinari, G. Lupidi, R. Scopelliti, S. Mukhopadhyay, T. Riedel and P. J. Dyson, Organometallics, 2014, 33, 3709–3715 CrossRef CAS;
(e) R. Aznar, A. Grabulosa, A. Mannu, G. Muller, D. Sainz, V. Moreno, M. Font-Bardia, T. Calvet and J. Lorenzo, Organometallics, 2013, 32, 2344–2362 CrossRef CAS;
(f) N. Busto, J. Valladolid, M. Martínez-Alonso, H. J. Lozano, F. A. Jalón, B. R. Manzano, A. M. Rodríguez, M. C. Carrión, T. Biver, J. M. Leal, G. Espino and B. García, Inorg. Chem., 2013, 52, 9962–9974 CrossRef CAS PubMed;
(g) G. Gasser and N. Metzler-Nolte, Curr. Opin. Chem. Biol., 2012, 16, 84–91 CrossRef CAS PubMed.
-
(a) X.-N. Cao, X.-M. Wan, F.-L. Yang, K. Li, X.-Q. Hao, T. Shao, X. Zhu and M.-P. Song, J. Org. Chem., 2018, 83, 3657–3668 CrossRef CAS PubMed;
(b) F. Chotard, R. Malacea-Kabbara, C. Balan, E. Bodio, M. Picquet, P. Richard, M. Ponce-Vargas, P. Fleurat-Lessard and P. L. Gendre, Organometallics, 2018, 37, 812–820 CrossRef CAS;
(c) J. Francos, L. Menéndez-Rodríguez, E. Tomás-Mendivil, P. Crochet and V. Cadierno, RSC Adv., 2016, 6, 39044–39052 RSC;
(d) J. Tauchman, B. Therrien, G. Süss-Fink and P. Štěpnička, Organometallics, 2012, 31, 3985–3994 CrossRef CAS;
(e) M. Hans, Q. Willem, J. Wouters, A. Demonceau and L. Delaude, Organometallics, 2011, 30, 6133–6142 CrossRef CAS;
(f) R. García-Álvarez, J. Díez, P. Crochet and V. Cadierno, Organometallics, 2011, 30, 5442–5451 CrossRef.
- A. Mukherjee and S. Bhattacharya, RSC Adv., 2021, 11, 15617–15631 RSC.
-
(a) N. A. F. Al-Rawashdeh, S. Chatterjee, J. A. Krause and W. B. Connick, Inorg. Chem., 2014, 53, 294–307 CrossRef CAS PubMed;
(b) Y. Sun, M. E. Ojaimi, R. Hammitt, R. P. Thummel and C. Turro, J. Phys. Chem. B, 2010, 114, 14664–14670 CrossRef CAS PubMed;
(c) M. Al-Noaimi, M. I. El-Barghouthi, M. El-khateeb, O. S. Abdel-Rahman, H. Görls and R. J. Crutchley, Polyhedron, 2008, 27, 2698–2704 CrossRef CAS;
(d) M. Z. Al-Noaimi, R. J. Abdel-Jalil, S. F. Haddad, R. H. Al-Far and R. J. Crutchley, Inorg. Chim. Acta, 2006, 359, 2395–2399 CrossRef CAS.
-
(a) R. A. Krause and K. Krause, Inorg. Chem., 1984, 23, 2195–2198 CrossRef CAS;
(b) S. Goswami, A. R. Chakravarty and A. Chakravorty, Inorg. Chem., 1983, 22, 602–609 CrossRef CAS;
(c) S. Goswami, A. R. Chakravarty and A. Chakravorty, Inorg. Chem., 1982, 21, 2737–2742 CrossRef CAS;
(d) R. A. Krause and K. Krause, Inorg. Chem., 1982, 21, 1714–1720 CrossRef CAS;
(e) R. A. Krause and K. Krause, Inorg. Chem., 1980, 19, 2600–2603 CrossRef CAS.
