DOI:
10.1039/D3RA04117D
(Paper)
RSC Adv., 2023,
13, 24077-24085
Fabrication and properties of Sn(IV) porphyrin-linked porous organic polymer for environmental applications†
Received
19th June 2023
, Accepted 7th August 2023
First published on 11th August 2023
Abstract
A robust porous organic polymer cross-linked by Sn(IV) porphyrin (SnPOP) was fabricated by reacting trans-dihydroxo-[5,15,10,20-tetrakis(phenyl)porphyrinato]tin(IV) (SnP) with fluorinated polyimide (FPI) via sol–gel formation, followed by supercritical CO2 drying. The structural and porous properties of SnPOP were characterized using FT-IR, UV-vis, and fluorescence spectroscopies, along with field-emission scanning electron microscopy and gas sorption experiments. The reaction between the SnP's oxophilic Sn(IV) center and FPI's carboxylic acid moiety resulted in a controllable cross-linked porous texture. This material features the desirable physical properties of porphyrin and exhibits mesoporous structures with a relatively high surface area. SnPOP is thermally stable at temperatures up to 600 °C and highly resistant to boiling water, strong acids, and bases, owing to its assembly via formation of covalent bonds instead of typically weaker hydrogen bonds. The modified chemical and morphological structures of SnPOP showed an impressive CO2 uptake capacity of 58.48 mg g−1 at 273 K, with a preference for CO2 over N2. SnPOP showed significant efficiency in removing pollutant dyes, such as methylene blue and methyl orange, from dye-contaminated water. Additionally, SnPOP was a photocatalyst for fabricating silver nanoparticles of regular shape and size. All these properties make SnPOP a potential candidate for environmental applications like pollutant removal, gas storage, and separation.
Introduction
Soft microporous materials, such as metal–organic frameworks (MOFs),1 porous coordination polymers (PCPs),2 covalent organic frameworks (COFs),3 and porous organic polymers (POPs),4 have attracted significant attention owing to their valuable features. These features include superior thermal and chemical stabilities, large accessible surface areas, insoluble backbones, tunable porosities, and various functionalities. The multidimensional properties of these materials make them good alternatives to classical porous materials, such as zeolites,5 metal oxides,6 ceramics,7 mesoporous silica,8 and activated charcoal.9 These materials have been extensively studied and utilized in various fields including heterogeneous catalysis,10 gas storage,11 biomedicine,12 gas separation,13 water purification,14 electrochemistry,15 and sensing.16 POPs, which are highly cross-linked amorphous porous materials, are typically characterized by ample molecular-scale porosity (pore diameters ranging from a few nanometers to micrometers). The porosity of these materials not only increases the catalytic activity by increasing the number of reactant-accessible catalyst sites but also adsorbs reactant molecules, thereby boosting catalytic reaction rates. Unlike MOFs, POPs are purely organic and are usually constructed using complementary pairs of rigid organic building blocks via strong covalent bonds.17–20 Among the various building blocks used in the fabrication of porous materials, porphyrin compounds are increasingly adopted because of their ability to translate building blocks into well-defined structures with regular pores. Porphyrins show excellent optical absorption properties in the visible region because of the presence of conjugated π electrons. Furthermore, they exhibit excellent absorption and electron transfer properties and are widely used in photocatalysis.21–23 Monomeric porphyrinic compounds are self-assembled into nano and microarchitectures via supramolecular noncovalent interactions, such as hydrogen bonds, π–π stacking, and van der Waals interaction. Moreover, their intriguing structures and flexible cavities allow for easy control of the active sides, facilitating the understanding of structure–property relationships.24–26 In this context, herein we report the synthesis and characterization of a porphyrin-based porous organic polymer (SnPOP) using metallated porphyrin as a cross-linker via a sol–gel route (Scheme 1).
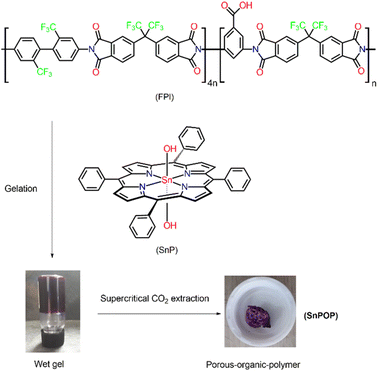 |
| Scheme 1 Synthesis of Sn(IV) porphyrin-linked porous organic polymer (SnPOP). | |
Sn(IV) porphyrin (SnP) was selected as the source of porphyrin compounds because of its unique optical properties and octahedral geometries. Owing to the oxophilic nature of the Sn(IV) center, Sn(IV) porphyrin can readily form stable six-coordinated complexes with two oxyanions (carboxylates or alkoxides) in the trans position. This advantageous tuning capability of SnPs makes them ideal scaffolds for constructing coordination complexes,27–35 nanostructures,36–44 and multiporphyrin arrays,45–47 which exhibit characteristic functions. Sn(IV)-porphyrin-based supramolecular nanostructures have been extensively studied as photocatalysts for wastewater remediation.48,49 Fluorinated polyimide (FPI) was used as the source of the organic polymer skeleton because of its chemical and physical stability. Our designed porous organic polymer (SnPOP) material utilizes SnP gelators to fabricate porous materials using the supercritical carbon dioxide extraction method.50–53 This is a promising method for fabricating porphyrin-based porous materials. Importantly, SnPOP is expected to retain the original properties of the SnPs and organic polymers to the maximum extent possible. The spectral features of SnPOP were successfully characterized, and its useful properties for environmental applications were studied. These properties include gas sorption capacities for N2 and CO2, dye removal abilities, and photocatalytic fabrication of metal nanoparticles.
