DOI:
10.1039/D3RA03444E
(Paper)
RSC Adv., 2023,
13, 23590-23600
Polarizability characteristics of twisted bilayer graphene quantum dots in the absence of periodic moiré potential
Received
23rd May 2023
, Accepted 1st August 2023
First published on 7th August 2023
Abstract
Recent studies have documented a rich phenomenology in twisted bilayer graphene (TBG), which is significantly relevant to interlayer electronic coupling, in particular to the cases under an applied electric field. While polarizability measures the response of electrons against applied fields, this work adopts a unique strategy of decomposing global polarizability into distributional contributions to access the interlayer polarization in TBG, as a function of varying twisting angles (θ). Through the construction of a model of twisted graphene quantum dots, we assess distributional polarizability at the first-principles level. Our findings demonstrate that the polarizability perpendicular to the graphene plates can be decomposed into intralayer dipoles and interlayer charge-transfer (CT) components, the latter of which provides an explicit measurement of the interlayer coupling strength and charge transfer potential. Our analysis further reveals that interlayer polarizability dominates the polarizability variation during twisting. Intriguingly, the largest interlayer polarizability and CT driven by an external field occur in the misaligned structures with a size-dependent small angle corresponding to the first appearance of AB stacking, rather than the well-recognized Bernal structures. A derived equation is then employed to address the size dependence on the angle corresponding to the largest values in interlayer polarizability and CT. Our investigation not only characterizes the CT features in the interlayer polarizability of TBG quantum dots, but also sheds light on the existence of the strongest interlayer coupling and charge transfer at small twist angles in the presence of an external electric field, thereby providing a comprehensive understanding of the novel properties of graphene-based nanomaterials.
1. Introduction
Graphene-based geometries, including monolayers, bilayers, and multilayers, have great potential for use in photoelectromagnetic devices due to their attractive properties.1–3 Intriguingly, the properties of bilayer graphene (BG) change profoundly at a relative twist angle between two component layers, so-called twisted bilayer graphene (TBG), resulting in a variety of interesting phenomena of superconductivity,4–6 correlated insulators,7,8 moiré bands and Hofstadter's butterfly spectra,9 van Hove singularities (VHSs),10 etc. These unique properties are highly relevant to the strong interlayer coupling, which has been expounded in depth through numerous theoretical investigations.11–13 Interlayer rotation, leading to various stacking patterns to form periodic moiré potential, significantly affects the interlayer coupling and thus determines the physical properties of TBG. However, many applications of bulk graphene are thwarted by possessing a zero bandgap.14–16 Actually, when 2D graphene sheets are converted into 0D quantum dots (QDs), to a certain extent, it extends varieties of characteristic properties and new phenomena owing to the remarkable size- and shape-dependent energy levels in electronic structure and optical absorption or emission spectra induced by the quantum confinement and edge effects.17–19
Recent advances in the exploration of graphene quantum dots (GQDs) have indicated their extensive potential as distinctive physicochemical and biological entities, serving as a novel platform in various fields including nanomedicine, biotechnology, cancer therapy and quantum information processing,20–23 etc. Specifically, GQDs, owing to their excellent biocompatibility, are ideal imaging probes in diverse modalities of bioimaging, such as fluorescence, in vivo optical and nuclear magnetism imaging.24,25 In addition, compounded with rapid response of photoluminescence-based GQDs and photostability, GQDs have emerged as promising biosensors for ion and small molecule detection, and as diagnostic platforms platform.26,27 Furthermore, GQDs exhibit high photothermal conversion ability in addition to intrinsic photoluminescence properties, enables their application in tumor imaging and tracking in cancer therapy.28 The small size of GQDs facilitates their rapid clearance from the body, thereby demonstrating excellent biosafety.29 Varieties of functional groups on the edge of GQDs can be integrated with therapeutics and targeting ligands. Moreover, the sp2-hybridized carbon in the lattice allows therapeutic molecules to be restricted to the planes of the GQDs, which promotes the drug loading capacity and enhances the efficiency of drug release. It can be thus applied to biosensing, including photodynamic therapy, drug delivery and gene delivery.28,30,31 In particular, considerable attention has been focused on the development of efficient radiosensitizers with low toxicity in radiation therapy (RT). Radiosensitizer can release the hypoxia in the tumor area through augment the reactive oxygen species (ROS) formation and DNA damages that significantly enhance the radiation treatment. Nevertheless, the clinical application of many conventional metal radiosensitizers with a high atomic number such as gold and gadolinium nanomaterials still faces many obstacles, particularly the high preparation cost, complicated purification technology and systemic toxicity.32 Recently, the non-metal GQDs with highly augmented photoluminescence properties and biocompatibility has received remarkable scientific attentions as a promising radiosensitizer in the field of RT. Abundant surface oxygen-containing groups facilitate the overproduction of ROS and mitochondrial damage, thereby promoting the radiation response.33,34 Consequently, constructing, functionalizing, and doping GQDs are essential for their impact on biological, electronic, magnetic, and optical properties.