-
(a) A. Mukherjee, D. A. Hrovat, M. G. Richmond and S. Bhattacharya, Dalton Trans., 2018, 47, 10264–10272 RSC;
(b) A. Mukherjee, P. Paul and S. Bhattacharya, J. Organomet. Chem., 2017, 834, 47–57 CrossRef CAS;
(c) J. Dutta, M. G. Richmond and S. Bhattacharya, Eur. J. Inorg. Chem., 2014, 4600–4610 CrossRef CAS;
(d) B. K. Dey, J. Dutta, M. G. B. Drew and S. Bhattacharya, J. Organomet. Chem., 2014, 750, 176–184 CrossRef CAS.
-
(a) J. R. Lawson, L. C. Wilkins, M. André, E. C. Richards, M. N. Ali, J. A. Platts and R. L. Melen, Dalton Trans., 2016, 45, 16177–16181 RSC;
(b) B. G. Shestakov, T. V. Mahrova, J. Larionova, J. Long, A. V. Cherkasov, G. K. Fukin, K. A. Lyssenko, W. Scherer, C. Hauf, T. V. Magdesieva, O. A. Levitskiy and A. A. Trifonov, Organometallics, 2015, 34, 1177–1185 CrossRef CAS;
(c) S. C. Patra, A. Saha Roy, V. Manivannan, T. Weyhermüller and P. Ghosh, Dalton Trans., 2014, 43, 13731–13741 RSC;
(d) D. A. J. Harding, E. G. Hope, K. Singh and G. A. Solan, Polyhedron, 2012, 33, 360–366 CrossRef CAS;
(e) J. Langer and H. Görls, Inorg. Chem. Commun., 2011, 14, 1612–1615 CrossRef CAS.
-
(a) F. Hartl, P. Rosa, L. Ricard, P. Le Floch and S. Záliš, Coord. Chem. Rev., 2007, 251, 557–576 CrossRef CAS;
(b) M. D. Walter, D. J. Berg and R. A. Andersen, Organometallics, 2007, 26, 2296–2307 CrossRef CAS;
(c) M. M. Khusniyarov, T. Weyhermüller, E. Bill and K. Wieghardt, J. Am. Chem. Soc., 2009, 131, 1208–1221 CrossRef CAS PubMed;
(d) W. Kaim, M. Sieger, S. Greulich, B. Sarkar, J. Fiedler and S. Záliš, J. Organomet. Chem., 2010, 695, 1052–1058 CrossRef CAS.
-
(a) J. R. Fulton, A. W. Holland, D. J. Fox and R. G. Bergman, Acc. Chem. Res., 2002, 35, 44–56 CrossRef CAS PubMed;
(b) S. D. Ittel, L. K. Johnson and M. Brookhart, Chem. Rev., 2000, 100, 1169–1204 CrossRef CAS PubMed.
-
(a) M. Trincado, J. Bösken and H. Grützmacher, Coord. Chem. Rev., 2021, 443, 213967 CrossRef CAS;
(b) F. R. Keene, Coord. Chem. Rev., 1999, 187, 121–149 CrossRef CAS;
(c) M. Trincado, D. Banerjee and H. Grützmacher, Energy Environ. Sci., 2014, 7, 2464–2503 RSC;
(d) N. Nicolas Sieffert and M. Bühl, J. Am. Chem. Soc., 2010, 132, 8056–8070 CrossRef PubMed.