Results and discussion
Syntheses and spectroscopic characterization
The design and the synthetic scheme of SnPOP are schematically illustrated in Scheme 1. Typically, a dichloromethane (DCM) solution of SnP was mixed with a dimethylformamide (DMF) solution of FPI, and the mixture was incubated in the dark to obtain a wet gel. Throughout the experiment, we made several interesting observations to optimize the reaction conditions in terms of solvent systems, reaction time, and concentration of the starting materials. Initially, solvent-dependent analysis revealed that the gel formation was successful in a DMF–DCM mixture (1
:
1), and partial gel formation occurred in DMF–THF (tetrahydrofuran). However, no gel was formed when only DMF was used. Additionally, we observed that low concentrations of starting materials (∼10−4 to 10−5 M) are best for wet gel formation. Gel formation began after 24 h, and the optimal time for complete gel formation was fixed at 72 h. After 72 h, the wet gel was immersed in DCM several times to remove DMF and unreacted SnP. Subsequently, the wet gel was dried using supercritical CO2 at high pressure and temperature to form porous SnPOP. The strong affinity of the axial hydroxyl ligand of SnP towards the carboxylic group of FPI led to the formation of a cross-linked polymer. FT-IR spectra of SnP, FPI, and SnPOP are shown in Fig. 1.
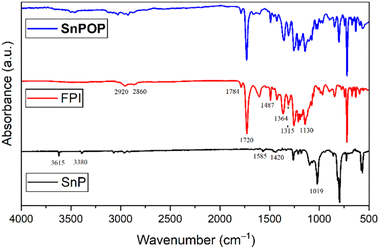 |
| Fig. 1 FT-IR spectra of SnP, FPI, and SnPOP. | |
In the case of SnP, the absorption peaks at 1019 cm−1 corresponded to the bending vibrations of C(sp2)–H in planar aromatic ring. On the other hand, out-of-plane bending vibrations of C(sp2)–H of the aromatic rings appeared at 794 cm−1. The peaks at 3380, 1585, and 1420 cm−1 were assigned to the stretching vibrations of C–H, C
C, and C–N, respectively, in the pyrrole ring. Furthermore, the peaks at 3615 cm−1 were attributed to the stretching vibrations of the O–H signal of the axial hydroxyl group in SnP. On the other hand, FPI exhibited two bands at 2920 and 2860 cm−1 belonging to the O–H stretching vibration of the carboxylic acid group. Additionally, the two bands at 1784 and 1720 cm−1 were attributed to the symmetric and asymmetric stretching vibrations of the carbonyl group of the imide ring. Furthermore, the bands at 1487 and 1364 cm−1 corresponded to the C–N stretching vibration and the aromatic ring, respectively. The characteristic bands of the CF3 group in FPI were observed in the region of 1315–1130 cm−1. The FT-IR spectrum of SnPOP clearly showed that the peak corresponding to O–H stretching at ∼3615 cm−1 completely vanished, confirming the complete reaction of the axial hydroxyl group of SnP with the carboxylic group of FPI. All other peaks remained unchanged or changed slightly compared with those of the starting components. These observations indicated the strong attachment of SnP to FPI to form SnPOP.
The solid-state UV-vis spectroscopy in Nujol was used to characterize the light absorption properties of SnP, FPI, and SnPOP (Fig. 2). SnP shows a strong light absorption peak at 428 nm corresponding to the Soret band, along with two weaker absorption peaks at 582 and 624 nm corresponding to the Q bands. On the other hand, FPI did not show any strong absorbance bands in the visible region. In comparison to SnP, SnPOP displayed a strong light absorption peak at 428 nm for the Soret band, as well as two weaker peaks at 565 and 605 nm for the Q bands. This result implied that the Q bands in SnP experienced a blue-shift, thereby confirming the strong attachment of SnP to SnPOP.
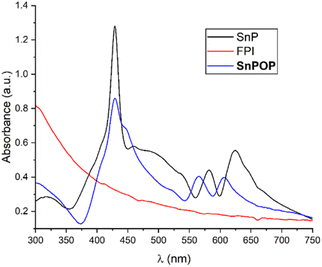 |
| Fig. 2 Solid state UV-vis absorption spectra of SnP, FPI, and SnPOP in Nujol. | |
The solid-state fluorescence spectra of SnP, FPI, and SnPOP were recorded (Fig. 3). SnP displays broad emission peaks at 602 and 650 nm at an excitation wavelength of 550 nm. FPI, however, did not show any fluorescence signal. In contrast, SnPOP exhibited emission peaks at 615 and 653 nm. The emission peak-to-peak ratio of SnP changed to that of SnPOP, suggesting a strong attachment.
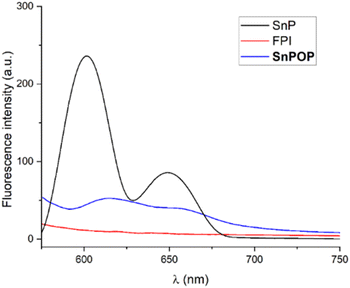 |
| Fig. 3 Solid state fluorescence (λex = 550 nm) spectra of SnP, FPI, and SnPOP in Nujol. | |
The morphological characteristics of SnPOP were evaluated by field-emission scanning electron microscopy (FE-SEM). The FE-SEM images clearly showed a two-dimensional fibrous sponge-like structure with interconnected bonds (Fig. 4).
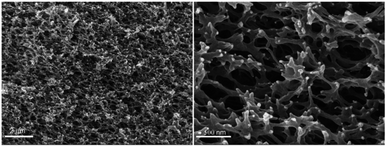 |
| Fig. 4 Low- and high-resolution FE-SEM images for SnPOP. | |
The sponges were smooth and flat, with an average length and width of approximately 400 and 50 nm, respectively. Irregularly arranged strips resembling those observed throughout the foamy sponge skeleton consisted of a classic discontinuous network of interpenetrating open and porous channels. The pore sizes of the larger scaffolds were cross-linked with an average diameter ranging between 260 and 350 nm, whereas the smaller channels had an average diameter ranging between 70 and 160 nm.