Numerous investigations have been conducted on GQDs-based systems with a focus on their diverse properties of monolayer and bilayer graphene quantum dots (BG QDs).2,3,19,24,35–37 The manipulation of the particular properties of these materials can be achieved through various means, such as altering and controlling the relative twist between two stacked GQD flakes, which results in the emergence of exotic physicochemical properties, quasi-crystalline order, and emergent correlated effects. For instance, Berashevich et al.38 applied two stacked graphene flakes by an interlayer twist to investigate the interlayer coupling of TBG flakes, finding that repulsion, decoupling and enhanced coupling appear at different exact twist angle. Mirzakhani et al.39 investigated the effect of twisting angle on the energy levels of TBG QDs at the absence of magnetic field, demonstrating that the dependence between the electronic structures and stacking patterns. In addition to interlayer twist, the introduction of an external electric field perpendicular to the BG QDs layers is also an effective tool to manipulate the interaction coupling effect and the charge rearrangement between the constituent layers. Many desirable properties of bilayer graphene and its QDs are induced at an external electric field,7,8,40–43 which can be detected, controlled and measured by experiments. In recent years, significant progresses in preparation and characterization on TBG QDs have become available. Many experiments have synthesized BG by physicochemical methods,44,45 to gain their QDs by cutting techniques,46,47 and to precisely control their interlayer twist angle.48
Polarizability serves as a valuable tool to explore the electron response to an external field, thereby enabling the study of response behavior of bilayer graphene quantum dots with a relative interlayer twist to an external electric field. In order to evaluating the competitive relationship between the intra- and inter-layer contributions upon the external applied field, a polarizability partitioning scheme is adopted based on density functional theory calculations, decomposing the total polarizability into atomic contributions – namely, intra- and inter-layer counterparts of polarizability.49 The TBG QDs twist angle dependences of, energy, band gap, charge flow behavior and polarization, including total, intra- and inter-layer parts, and atomic charge variation induced by an external field, are analyzed systematically in this work. Our investigation on the size- and interlayer twist-mediated polarizability characteristics in TBG QDs demonstrates that controlling the stacking pattern of layered-materials is a serviceable pathway to realize fascinating physicochemical and biological properties and provide a deep insight on design of novel graphene-based devices to further extend their applications.
2. Methodology
In this study, we utilize four coronene-based monolayer GQDs composed of 13, 37, 73, and 121 carbon atoms, each with 9, 15, 21, and 27 terminal hydrogen atoms, to construct twisted bilayer GQDs, as illustrated in Fig. 1. Each monolayer contains a single valence electron and is situated parallel to the xy plane. The influence of interlayer distances on the strength of interlayer coupling has been discussed in previous work.49,50 It is worth noting that an optimized interlayer distance of approximately 3.34 Å (ref. 51 and 52) is adopted to the fixed GQDs samples in experiments. Consequently, we treat the GQDs with an equilibrium interlayer distance of 3.34 Å in the following calculations. Compared to the stationary bottom layer, the top layer of the quantum dot (QD) is rotated by an angle θ around one of the carbon (C) atoms located in the central six-membered ring, as depicted in Fig. 1(a). The constructed interlayer twist configurations are created by fixing the bottom layer and twisting the opposite layer about the carbon atoms of the innermost cycle from 0° to 60° at an interval of 1°, which are respectively denoted as TBG44, TBG104, TBG188 and TBG296 depending on their total number of atoms. The stacking patterns vary with twisting, which are categorized as AA, SP and AB stackings. One corresponds to AA stacking, where the C atoms in the top layer is right above the atoms in bottom layer. In the AB stacking, atoms in top layer position above the center of six-rings in the bottom layer. Meanwhile, the SP stacking pattern is considered as the intermediate between AA and AB stackings. Fig. 1(b) depicts the optimized geometrical TBG systems with different sizes at θ = 0° (AA stacking) and 60° (full-AB stacking). Similar models were built in ref. 49 and 50 in which the monomers are extended phenalenyls, and the rotation axis passes through the center of the innermost six-membered ring.