-
(a) R. G. Alabau, M. A. Esteruelas, A. Martínez, M. Oliván and E. Oñate, Organometallics, 2018, 37, 2732–2740 CrossRef CAS;
(b) K. Paudel, B. Pandey, S. Xu, D. K. Taylor, D. L. Tyer and C. L. Torres, Org. Lett., 2018, 20, 4478–4481 CrossRef CAS PubMed;
(c) V. Cherepakhin and T. J. Williams, ACS Catal., 2018, 8, 3754–3763 CrossRef CAS PubMed;
(d) D.-W. Tan, H.-X. Li, M.-J. Zhang, J.-L. Yao and J.-P. Lang, ChemCatChem, 2017, 9, 1113–1118 CrossRef CAS;
(e) S. Chakraborty, P. E. Piszel, W. W. Brennessel and W. D. A Jones, Organometallics, 2015, 34, 5203–5206 CrossRef CAS;
(f) S. Chakraborty, P. O. Lagaditis, M. Förster, E. A. Bielinski, N. Hazari, M. C. Holthausen, W. D. Jones and S. Schneider, ACS Catal., 2014, 4, 3994–4003 CrossRef CAS;
(g) A. V. Polukeev, P. V. Petrovskii, A. S. Peregudov, M. G. Ezernitskaya and A. A. Koridze, Organometallics, 2013, 32, 1000–1015 CrossRef CAS;
(h) G. Zhang, K. V. Vasudevan, B. L. Scott and S. K. Hanson, J. Am. Chem. Soc., 2013, 135, 8668–8681 CrossRef CAS PubMed;
(i) C. Gunanathan and D. Milstein, Science, 2013, 341, 1229712 CrossRef PubMed.
-
(a) D. H. Nguyen, X. Trivelli, F. Capet, Y. Swesi, A. Favre-Réguillon, L. Vanoye, F. Dumeignil and R. M. Gauvin, ACS Catal., 2018, 8, 4719–4734 CrossRef CAS;
(b) Z. Wang, B. Pan, Q. Liu, E. Yue, G. A. Solan, Y. Maa and W.-H. Sun, Catal. Sci. Technol., 2017, 7, 1654–1661 RSC;
(c) Q. Wang, H. Chai and Z. Yu, Organometallics, 2017, 36, 3638–3644 CrossRef CAS;
(d) I. Dutta, A. Sarbajna, P. Pandey, S. M. W. Rahaman, K. Singh and J. K. Bera, Organometallics, 2016, 35, 1505–1513 CrossRef CAS;
(e) C. Vicent and D. G. Gusev, ACS Catal., 2016, 6, 3301–3309 CrossRef CAS;
(f) K.-N. T. Tseng, J. W. Kampf and N. K. Szymczak, ACS Catal., 2015, 5, 5468–5485 CrossRef CAS;
(g) K.-N. T. Tseng, J. W. Kampf and N. K. Szymczak, Organometallics, 2013, 32, 2046–2049 CrossRef CAS;
(h) M. Nielsen, H. Junge, A. Kammer and M. Beller, Angew. Chem., Int. Ed., 2012, 51, 5711–5713 CrossRef CAS PubMed;
(i) S. Shahane, C. Fischmeister and C. Bruneau, Catal. Sci. Technol., 2012, 2, 1425–1428 RSC;
(j) J. Zhao and J. F. Hartwig, Organometallics, 2005, 24, 2441–2446 CrossRef CAS;
(k) J. Zhang, M. Gandelman, L. J. W. Shimon, H. Rozenberg and D. Milstein, Organometallics, 2004, 23, 4026–4033 CrossRef CAS.
-
(a) A. H. Velders, K. van der Schilden, A. C. G. Hotze, J. Reedijk, H. Kooijman and A. L. Spek, Dalton Trans., 2004, 448–455 RSC;
(b) D. Hesek, Y. Inoue, S. R. L. Everitt, H. Ishida, M. Kunieda and M. G. B. Drew, J. Chem. Soc., Dalton Trans., 1999, 3701–3709 RSC.
- For conversion of 50 mg trans-isomer into the corresponding cis-isomer, refluxing was done for 10 h, and progress of the reaction was monitored by 1H-NMR.
- H. tom Dieck, W. Kollvitz and I. Kleinwächter, Inorg. Chem., 1984, 23, 2685–2691 CrossRef CAS.