Similar to crystalline polyarylether-based COFs,54 amorphous SnPOP displays high thermal and chemical stabilities. The thermogravimetric analysis (TGA) curves of SnPOP are shown in Fig. S1.† The first change in the curve (between 100 and 200 °C) corresponded to the removal of physically adsorbed water on the surface, accounting for ∼3% of the total mass loss. Enormous weight losses (∼43%) were observed at around 612 °C, which was attributed to the elimination of surface-bound organic groups in SnPOP. These observations indicated that SnPOP was thermally stable at temperatures up to ∼600 °C. Interestingly, SnP underwent dramatic weight loss starting only at 350 °C.55 To investigate the chemical stability of SnPOP, different chemical environments, including different aqueous and organic solvents, were used to expose the SnPOP samples for 24 h. It was observed that SnPOP swelled in THF, DCM, acetone, and ethyl acetate, while retaining its gel form (Fig. S2†). In contrast, SnPOP retained its original skeleton and porous networks even after treatment with NaOH (10 M), HCl (10 M), or boiling water, as demonstrated by the morphology of SnPOP (Fig. S3a–c†). However, when immersed in aprotic polar solvents, such as DMF and DMAc, SnPOP lost its original skeleton and porous framework (Fig. S3d†).
Gas sorption
To study the porous properties of SnPOP, N2 adsorption and desorption analyses were performed at 77 K after degasification at 200 °C for 12 h to remove the adsorbed molecules. The adsorption and desorption isotherms of N2 for SnPOP are shown in (Fig. 5).
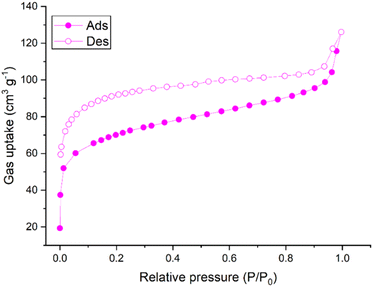 |
| Fig. 5 Adsorption and desorption isotherms of N2 for SnPOP at 77 K. | |
The N2 sorption isotherms of SnPOP exhibited a combination of type I and II isotherms, along with strong adsorption at low pressures (P/P0 < 0.1), suggesting that mesopores and micropores coexist in the framework. The BET-specific surface area of SnPOP was calculated to be 227.2 m2 g−1. To further confirm the pore size of SnPOP, the pore size distribution (PSD) plots calculated from the adsorption branch of the isotherms using the Non-local density functional theory (NLDFT) method are shown in Fig. S2.† The PSD of SnPOP was mostly centered at ∼2.04 nm. The specific surface area value of SnPOP was determined using the relative pressure (P/P0) range of 0.01–0.10.
Considering the high porosity, we studied the potential applications of SnPOP for CO2 adsorption and separation. The adsorption isotherms of CO2, and N2 were measured at 273 and 298 K, respectively. As shown in Fig. 6, the sorption amounts of CO2 are as high as 31.85 cm3 g−1 (58.48 mg g−1) at 273 K and 19.89 cm3 g−1 (36.52 mg g−1) at 298 K.
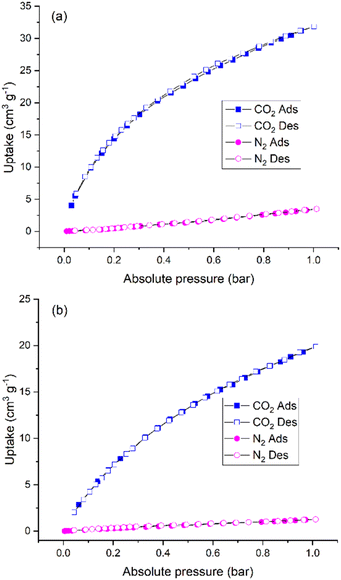 |
| Fig. 6 CO2 and N2 sorption isotherms for SnPOP at 273 K (a) and 298 K (b). | |
All sorption isotherms revealed moderate CO2 adsorption, comparable to those reported for porphyrin-based porous materials (Table S1†). Meanwhile, the CO2 uptake is much higher than N2 uptake (3.48 cm3 g−1 or 4.35 mg g−1 at 273 K, and 1.27 cm3 g−1 or 1.59 mg g−1 at 298 K). The isotherms for both gases at 273 and 298 K demonstrated no uptake in the low-concentration region, but gradually increased adsorption at higher concentrations. We further calculated the CO2/N2 selectivity based on the experimental conditions. The ideal adsorption selectivity of SnPOP was calculated using the following equation:56
αabs12 = (Nads1/Nads2)(y2/y1) |
where
N is the absorbed amount,
y is the mole fraction,
α is the ideal adsorption selectivity coefficient, and subscripts 1 and 2 indicate stronger (CO
2) and weaker absorbent (N
2), respectively.
For example, the ideal adsorption selectivity of SnPOP was found to be as high as 21.2 for CO2/N2 at 273 K and 23.1 for CO2/N2 at 298 K. The selectivity of SnPOP at similar temperatures was comparable to or higher than that of recently reported porphyrin-based porous materials. The absorption isotherms clearly showed that SnPOP selectively absorbed CO2 over N2,13,57,58 which can be attributed to the dipole–quadrupole interaction between the organic functional groups present in SnPOP and the guest CO2 molecules. The dispersion force within CO2 molecules was significantly affected by their quadrupole moment or polarizability.