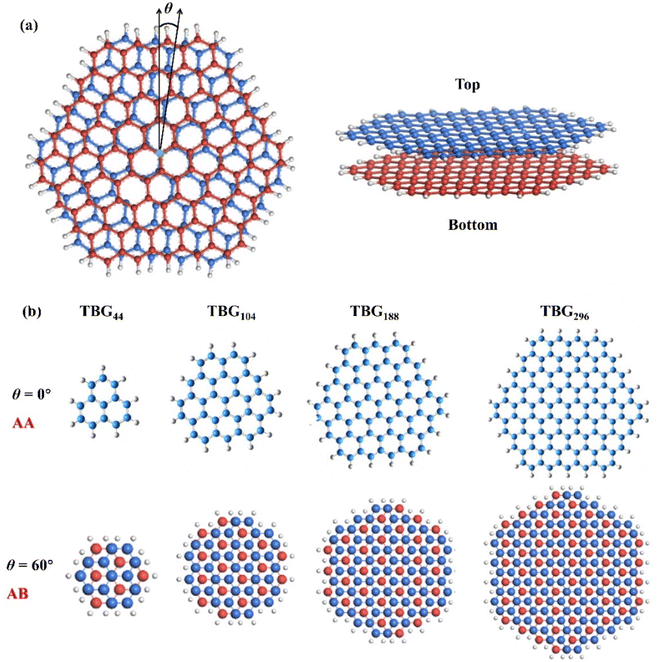 |
| Fig. 1 (a) Top and side view of a twisted bilayer graphene quantum dot (TBG QD) characterized by a specific twisting angle denoted as θ. (b) The top-view diagram of the four constructed TBGN configurations with a twist angle of θ = 0° and 60°, named AA and AB stacking patterns respectively. Blue and red balls represent carbon atoms of different component layers respectively. | |
All the first-principles calculation results presented in this paper are evaluated within the spin-polarized density functional theory (DFT) framework, as implemented in Gaussian 16 package,53 including the geometric optimization, energetic and electronic properties under the effect of the external electrical field. The influence and reliability of dispersion correction for functional and the diffuse functions for basis set on the polarizability of GQDs have been explored in ref. 49 and 54. “To accurately describe the long-range and van der Waals interlayer coupling in the twisted bilayer structures of different sizes, the functional CAM-B3LYP55 including long-range correction and Grimme's D3 correction56 and the standard Gaussian-type basis set 6-31G(d,p)57 are employed to study the TBG QDs with different sizes.
Electron delocalization in a layered configuration has two origins, intra and inter contributions respectively. The intra-layer part derives from electron redistribution on two sides of the layer. The inter-layer part involves the overlap of electron cloud of the two layers, or electron flow between them. To differentiate the intra- and inter-layer contribution counterparts, a polarizability partitioning scheme has been developed to decompose the total polarizability into distributional contributions. The TBG QD is decomposed into two parts, the top and the bottom contributions, along their symmetric xy plane. One has
|
αzz = αPzz,T + αpzz,B + αQzz
| (1) |
where
αP denotes the intra-layer polarizability and
αQ the inter-layer polarizability. B and T denote the bottom and top layers, respectively. Both the intra- and inter-layer polarizability are evaluated with a finite field approach. The field strength is adopted 0.001 a.u., which produces adequate accuracy in numerical differentiation.
|
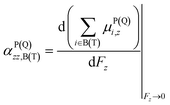 | (2) |
where
|
μPi,z = ∫(rz − r0i,z)wi(r)ρ(r)dr
| (3) |
|
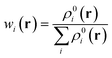 | (4) |
and
|
μQi,z = r0i,zqi = r0i,z∫wi(r)ρ(r)dr
| (5) |
where
ρ(
r) is the electron density at
r,
r0i is the position of atom
i, and
qi is the atomic net charge. Additionally,
ρ0i(
r) is the pre-molecular density. Based on Hirshfeld approach,
58 the electron density can be divided into atomic contribution, in which the density is partitioned according to a weight factor (
wi) calculated from
ρ0i(
r) of the individual atoms.