- J. Breuer, H.-W. Frühauf, J. J. W. Smeets and A. L. Spek, Inorg. Chim. Acta, 1999, 291, 438–447 CrossRef CAS.
- M. Menon, A. Pramanik and A. Chakravorty, Inorg. Chem., 1995, 34, 3310–3316 CrossRef CAS.
- V. Pank, J. Klaus, K. von Deuten, M. Feigel, H. Bruder and H. tom Dieck, Transition Met. Chem., 1981, 6, 185–189 CrossRef CAS.
- H.-J. Xu, X.-Y. Lu, Y. Cheng, J.-F. Sun, X.-T. Chen and Z.-L. Xue, Organometallics, 2009, 28, 6687–6694 CrossRef CAS.
-
(a) S. Goswami, A. R. Chakravarty and A. Chakravorty, Inorg. Chem., 1981, 20, 2246–2250 CrossRef CAS;
(b) R. A. Krause and K. Krause, Inorg. Chem., 1980, 19, 2600–2603 CrossRef CAS.
-
(a) B. P. Sullivan, D. J. Salmon, T. J. Meyer and J. Peedin, Inorg. Chem., 1979, 18, 3369–3374 CrossRef CAS;
(b) J. N. Braddock and T. J. Meyer, Inorg. Chem., 1973, 12, 723–727 CrossRef CAS.
- L. P. Hammett, Physical Organic Chemistry, McGraw Hill, New York, 2nd edn, 1970 Search PubMed.
- R. N. Mukherjee, O. A. Rajan and A. Chakravorty, Inorg. Chem., 1982, 21, 785–790 CrossRef CAS.
- The presence of benzaldehyde was detected as a minor product in all of the reactions reported in Table S10.† Here the yield of aldehyde was determined as 5%, 8%, 11%, 18%, 2%, and 1% for entries 1–6, respectively.
- G. R. Fulmer, A. J. M. Miller, N. H. Sherden, H. E. Gottlieb, A. Nudelman, B. M. Stoltz, J. E. Bercaw and K. I. Goldberg, Organometallics, 2010, 29, 2176–2179 CrossRef CAS.
- U. K. Das, Y. Ben-David, G. Leitus, Y. Diskin-Posner and D. Milstein, ACS Catal., 2019, 9, 479–484 CrossRef CAS.
-
(a) V. Sinha, B. Pribanic, B. d. Bruin, M. Trincado and H. Grützmacher, Chem.–Eur. J., 2018, 24, 5513–5521 CrossRef CAS PubMed;
(b) X. Yang, T. Gianetti, J. Harbort, M. D. Wçrle, L. Tan, C.-Y. Su, P. Jurt, J. R. Harmer and H. Grützmacher, Angew. Chem., 2016, 128, 12178–12181 CrossRef.
- The molar conductivity for a 1
:
1 electrolyte in ethanol is 35.0–45.0 S cm2 mol−1. -
(a) Y. Htet and A. G. Tennyson, Angew. Chem., Int. Ed. Engl., 2016, 55, 8556–8560 CrossRef CAS PubMed;
(b) A. Pal, S. Shah and S. Devi, Mater. Chem. Phys., 2009, 114, 530–532 CrossRef CAS.
-
(a) J. K. Burdett, Inorg. Chem., 1975, 14, 375–382 CrossRef CAS;
(b) M. Elian and R. Hoffmann, Inorg. Chem., 1975, 14, 1058–1076 CrossRef CAS;
(c) T. A. Albright, J. K. Burdett and M. H. Whangbo, Orbital Interactions in Chemistry, Wiley, New York, 1985 Search PubMed.