Dye adsorption
To assess the adsorption capacity of SnPOP for bulky molecule transportation, azo dyes were selected. Specifically, we selected the anionic dye methyl orange (MO) and cationic dye methylene blue (MB). Approximately 20 mg of SnPOP was mixed with 100 mL aqueous solution of dye (50 mg L−1) and continuously stirred for 5 h at room temperature in the dark. Subsequently, the solid particles were separated from the solution through centrifugation. The residual concentrations of the dyes were determined by UV-vis spectroscopy after dilution, and calculated according to the standard curve. It can be seen in Fig. 7 and 8, SnPOP exhibited outstanding adsorption both for MB and MO with removal efficiencies of 75% and 70%, respectively. As shown in Fig. S3 and S4,† SnPOP showed a higher uptake capacity of cationic dye (187.5 mg g−1 for MB) compared to anionic dye (175 mg g−1 for MO). These results demonstrate that the porphyrin-linked porous SnPOP materials have a fairly high capability for dye adsorption and are comparable to reported porphyrin-based porous materials (Table S2†).
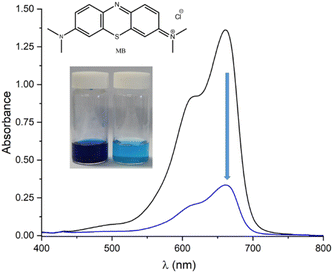 |
| Fig. 7 UV-vis absorption spectral change of methylene blue (MB) in water upon SnPOP treatment. | |
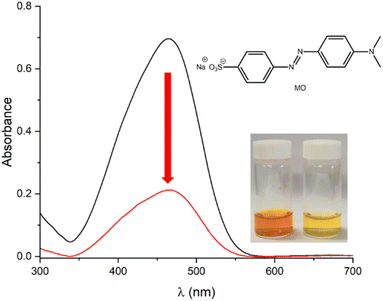 |
| Fig. 8 UV-vis absorption spectral change of methyl orange (MO) in water upon SnPOP treatment. | |
Photocatalytic fabrication of Ag nanoparticles
Currently, silver nanostructures have received considerable attention because of their reduced density, increased surface area, and unique electronic and optical properties, which have led to a wide range of important applications in biosensing,59 catalysis,60 photochemistry,61 and photodynamic therapy.62 Among others, the photochemical method is the most suitable process for controlling the morphology of metal nanostructures and their tunable properties for enhanced applications. In this method, researchers use the direct chemical reduction of a metal precursor to fabricate metal nanostructures.63 Occasionally, photosensitizers are used as intermediate species.64 Shelnutt et al. observed that SnP can reduce Pt(II) ion species to Pt nanoparticles (NPs) with well-defined shapes and uniform sizes under visible light irradiation.65 Additionally, Pereira et al. reported a facile photoredox process for fabricating Au and Ag NPs from a water-soluble Sn(IV) porphyrin complex.66 Inspired by previous observations, we used SnPOP as a photoredox catalyst to fabricate Ag NPs under visible light irradiation.
The photocatalytic method described in Scheme 2 was used to synthesize Ag NPs. The photocatalyst used in this study was the Sn(IV) porphyrin-linked porous polymer, SnPOP. Photocatalytic reduction of silver salts occurred in the presence of visible light and the electron donor triethylamine (TEA). The absorption of visible light by SnPOP yields a long-lived excited triplet π–π* state, SnPOP*, which is rapidly reduced by an ED such as TEA. The resulting product is a long-lived radical ion, SnPOP−˙, which is a strong reductant capable of effectively reducing Ag+ to Ag0. The reduction of the metal regenerates neutral SnPOP, which can then absorb light and initiate a successive photochemical cycle. The photocatalytic cycle is reductive because the initial electron transfer event involves the reduction of the porphyrin anion by TEA. It is important to note that we did not use any capping agent like poly(N-vinyl pyrrolidone) (PVP) or any surfactants.53,55 The use of surfactants such as sodium dodecyl sulphate (SDS) makes it difficult to separate Ag NPs from the reaction mixture. We examined the optical and surface properties of the as-prepared Ag NPs using UV-vis spectrophotometry, FE-SEM/EDS, and TEM.
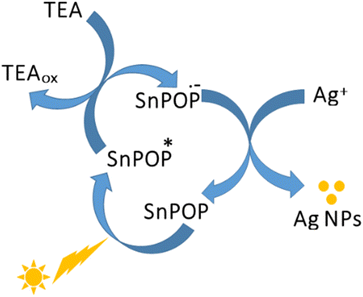 |
| Scheme 2 Photocatalytic reaction cycle for the synthesis of silver nanoparticles (Ag NPs) from the silver cations in the presence of SnPOP at room temperature. | |
The surface morphology and size of the synthesized Ag NPs on SnPOP were observed by FE-SEM and the images are shown in Fig. S4.† The Ag NPs exhibited regular and homogeneous structures, with almost spherical shapes and diameters of 15–60 nm (Fig. S5†). Additionally, agglomerated growth of Ag NPs was observed, with an estimated particle size ranging from 150 to 400 nm and clear boundaries. To confirm the formation of the synthesized Ag NPs energy-dispersive X-ray spectroscopy (EDX) was performed. The EDX analysis was performed to examine the elemental composition, homogeneity, and distribution of the as-prepared Ag NPs. As shown in Fig. S6,† the EDX spectrum confirms the presence of Ag NPs on SnPOP. A strong signal in the Ag region is evident, indicating the formation or creation of Ag NPs at approximately 3.1 keV. Furthermore, the presence of weak absorption signals for carbon and oxygen were observed at approximately 0.24 and 0.49 keV, respectively.
Experimental
Materials and methods
All commercial chemicals were used as received, unless otherwise specified. Toluene and pyrrole were distilled from the calcium hydride solution. The synthesis of trans-dihydroxo-[5,15,10,20-tetraphenylporphyrinato]tin(IV) (SnP) followed a previously reported procedure.67 FPI was synthesized according to the reported procedure.68 Elemental analysis was performed using a ThermoQuest EA 1110 analyzer. Steady-state UV-vis spectra were recorded using a Shimadzu UV-3600 spectrophotometer (Shimadzu, Tokyo, Japan). Fluorescence spectra were recorded using a Shimadzu RF-5301PC fluorescence spectrophotometer (Shimadzu, Tokyo, Japan). Fourier transform infrared (FT-IR) spectra (KBr) were obtained using a Shimadzu FTIR-8400S spectrophotometer (Shimadzu, Tokyo, Japan). Thermogravimetric analysis (TGA) was performed using an Auto-TGA Q500 instrument (TA Instruments, New Castle, DE, USA) under nitrogen. The Brunauer–Emmett–Teller (BET) surface area was determined with an analyzer (BELSORP-mini volumetric adsorption equipment) using N2 adsorption isotherms at 77 K. Surface and pore size data were obtained using Autosorb-iQ and Quadrasorb SI. Field-emission scanning electron microscopy (FE-SEM) images were obtained using a MAIA III instrument (TESCAN, Brno, Czech Republic).