3. Results and discussion
3.1 Configuration stability and intrinsic electronic property
Before elucidating the polarizability characteristics, it is essential to delve into relative structural stability of TBG QDs with diverse size. The relative energy of TBGN systems is significantly dependent on the interlayer twisting. Taking the structure of θ = 0° as a reference, Fig. 2(a) displays the dependence of the twist angle on the average relative energy (ΔEave) of TBG QDs between untwisted and twisted structures. For each size, it is found that the highest energy of ΔEave is located at the untwisting point (θ = 0°), corresponding to the full-AA stacking pattern, and the energy decreases rapidly as the angle twists away from θ = 0°. It is found that ΔEave of TBG44, TBG104, TBG188 and TBG296 decreases to the first local minimum (θE) around 41°, 22°, 15° and 11°, respectively. θE decreases with increasing of the TBG QD size. After θE, ΔEave remains conspicuous oscillations until θ = 60°, at which the full-AB stacking (Bernal structure) with the lowest energy is achieved. During interlayer twisting, the number of local minima of ΔEave varies with QD size. Specifically, there is 1, 2, 3 and 4 local minima points of the average relative energy for the TBG44, TBG104, TBG188 and TBG296 systems, respectively. For instance, the TBG188 structure has a relative local minimum ΔEave value that exists simultaneously at θ = 15°, 35° and 60°. Meanwhile, the differences between those local energy minima and maxima values are approximately the same for all the given sizes. Moreover, the QD model with the smallest size has the most stable structure at θE rather than θ = 60° due to the strong effect of the terminal hydrogen atoms. While for TBG104, the ΔEave values corresponding to θ = θE and 60° are almost equal. And for the other two configurations with larger sizes, the ΔEave reaches the greatest negative value at θ = 60°, indicating that the terminal effect deceases with QD size. The results are in good agreement with the previous reports that full-AB stacking is the most stable stacking pattern for bilayer graphene.38,59,60 The ΔEave variation is similar to those found in the phenalenyl-based models.50 Using high-level ab initio calculations, Cui et al.61 computed the interaction energy of TBG44 in both the singlet and triplet states, finding that the energy landscape depends closely on the electron configuration and interlayer distance. While the minima occur at 0° and 60° in the singlet state, the energy peaks at 0° in the triplet state and decreases with the twist angle. The potential of energy surface becomes flat at θ = 30, 45 and 60°. Our findings suggest that in addition to the well-known Bernal structures, there are many analogous local energy minima for TBG QDs with different sizes in the process of interlayer twisting. That is, the stabilities of TBG QDs at some immediate angles can be compared to that of the most stable Bernal structures.
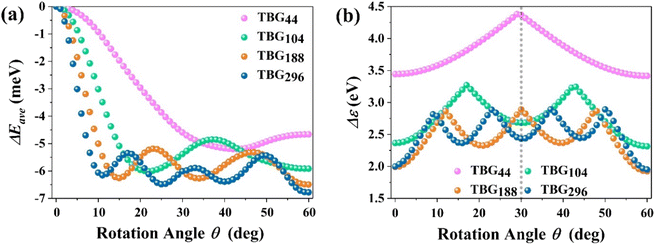 |
| Fig. 2 (a) The variation diagram of the average relative energy (ΔEave) of TBGN (N = 44, 104, 188 and 296) as a function of the rotation angle θ from 0° to 60°. (b) Variation of the HOSO–LOSO gap (Δε) of TBG QDs versus twist angle. | |
Furthermore, the implementation of an interlayer twist in the BG QD structures has been established as a convenient method to manipulate their intrinsic electronic characteristics. Fig. 2(b) illustrates the evolutions in the energy gap (Δε) between the highest occupied spin-orbitals (HOSO) and the lowest unoccupied spin-orbital (LUSO) of the TBGN systems with increasing rotation angle, ranging from 0° to 60°. Our results clearly demonstrate that the band gaps of the all four studied sizes have an obvious twisting dependence, in which the variation trends have a six-fold rotating symmetry with a mirror symmetry of θ = 30°. Therefore, we can clearly obtain the twist and size dependence of band gap when an interlayer rotation in the range from 0° to 30° is exerted, which plays a significant role in practical engineering of the unique functionality of TBG QDs-based optoelectronic devices. The Δε of TBGN increases rapidly upon twist, and then reaches their varieties of local minima and maxima, among which the number of local maxima is 1, 2, 3 and 4 for TBG44, TBG104, TBG188 and TBG296, respectively. At this point, Δε reaches the first local maximum (θg) at approximately 30°, 17°, 12° and 9° for the four models, with the respective value of 4.37, 3.27, 2.86 and 2.81 eV. The size-dependent band gap can be attributed to the weak quantum confinement effect in the QD systems with larger size, leading to a reduction in Δε with increasing QD size. Furthermore, Δε of TBG44 maintains a continuously rising trend with the increasing twisting angle when θ deviates from 0°, and displays a downward trend once θ is exceeds 30°, that the values of Δε are larger than those of other larger sizes at varying rotation angles. For the other three sizes, the local minima values are almost identical for corresponding size except for θ = 0° and 60°, and the similar trend is observed for the local maxima, while the difference of Δε among TBG104, TBG188 and TBG296 decreases with QD size. The above results reveal that the intrinsic electronic properties of TBG QDs depends strongly on the rotational symmetry as well as twist angle, which regularly alter the relative positions of carbon atoms between the two constituent graphene layers.