- For representative DFT studies on RuL4 species (where L = CO and PH3), see:
(a) T. Ziegler, V. Tschinke, L. Fan and A. D. Becke, J. Am. Chem. Soc., 1989, 111, 9177–9185 CrossRef CAS;
(b) J. Li, G. Schreckenbach and T. Ziegler, J. Am. Chem. Soc., 1995, 117, 486–494 CrossRef CAS;
(c) S. A. Macgregor, O. Eisenstein, M. K. Whittlesey and R. N. Perutz, Dalton Trans., 1998, 291–300 RSC.
- C. Hall, W. D. Jones, R. J. Mawby, R. Osman, R. N. Perutz and M. K. Whittlesey, J. Am. Chem. Soc., 1992, 114, 7425–7435 CrossRef CAS.
- H. Li and M. B. Hall, J. Am. Chem. Soc., 2015, 137, 12330–12342 CrossRef CAS PubMed.
-
(a) W. W. N. O, A. J. Lough and R. H. Morris, Organometallics, 2012, 31, 2137–2151 CrossRef CAS;
(b) W. W. N. O, A. J. Lough and R. H. Morris, Organometallics, 2012, 31, 2152–2165 CrossRef CAS;
(c) T. Liu, Q. Liao, M. O'Hagan, E. B. Hulley, D. L. DuBois and R. M. Bullock, Organometallics, 2015, 34, 2747–2764 CrossRef CAS;
(d) D. H. Nguyen, X. Trivelli, F. Capet, J.-F. Paul, F. Dumeignil and R. M. Gauvin, ACS Catal., 2017, 7, 2022–2032 CrossRef CAS.
-
(a) N. Govindarajan, V. Sinha, M. Trincado, H. Grützmacher, E. J. Meijer and B. d. Bruin, ChemCatChem, 2020, 12, 2610–2621 CrossRef CAS;
(b) M. Trincado, V. Sinha, R. E. Rodriguez-Lugo, B. Pribanic, B. d. Bruin and H. Grützmacher, Nat. Commun., 2017, 8, 14990 CrossRef CAS PubMed;
(c) R. E. Rodríguez-Lugo, M. Trincado, M. Vogt, F. Tewes, G. Santiso-Quinones and H. Grützmacher, Nat. Chem., 2013, 5, 342–347 CrossRef PubMed.
- I. P. Evans, A. Spencer and G. Wilkinson, J. Chem. Soc., Dalton Trans., 1973, 204–209 RSC.
-
(a) G. M. Sheldrick, SHELXS-97 and SHELXL-97, Fortran programs for crystal structure solution and refinement, University of Göttingen: Göttingen, Germany, 1997 Search PubMed;
(b) G. M. Sheldrick, A short history of SHELX, Acta Crystallogr., Sect. A: Found. Crystallogr., 2008, 64, 112–122 CrossRef CAS PubMed;
(c) O. V. Dolomanov, L. J. Bourhis, R. J. Gildea, J. A. K. Howard and H. Puschmann, OLEX2: a complete structure solution, refinement and analysis program, J. Appl. Crystallogr., 2009, 42, 339–341 CrossRef CAS.
- Y. Zhao and D. G. Truhlar, Theor. Chem. Acc., 2008, 120, 215–241 Search PubMed.