Synthesis of Sn(IV) porphyrin-linked porous organic polymer
A 5 mL dichloromethane (DCM) solution of SnP (6.5 × 10−5 M) was mixed with 5 mL of dimethylformamide (DMF) solution of FPI (1.3 × 10−4 M) in a sample vial. The solution was kept in a dark at room temperature (298 K) for gelation. After 3 days, a wet gel was formed. The wet gels were then subjected to solvent exchange for 24 h to remove DMF and unreacted SnP three times in succession by immersing in DCM. Subsequently, the wet gel was dried using supercritical CO2 at 333 K and 100 bar for 6 h. After the autoclave was depressurized slowly at room temperature for about 6 h, a SnPOP was obtained.
Photocatalytic fabrication of Ag nanoparticles using Sn(IV) porphyrin-linked porous organic polymer
A 5 mL acetonitrile solution of silver nitrate (9 mM) and TEA was mixed in a 20 mL glass vial. To this solution, 50 mg SnPOP was added, and the reaction system was subjected to stirring at room temperature while being irradiated with a xenon bulb at a power of 50 W for 1 h. At the end of the reaction, the color of the SnPOP changed from purple to blue, suggesting the formation of Ag nanoparticles. The solution was decanted from the reaction mixture and the product was dried in vacuo.
Conclusions
We developed a robust SnPOP by reacting SnP with FPI. The fabrication process of SnPOP consists of two steps. First, a wet gel was formed by reacting SnP with FPI in DMF-DCM at room temperature. In the second step, the wet gel was transformed into SnPOP with high porosity through extraction with supercritical CO2. The reaction between the oxophilic Sn(IV) center in SnP and the carboxylic acid functional group of FPI resulted in a controllable cross-linked porous texture. More importantly, the intrinsic properties of SnP and organic polymer were likely to be maximally retained in SnPOP. SnPOP is thermally stable up to 600 °C and extremely resistant to various chemical environments, including boiling water, strong acids, and bases. This is because of the covalent bond formation during the assembly of SnPOP, which is stronger than the typically weaker hydrogen bonds. Moreover, this material features the attractive properties of porphyrins and exhibits mesoporous structures with a relatively high surface area. The modified chemical and morphological structures in SnPOP showed an impressive CO2 uptake capacity of 58.48 mg g−1 at 273 K and enable preferential CO2 uptake over N2. SnPOP showed a significant ability to remove pollutant dyes, such as MB and MO, from dye-contaminated water. In addition, SnPOP has been used as a photocatalyst for the fabrication of Ag nanoparticles with regular shape and size. In conclusion, our report on Sn(IV) porphyrin-linked organic polymers provides valuable insights into the development of various stable functional materials with high porosity for environmental applications, such as pollutant removal, gas storage, and separation.
Author contributions
Data curation, investigation, methodology, C.-J. L.; formal analysis, software, validation, visualization, and writing, N. K. S.; conceptualization, funding acquisition, project administration, review, resources, supervision, validation, and editing, H.-J. K.
Conflicts of interest
There are no conflicts to be declared.
Acknowledgements
This study was supported by the National Research Foundation of Korea (NRF) (grant no. 2022R1F1A1074420) funded by the Korean government (MSIT).
References
- H. Li, M. Eddaoudi, M. O'Keeffe and O. M. Yaghi, Design and synthesis of an exceptionally stable and highly porous metal–organic framework, Nature, 1999, 402, 276–279 CrossRef CAS
. - S. Kitagawa, R. Kitaura and S. I. Noro, Functional porous coordination polymers, Angew. Chem., Int. Ed., 2004, 43, 2334–2375 CrossRef CAS PubMed
. - A. P. Côté, A. I. Benin, N. W. Ockwig, M. O'Keeffe, A. I. Matzger and O. M. Yaghi, Porous, Crystalline, Covalent Organic Frameworks, Science, 2005, 310, 1166–1170 CrossRef PubMed
. - P. Kaur, J. T. Hupp and S. T. Nguyen, Porous Organic Polymers in Catalysis: Opportunities and Challenges, ACS Catal., 2011, 1, 819–835 CrossRef CAS
. - Z. Cao, X. Cai, A. C. Feltrin, P. Feng, A. Kaiser and F. Akhtar, Calcium/strontium chloride impregnated zeolite A and X granules as optimized ammonia sorbents, RSC Adv., 2022, 12, 34910–34917 RSC
. - L. Wang, C. Shi, L. Pan, X. Zhang and J.-J. Zou, Rational design, synthesis, adsorption principles and applications of metal oxide adsorbents: a review, Nanoscale, 2020, 12, 4790–4815 RSC