3.2 Axial polarizability in the direction perpendicular to the QD plates
The twist angle (θ) dependence of the axial polarizability (αzz) for TBG QDs is further investigated to explore the electron response behavior to an external field applied in the direction perpendicular to the QDs plane. Fig. 3(a–d) displays the calculated αzz variations of TBGN vs. twist angle. αzz increases instantly when θ leaves 0°, arrives at the first maximum (θP), then reduces rapidly to a local minimum, and then remains a strong fluctuation with twisting until θ = 60°. Obviously, αzz strongly depends on twist angle and QD size. Upon twist, αzz reaches the first peak with maximum value of 107, 268, 492 and 778 a.u. for TBG44, TBG104, TBG188 and TBG296, respectively, suggesting that the constructed TBG QDs are the mesoscopic systems with marked quantum size effect that the corresponding αzz is much stronger with the QDs size increasing. What's more, θP of those four sizes locates at about 32°, 18°, 12° and 9°, respectively, decreasing with the increase of QD size. Besides the effect on the magnitude of αzz, QD size also has a strong effect on polarizability fluctuations during twist. The number of αzz local minima/maxima varies regularly with the change of the QD size, which corresponds to 1, 2, 3 and 4 for TBG44, TBG104, TBG188 and TBG296, respectively. For TBG188 and TBG296 bilayers, the amplitude of following fluctuations gradually increases with the increasing rotation angles after reaching the largest polarizability at θP.
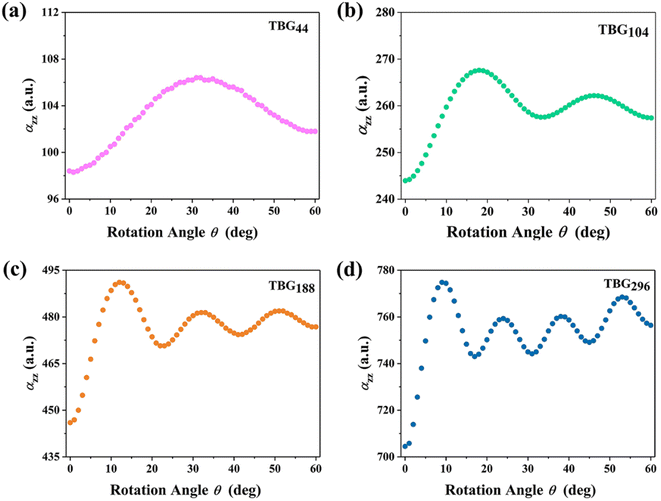 |
| Fig. 3 (a–d) The axial polarizability (αzz) of the constructed TBG QDs varies as a function of twist angle (θ). | |
In general, the transition of HOSO and LUSO states from θ = 0° to θ = θP implies the electron density rearrangement between electron donor to electron acceptor states. The relative displacement of carbon atoms between the two constituent layers induced by the interlayer rotation allows for the diverse interlayer stacking patterns in TBG QDs, in which θ = θP corresponds to the immediate stacking order between the full-AA and full-AB structures. The dependence of HOSO and LUSO displacements on the electric field direction implies that the orbital distribution between the two layers of twisted graphene quantum dots is non-uniform. Fig. 4(a) illustrates the distribution of HOSO and LUSO orbitals (Kohn–Sham orbitals) of TBG104 at 0° and θP rotation angles under the presence of an external electric field, which can be attributed to the interlayer coupling effect in TBG configurations. The HOSO and LUSO of both twisted and untwisted structures are mainly contributed by the C-pz orbitals on the conjugated carbon rings. In the case of AA stacking (θ = 0°), the pz orbitals of one layer interact with those of the other layer. Interlayer coupling is weaker due to the forbidden overlap of the HOSO in stacked TBG materials with opposite phases. Conversely, interlayer coupling is stronger due to the allowed overlap of the LUSO in stacked TBG with identical phases. When the twist angle is θp, the pz orbitals of the upper layer moves towards the six-membered ring of the lower layer, forming a favorable p–π interaction that enhances interlayer coupling. In the twisted structure, significant interlayer orbital overlap is observed for both the HOSO and LOSO states distributions. In the twisted structure, the interlayer coupling resulting from p–π interactions reduces the HOSO and enhances the LUSO, leading to further separation between the HOSO–LUSO. As a result, the twisted structure has a larger Δε value compared to the non-twisted structure, confirmed by Fig. 2(b). Overall, an interlayer rotation is mainly responsible for the energy gap transition through changing the charge density overlapping, originating from a decrease of the energy level of HOSO and an increase of the energy level of LOSO states. Fig. 4(b) also clearly shows the configurations of the given four TBG QDs with a twist angle of θP.