- M. J. Frisch, G. W. Trucks, H. B. Schlegel, G. E. Scuseria, M. A. Robb, J. R. Cheeseman, G. Scalmani, V. Barone, G. A. Petersson, H. Nakatsuji, X. Li, M. Caricato, A. Marenich, J. Bloino, B. G. Janesko, R. Gomperts, B. Mennucci, H. P. Hratchian, J. V. Ortiz, A. F. Izmaylov, J. L. Sonnenberg, D. Williams-Young, F. Ding, F. Lipparini, F. Egidi, J. Goings, B. Peng, A. Petrone, T. Henderson, D. Ranasinghe, V. G. Zakrzewski, J. Gao, N. Rega, G. Zheng, W. Liang, M. Hada, M. Ehara, K. Toyota, R. Fukuda, J. Hasegawa, M. Ishida, T. Nakajima, Y. Honda, O. Kitao, H. Nakai, T. Vreven, K. Throssell, J. A. Montgomery Jr, J. E. Peralta, F. Ogliaro, M. Bearpark, J. J. Heyd, E. Brothers, K. N. Kudin, V. N. Staroverov, T. Keith, R. Kobayashi, J. Normand, K. Raghavachari, A. Rendell, J. C. Burant, S. S. Iyengar, J. Tomasi, M. Cossi, J. M. Millam, M. Klene, C. Adamo, R. Cammi, J. W. Ochterski, R. L. Martin, K. Morokuma, Ö. Farkas, J. B. Foresman and D. J. Fox, Gaussian 09, Revision E.01, Gaussian, Inc., Wallingford CT, 2009 Search PubMed.
- D. Andrae, U. Haeussermann, M. Dolg, H. Stoll and H. Preuss, Theor. Chim. Acta, 1990, 77, 123–141 CrossRef CAS.
-
(a) G. A. Petersson, A. Bennett, T. G. Tensfeldt, M. A. Al-Laham, W. A. Shirley and J. Mantzaris, J. Chem. Phys., 1988, 89, 2193–2218 CrossRef CAS;
(b) G. A. Petersson and M. A. Al-Laham, J. Chem. Phys., 1991, 94, 6081–6090 CrossRef CAS.
-
(a) JIMP2, version 0.091, a free program for the visualization and manipulation of molecules: M. B. Hall and R. F. Fenske, Inorg. Chem., 1972, 11, 768–775 CrossRef CAS;
(b) J. Manson, C. E. Webster and M. B. Hall, Texas A&M University, College Station, TX, 2006, https://www.chem.tamu.edu/jimp2/index.html.
- C. Lee, W. Yang and R. G. Parr, Phys. Rev. B: Condens. Matter Mater. Phys., 1988, 37, 785–789 CrossRef CAS PubMed.
- L. E. Roy, P. J. Hay and R. L. Martin, J. Chem. Theory Comput., 2008, 4, 1029–1031 CrossRef CAS PubMed.
- M. M. Francl, W. J. Pietro, W. J. Hehre, J. S. Binkley, M. S. Gordon, D. J. Defrees and J. A. Pople, J. Chem. Phys., 1982, 77, 3654–3665 CrossRef CAS.
Footnote |
† Electronic supplementary information (ESI) available: Crystallographic data for trans-1 and cis-1 (Table S1), trans-2 and cis-2 (Table S2), trans-3 and cis-3 (Table S3), trans-4 and cis-4 (Table S4); Crystal structure of trans-1, trans-2 and trans-4 (Fig. S1); crystal structure of cis-1, cis-2 and cis-4 (Fig. S2); selected bond parameters for trans-1 and cis-1 (Table S5); selected bond parameters for trans-2 and cis-2 (Table S6); selected bond parameters for trans-3 and cis-3 (Table S7); selected bond parameters for trans-4 and cis-4 (Table S8); cyclic voltammetric data of trans-isomers and cis-isomers (Table S9); cyclic voltammogram of cis-3 (Fig. S3); least-squares plot of E½ values of Ru(II)–Ru(III) couple versus Hammett substituent constant (4σ) for the trans-isomers and the cis-isomers (Fig. S4); optimization for catalytic acceptorless dehydrogenation of alcohols (Table S10); 1H NMR of H2 evolved from benzyl alcohol (Fig. S5) and cyclohexanol (Fig. S6) oxidation; Cartesian coordinates for the optimized geometries of all the molecules in Fig. 3 and 4 along with the computed electronic and zero-point energies (Table S11). CCDC 1866863–1866870. For ESI and crystallographic data in CIF or other electronic format see DOI: https://doi.org/10.1039/d3ra04750d |
|
This journal is © The Royal Society of Chemistry 2023 |