. - Z. Yang, H. Liang, L. Jin and D. Poelman, High-performance lead-free bulk ceramics for electrical energy storage applications: design strategies and challenges, J. Mater. Chem. A, 2021, 9, 18026–18085 RSC
. - M. Iranshahy, M. Y. Hanafi-Bojd, S. H. Aghili, M. Iranshahi, S. M. Nabavi, S. Saberi, R. Filosa, I. F. Nezhad and M. Hasanpour, Curcumin-loaded mesoporous silica nanoparticles for drug delivery: synthesis, biological assays and therapeutic potential – a review, RSC Adv., 2023, 13, 22250–22267 RSC
. - L. Yang, W. Yungang, L. Tao, Z. Li, B. Yanyuan and X. Haoran, High-performance sorbents from ionic liquid activated walnut shell carbon: an investigation of adsorption and regeneration, RSC Adv., 2023, 13, 22744–22757 RSC
. - C. Perego and R. Millinib, Porous Materials in Catalysis: Challenges for Mesoporous Materials, Chem. Soc. Rev., 2013, 42, 3956–3976 RSC
. - H. Li, L. Li, R.-B. Lin, W. Zhou, Z. Zhang, S. Xiang and B. Chen, Porous Metal-Organic Frameworks for Gas Storage and Separation: Status and Challenges, EnergyChem, 2019, 1, 100006 CrossRef
. - S. K. Das, S. Mishra, K. D. Saha, D. Chandra, M. Hara, A. A. Mostafa and A. Bhaumik, N-Rich, Polyphenolic Porous Organic Polymer and Its In Vitro Anticancer Activity on Colorectal Cancer, Molecules, 2022, 27, 7326 CrossRef CAS PubMed
. - N. K. Shee, C.-J. Lee and H.-J. Kim, Hexacoordinated Sn(IV) porphyrin-based square-grid frameworks exhibiting selective uptake of CO2 over N2, Bull. Korean Chem. Soc., 2022, 43, 103–109 CrossRef CAS
. - N. K. Shee, H. J. Jo and H.-J. Kim, Coordination framework materials fabricated by the self-assembly of Sn(IV) porphyrins with Ag(I) ions for the photocatalytic degradation of organic dyes in wastewater, Inorg. Chem. Front., 2022, 9, 1270–1280 RSC
. - D. H. Yang, Y. Tao, X. Ding and B. H. Han, Porous Organic Polymers for Electrocatalysis, Chem. Soc. Rev., 2022, 51, 761–791 RSC
. - Z.-W. Li, B. Tan, Z.-F. Wu and X.-Y. Huang, A Robust Strontium Coordination Polymer with Selective and Sensitive Fluorescence Sensing Ability for Fe3+ Ions, Materials, 2023, 16, 577 CrossRef CAS PubMed
. - Y. Zhang and S. N. Riduan, Functional porous organic polymers for heterogeneous catalysis, Chem. Soc. Rev., 2012, 41, 2083–2094 RSC
. - M. Inukai, M. Tamura, S. Horike, M. Higuchi, S. Kitagawa and K. Nakamura, Storage of CO2 into Porous Coordination Polymer Controlled by Molecular Rotor Dynamics, Angew. Chem., Int. Ed., 2018, 57, 8687–8690 CrossRef CAS PubMed
. - T. Zhang, G. Xing, W. Chen and L. Chen, Porous organic polymers: a promising platform for efficient photocatalysis, Mater. Chem. Front., 2020, 4, 332–353 RSC
. - Z. Xu, L. Hu, J. Ming, X. Cui, M. Zhang, J. Dou, W. Zhang and B. Zhou, Self-Gated Porous Organic Polymer as Drug Delivery System for PH Stimuli-Responsive Controlled Quercetin Release, Microporous Mesoporous Mater., 2020, 303, 110259 CrossRef CAS
. - X. W. Jiang, Y. F. Liu, J. Liu, Y. L. Luo and Y. Lyu, Facile synthesis of porous organic polymers bifunctionalized with azo and porphyrin groups, RSC Adv., 2015, 5, 98508–98513 RSC
. - T. Hasobe, Supramolecular nanoarchitectures for light energy conversion, Phys. Chem. Chem. Phys., 2010, 12, 44–57 RSC
. - A. M. Kobaisy, M. F. Elkady, A. A. Abdel-Moneim and M. E. El-Khouly, Surface-decorated porphyrinic zirconium-based metal–organic frameworks (MOFs) using post-synthetic self-assembly for photodegradation of methyl orange dye, RSC Adv., 2023, 13, 23050–23060 RSC
. - I. Beletskaya, V. S. Tyurin, A. Y. Tsivadze, R. Guilard and C. Stern, Supramolecular chemistry of metalloporphyrins, Chem. Rev., 2009, 109, 1659–1713 CrossRef CAS PubMed
. - S. Durot, J. Taesch and V. Heitz, Multiporphyrinic Cages: Architectures and Functions, Chem. Rev., 2014, 114, 8542–8578 CrossRef CAS PubMed
. - N. K. Shee, J.-W. Seo and H.-J. Kim, Spectrophotometric Study of Bridging N-Donor Ligand-Induced Supramolecular Assembly of Conjugated Zn-Trisporphyrin with a Triphenylamine Core, Molecules, 2021, 26, 4771 CrossRef CAS PubMed
. - H. J. Kim, K.-M. Park, T. K. Ahn, S. K. Kim, K. S. Kim, D. Kim and H.-J. Kim, Novel fullerene–porphyrin–fullerene triad linked by metal axial coordination: Synthesis, X-ray crystal structure, and spectroscopic characterizations of trans-bis([60]fullerenoacetato)tin(iv) porphyrin, Chem. Commun., 2004, 2594–2595 RSC
. - H.-J. Kim, H. J. Jo, J. Kim, S.-Y. Kim, D. Kim and K. Kim, Supramolecular self-assembly of tin(IV) porphyrin channels stabilizing single-file chains of water molecules, CrystEngComm, 2005, 7, 417–420 RSC