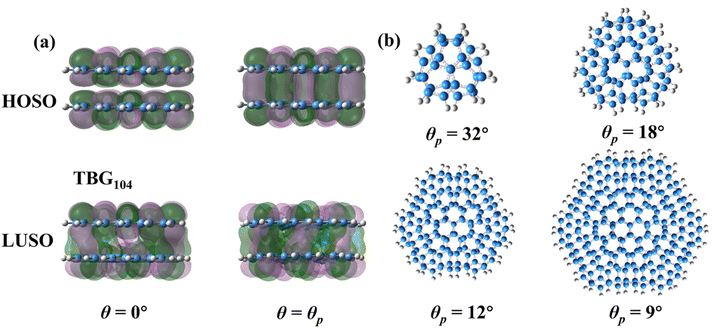 |
| Fig. 4 (a) The distribution of HOSO and LUSO orbitals for TBG104 system at θ = 0° and θP. (b) The equilibrium configurations of TBG QDs at θp. | |
Furthermore, Fig. 5 shows the average axial polarizability
of TBGN. The
value of TBG44 is much smaller than that of those three larger structures. The enhanced
can be observed in the other three larger TBGN, which implies that those three sizes in the z-direction possess promoted ability of electron rearrangement under an external electric field.
at θP increases with increases of QD size, while the difference decreases with size. In addition to the first peak, the rest of local maxima/minima of
for TBG104, TBG188 and TBG296 coincide in some twist angles varying with size, showing small difference among them. The phenalenyl-based models49 exhibit similar variation in
although the polarizability components are to some extent different in magnitude. It is understandable that the unpaired electrons exhibit a different delocalization effect from those in a closed shell, which usually leads to different polarization responses.
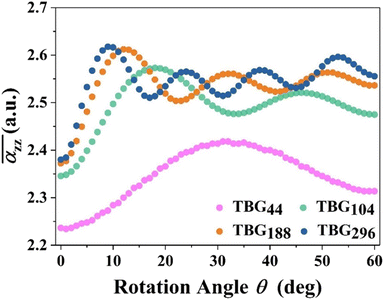 |
| Fig. 5 The average axial polarizability of TBG QDs as functional of twist angle (θ). | |
Interestingly, αz and its
reach the largest value at size-dependent θp, corresponding to the first occurrence of AB stacking in the outmost cycle. In this case, the GQD's diameter (D) and its corresponding location for the appearance of largest αzz (θp) can be used the following geometry relation:49
|
 | (6) |
where
d is the carbon bond length. Using
eqn (6), the twist angle with largest
αz appears for those three larger GQD models can then be predicted as 16.4°, 11.0° and 8.2°, matching well with the calculated values of 18°, 12° and 9°, as shown in
Fig. 3. It is evident that for larger size quantum dots, the given geometric relation can predict more accurately the twist angle corresponding to the appearance of the largest peak of
3.3 Decomposed intra- and interlayer polarizability
Interlayer coupling is crucial for many intriguing novel properties of twisted bilayer graphene, especially under an external electric field. Actually, interlayer polarizability is an important knob to explore the interlayer coupling when an external filed is perpendicularly applied to the stacking plane of TBG. Intra-layer polarization is derived from the charge redistribution on both sides of each layer, while the interlayer polarization comes from electron redistribution between constituent layers. The decomposed intra- and interlayer components, αPzz and αQzz, of TBGN are shown in Fig. 6. αPzz has a large value but slight variations during twisting, reducing by 0.61%, 1.63%, 2.36% and 2.58% for TBG44, TBG104, TBG188 and TBG296, respectively, indicting the intralayer part dominates in αzz but keeps almost unchanged during twist. It is surprising that αQzz increases by 46.52, 58.25, 63.42 and 65.86%, respectively, and remains almost similar trend as well as possesses the same local maxima and minima with those of αzz. Taking the largest size as an example, αQzz increases rapidly at small θ, comes to its first peak at 9°, drops and reaches the first minimum at about 15°, thereafter, arrives at other local maxima (22°, 37° and 52°) and minima (30°, 45° and 60°). Similar variations also occur for the phenalenyl-based models.49 It is evident that both of the variation gradients of αPzz and αQzz relative to untwisted structures increase with size, showing high dependency on QD size. The result manifests that the variation of axial polarizability in the z direction is dominated by interlayer counterpart during rotating. The enhanced interlayer polarization highly depends on the stacking patterns.