. - H. J. Kim, W. S. Jeon, J. H. Lim, C. S. Hong and H.-J. Kim, Synthesis, X-ray crystal structure, and electrochemistry of trans-bis(ferrocenecarboxylato)(tetraphenylporphyrinato)tin(IV), Polyhedron, 2007, 26, 2517–2522 CrossRef CAS
. - H. J. Kim, J. H. Jang, H. Choi, T. Lee, J. Ko, M. Yoon and H.-J. Kim, Photoregulated Fluorescence Switching in Axially-Coordinated Tin(IV) Porphyrinic Dithienylethene, Inorg. Chem., 2008, 47, 2411–2415 CrossRef CAS PubMed
. - W. Kim, T. Tachikawa, T. Majima, C. Li, H.-J. Kim and W. Choi, Tin-porphyrin sensitized TiO2 for the production of H2 under visible light, Energy Environ. Sci., 2010, 3, 1789–1795 RSC
. - H. J. Jo, S. H. Kim and H.-J. Kim, Supramolecular Assembly of Tin(IV) Porphyrin Cations Stabilized by Ionic Hydrogen-Bonding Interactions, Bull. Korean Chem. Soc., 2015, 36, 2348–2351 CrossRef CAS
. - N. K. Shee, M. K. Kim and H.-J. Kim, Fluorescent chemosensing for aromatic compounds by supramolecular complex composed of tin(IV) porphyrin, viologen, and cucurbit[8]uril, Chem. Commun., 2019, 55, 10575–10578 RSC
. - M. K. Kim, N. K. Shee, J. Lee, M. Yoon and H.-J. Kim, Photoinduced Electron Transfer upon Supramolecular Complexation of (Porphyrinato) Sn-Viologen with Cucurbit [7] uril, Photochem. Photobiol. Sci., 2019, 18, 1996–2002 CrossRef CAS PubMed
. - N. K. Shee, C.-J. Lee and H.-J. Kim, Crystal structure of bis (benzoato-κO)[5,15-di-phenyl-10,20-bis(pyridin-4-yl)porphyrinato-κ4N,N′,N′′,N′′′]tin(IV), IUCrData, 2019, 4, x190787 CrossRef CAS
. - S. J. Lee, R. A. Jensen, C. D. Malliakas, M. G. Kanatzidis, J. T. Hupp and S. T. Nguyen, Effect of secondary substituent on the physical properties, crystal structures, and nanoparticle morphologies of (porphyrin)Sn(OH)2: diversity enabled via synthetic manipulations, J. Mater. Chem., 2008, 18, 3640–3642 RSC
. - C. J. Medforth, Z. Wang, K. E. Martin, Y. Song, J. L. Jacobsen and J. A. Shelnutt, Self-assembled porphyrin nanostructures, Chem. Commun., 2009, 7261–7277 RSC
. - C. Li, K.-M. Park and H.-J. Kim, Ionic assembled hybrid nanoparticle consisting of tin(IV) porphyrin cations and polyoxomolybdate anions, and photocatalytic hydrogen production by its visible light sensitization, Inorg. Chem. Commun., 2015, 60, 8–11 CrossRef CAS
. - N. K. Shee, M. K. Kim and H.-J. Kim, Supramolecular Porphyrin Nanostructures Based on Coordination-Driven Self-Assembly and Their Visible Light Catalytic Degradation of Methylene Blue Dye, Nanomaterials, 2020, 10, 2314 CrossRef CAS PubMed
. - N. K. Shee and H.-J. Kim, Self-Assembled Nanomaterials Based on Complementary Sn(IV) and Zn(II)-Porphyrins, and Their Photocatalytic Degradation for Rhodamine B Dye, Molecules, 2021, 26, 3598 CrossRef CAS PubMed
. - N. K. Shee and H.-J. Kim, Morphology-controlled self-assembled nanostructures of complementary metalloporphyrin triads through intermolecular coordination tuning and their photocatalytic degradation for Orange II, Inorg. Chem. Front., 2022, 9, 5538–5548 RSC
. - N. K. Shee and H.-J. Kim, Sn(IV)-Porphyrin-Based Nanostructures Featuring Pd(II)-Mediated Supramolecular Arrays and Their Photocatalytic Degradation of Acid Orange 7 Dye, Int. J. Mol. Sci., 2022, 23, 13702 CrossRef CAS PubMed
. - S. H. Kim and H.-J. Kim, Photocatalytic Hydrogen Production by the Sensitization of Sn(IV)-Porphyrin Embedded in a Nafion Matrix Coated on TiO2, Molecules, 2022, 27, 3770 CrossRef CAS PubMed
. - N. K. Shee and H.-J. Kim, Supramolecular squares of Sn(iv)porphyrins with Re(i)-corners for the fabrication of self-assembled nanostructures performing photocatalytic degradation of Eriochrome Black T dye, Inorg. Chem. Front., 2023, 10, 174–183 RSC
. - H.-J. Kim, N. Bampos and J. K. M. Sanders, Assembly of Dynamic Heterometallic Oligoporphyrins Using Cooperative Zinc-Nitrogen, Ru-Nitrogen and Tin-Oxygen Coordination, J. Am. Chem. Soc., 1999, 121, 8120–8121 CrossRef CAS
. - M. T. Indelli, C. Chiorboli, M. Ghirotti, M. Orlandi, F. Scandola, H. J. Kim and H.-J. Kim, Photoinduced Electron Transfer in Ruthenium(II)/Tin(IV) Multiporphyrin Arrays, J. Phys. Chem. B, 2010, 114, 14273–14282 CrossRef CAS PubMed
. - H. J. Kim, N. K. Shee, K. M. Park and H.-J. Kim, Assembly and X-ray crystal structures of heterometallic multiporphyrins with complementary coordination between ruthenium(II) and tin(IV) porphyrins, Inorg. Chim. Acta, 2019, 488, 1–7 CrossRef CAS
. - N. K. Shee and H.-J. Kim, Three Isomeric Zn(II)-Sn(IV)-Zn(II) Porphyrin-Triad-Based Supramolecular Nanoarchitectures for the Morphology-Dependent Photocatalytic Degradation of Methyl Orange, ACS Omega, 2022, 7, 9775–9784 CrossRef CAS PubMed