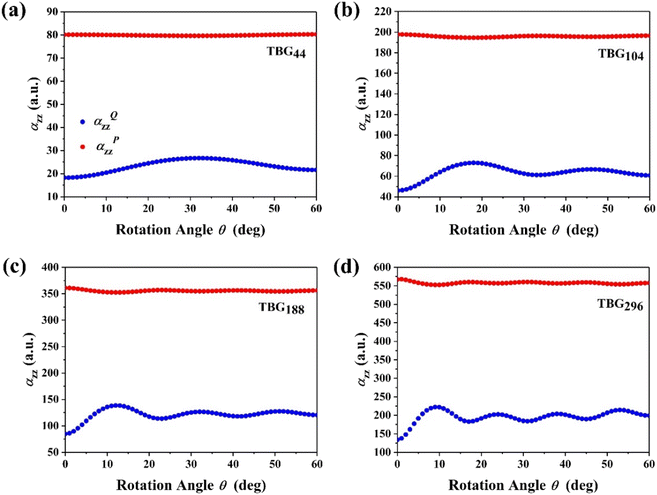 |
| Fig. 6 (a–d) The intralayer polarizability (αPzz, red) and interlayer part (αQzz, blue) of TBG as functional of twist angle. | |
Upon twisting, the relative displacements of carbon atoms in the opposite layer result in various stacking patterns with diverse interlayer couplings. For full-AA stacking, carbon atoms sit right above the carbon atoms in the opposite layer, resulting in the strongest interlayer repulsion and the smallest αQzz. Once θ stays away from 0°, the carbon atoms in the outmost cycle gradually move to the center of the six-membered ring, making the weakening of repulsive interaction and increasing of interlayer attraction. αQzz reaches the largest value at θp when AB stacking firstly appears at the outmost cycle to being a mixture of AA, SP and AB stackings. After θp, the coupling becomes more complexed because the next outmost and other cycles begin to form AB stacking. Especially, αQzz at θ = 60° is a local minimum rather than a maximum, indicating that αQzz is not just determined by van der Waals interaction, but other electronic coupling. Therefore, the mixing proportion of AA, SP and AB stackings is crucial for the interlayer coupling of TBG QDs owing to the absence of periodic moiré potential.
3.4 Average atomic charge (dq/dF) from inner to outmost cycle
According to eqn (2)–(5), one can decompose the interlayer polarization into atomic contributions. Because μPi,z is coordinate-related, it can be defined as follow: |
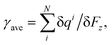 | (8) |
where γ represents the variation of the net charge of atom i driven by an external electric field. δqi and δFz denote the variation of the net charge of ith atom and the external field, respectively. N is the total number of the atoms in each cycle. γave is the average γ in each cycle. Actually, γ is a crucial term in αzz, which is thus applied to evaluate the atomic contribution to αQzz. Each layer of the four constructed TBGN models can be decomposed into 2m + 1 (m = 1, 2, 3, 4) cycles, respectively. Fig. 7 presents the γave of each cycle for the structures of θ = 0–60°. For those four sizes, each cycle of γave variation increases upon twist, in which innermost and second innermost cycles show a little different trend for corresponding larger three sizes of αzz and αQzz, while other cycles have the similar trends. At a specific QD size, the outmost cycle possesses the largest γave and the next outmost cycle is the second largest, while that of the other cycles present small difference among them during twist. For example, largest γave in the innermost cycle is found at θ = 32°, 45°, 48° and 51° for TBG44, TBG104, TBG188 and TBG296, with the value of 0.10, 0.13, 0.15, 0.16 a.u., respectively, while largest γave in the outermost cycle appears to θ = θp, whose values are 0.25, 0.33, 0.37 and 0.40 a.u., respectively.
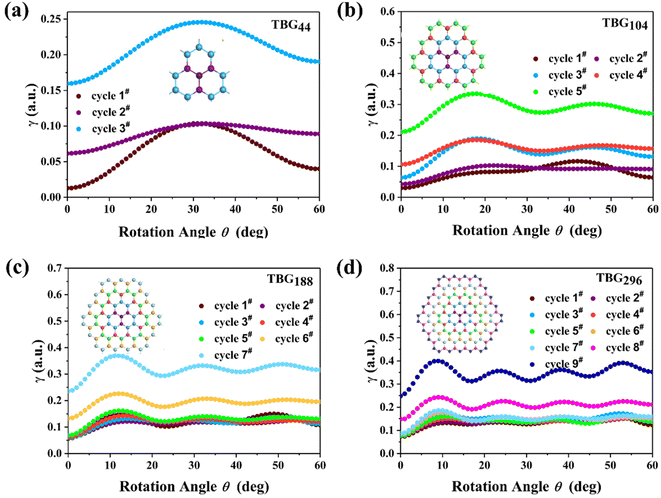 |
| Fig. 7 (a–d) Averaged atomic γ of each cycle of TBGN as functional of twist angle. The TBG44, TBG104, TBG188 and TBG296 configurations are grouped into 3, 5, 7 and 9 cycles respectively, distinguished by color. | |
In order to deeply investigate the atomic contribution to αQzz, TBG296 is selected to proceed further analyze. Fig. 8 depicts the twist angle dependence on the carbon atomic γ of this system. γ for all the atoms presents the similar trend with αQzz until around θ = θp. Thereafter, γ for some atoms have much different trends with that of αQzz. It is noted that the γ variations for most atoms are multifarious at larger twist angle (θ > 35°). For example, at θ = 60°, some atoms in the outmost cycle have smallest γ, while some atoms have largest γ, even higher than that of at θ = θp. The results demonstrate that αQzz highly relies on the relative atomic position. Furthermore, Fig. 9 displays the γ for carbon atoms of TBGN at θ = 0° and θp. Atoms in different cycles have different γ, which has similar values in the inner cycle in addition to the outmost and its next cycles. And in the same cycle γ shows small difference. γ at θ = 0° is much smaller than that of θ = θp, and γ inclined to promote with size. The γ variations in Fig. 7–9 demonstrate that the magnitude of γ is strongly depend on the location of atom relative to the center and its stacking pattern with respect to six-membered ring in opposite layer. Atoms closer to the AA stacking tend to have smaller γ, while those closer to AB stacking and/or atoms in the outmost cycles tend to have larger γ.