. - N. K. Shee and H.-J. Kim, Sn(IV) Porphyrin-Based Ionic Self-Assembled Nanostructures and Their Application in Visible Light Photo-Degradation of Malachite Green, Catalysts, 2022, 12, 799 CrossRef CAS
. - X. Zhao, L. Yuan, Z.-Q. Zhang, Y.-S. Wang, Q. Yu and J. Li, Synthetic Methodology for the Fabrication of Porous Porphyrin Materials with Metal–Organic–Polymer Aerogels, Inorg. Chem., 2016, 55, 5287–5296 CrossRef CAS PubMed
. - X. Xu, Q. Yu, D. Zhao, W. Zhang, N. Wang and J. Li, Synthesis and characterization of porphyrin-based porous coordination polymers obtained by supercritical CO2 extraction, J. Mater. Sci., 2018, 53, 10534–11054 CrossRef
. - W. Zhang, J. J. Dynes, Y. Hu, P. Jiang and S. Ma, Porous metal-metalloporphyrin gel as catalytic binding pocket for highly efficient synergistic catalysis, Nat. Commun., 2019, 10, 1913 CrossRef PubMed
. - Y. Keum, B. Kim, A. Byun and J. Park, Synthesis and Photocatalytic Properties of Titanium-Porphyrinic Aerogels, Angew. Chem., Int. Ed., 2020, 59, 21591–21596 CrossRef CAS PubMed
. - X. Guan, H. Li, Y. Ma, M. Xue, Q. Fang, Y. Yan, V. Valtchev and S. Qiu, Chemically stable polyarylether-based covalent organic frameworks, Nat. Chem., 2019, 11, 587–594 CrossRef CAS PubMed
. - N. K. Shee, B.-H. Park and H.-J. Kim, Hybrid Composite of Sn(IV)-Porphyrin and Mesoporous Structure for Enhanced Visible Light Photocatalytic Degradation of Organic Dyes, Molecules, 2023, 28, 1886 CrossRef CAS PubMed
. - Y. S. Bae and R. Q. Snurr, Development and evaluation of porous materials for carbon dioxide separation and capture, Angew. Chem., Int. Ed., 2011, 50, 11586–11596 CrossRef CAS PubMed
. - Z. Wang, S. Yuan, A. Mason, B. Reprogle, D. J. Liu and L. Yu, Nanoporous porphyrin polymers for gas storage and separation, Macromolecules, 2012, 45, 7413–7419 CrossRef CAS
. - N. Huang, R. Krishna and D. Jiang, Tailor-Made Pore Surface Engineering in Covalent Organic Frameworks: Systematic Functionalization for Performance Screening, J. Am. Chem. Soc., 2015, 137, 7079–7082 CrossRef CAS PubMed
. - W. S. T. Tun, S. Kulchat, C. Talodthaisong, S. Daduang, J. Daduang, K. Rongchai and R. Patramanon, A machine learning colorimetric biosensor based on acetylcholinesterase and silver nanoparticles for the detection of dichlorvos pesticides, Mater. Chem. Front., 2022, 6, 1487–1498 RSC
. - A. Shen, R. Luo, X. Liao, C. He and Y. Li, Highly dispersed silver nanoparticles confined in a nitrogen-containing covalent organic framework for 4-nitrophenol reduction, Mater. Chem. Front., 2021, 5, 6923–6930 RSC
. - D. Gola, A. Kriti, N. Bhatt, M. Bajpai, A. Singh, A. Arya, N. Chauhan, S. K. Srivastava, P. K. Tyagi and Y. Agrawal, Silver nanoparticles for enhanced dye degradation, Curr. Res. Green Sustainable Chem., 2021, 4, 100132 CrossRef CAS
. - M. T. Yaraki, Y. Pan, F. Hu, Y. Yu, B. Liu and Y. N. Tan, Nanosilver-enhanced AIE photosensitizer for simultaneous bioimaging and photodynamic therapy, Mater. Chem. Front., 2020, 4, 3074–3085 RSC
. - H. Wang, X. Qiao, J. Chen and S. Ding, Preparation of silver nanoparticles by chemical reduction method, Colloids Surf., A, 2005, 256, 111–115 CrossRef CAS
. - F. Bai, Z. Sun, H. Wu, R. E. Haddad, X. Xiao and H. Fan, Templated Photocatalytic Synthesis of Well-Defined Platinum Hollow Nanostructures with Enhanced Catalytic Performance for Methanol Oxidation, Nano Lett., 2011, 11, 3759–3762 CrossRef CAS PubMed
. - Y. Song, Y. Yang, C. J. Medforth, E. Pereira, A. K. Singh, H. Xu, Y. Jiang, C. J. Brinker, F. van Swol and J. A. Shelnutt, Controlled synthesis of 2-D and 3-D dendritic platinum nanostructures, J. Am. Chem. Soc., 2004, 126, 635–645 CrossRef CAS PubMed
. - P. Quaresma, L. Soares, L. Contar, A. Miranda, I. Osorio, P. A. Carvalho, R. Franco and E. Pereira, Green photocatalytic synthesis of stable Au and Ag nanoparticles, Green Chem., 2009, 11, 1889–1893 RSC
. - H. J. Jo, S. H. Jung and H.-J. Kim, Synthesis and Hydrogen-Bonded Supramolecular Assembly of trans-Dihydroxotin(IV) Tetrapyridylporphyrin Complexes, Bull. Korean Chem. Soc., 2004, 25, 1869–1873 CrossRef CAS
. - H. Q. Pham, G. Kim, H. M. Jung and S.-W. Song, Fluorinated Polyimide as a Novel High-Voltage Binder for High-Capacity Cathode of Lithium-Ion Batteries, Adv. Funct. Mater., 2018, 28, 1704690 CrossRef
.
|
This journal is © The Royal Society of Chemistry 2023 |
Click here to see how this site uses Cookies. View our privacy policy here.