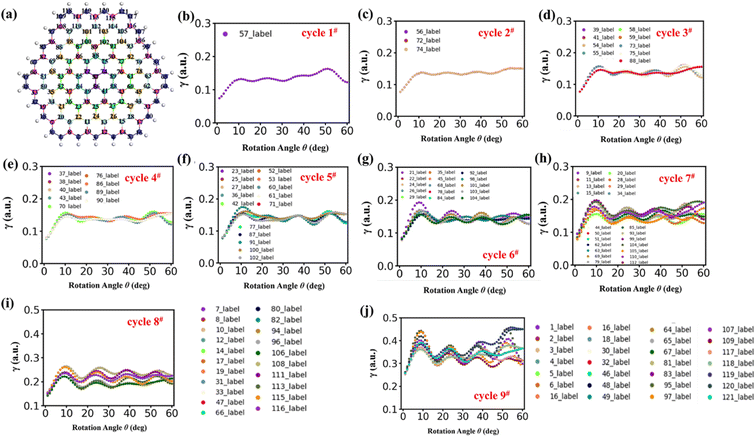 |
| Fig. 8 (a) Presents the top layer of TBG296 with the labelled atom number. (b–j) The atomic γ of TBG296 in the top layer of cycle 1#–cycle 9# with twisting. | |
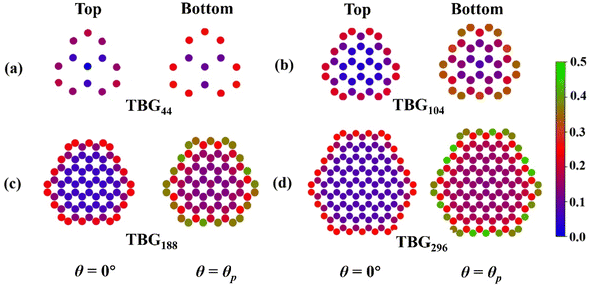 |
| Fig. 9 The atomic γ (in a.u.) of in the top and bottom layer of (a) TBG44, (b) TBG104, (c) TBG188 and (d) TBG296 at θ = 0° (left) and θp (right). | |
4. Conclusion
In summary, we have carried out an in-depth theoretical investigation regarding the characteristic of interlayer polarizability and charge transfer in twisted bilayer graphene quantum dots driven by an external electric field. Our finding indicate that a relatively stable stacking pattern exists, which possesses the largest polarizability and its interlayer part, in contrast to the well-known Bernal stackings. The variation trends of band gap for the four studied sizes exhibit a six-fold rotating symmetry with a mirror symmetry of θ = 30°. Furthermore, a quantity, αQzz, is defined from electron density decomposition and used to measure the interlayer polarizability effectively, dominating the variation of total polarizability with twisting angle. We have also verified the field-driven charge flow from one layer to another layer through the variations in αQzz. αzz and αQzz, and charge transfer driven by an external field find their minimum at θ = 0° and largest values at size-dependent θp, which is corresponding to the AB stacking firstly appears at outmost cycle. By utilizing a derived equation with the relation between the GQD's diameter and twist angle, one can obtain the corresponding location for the appearance of largest αzz, αQzz and charge transfer. This allows one to predict the size of a TBG sample from the “magic angle” at which phenomena related to the largest polarizability appear. Moreover, our investigation also revealed that the largest atomic γ value is achieved at the outmost cycle for a specific angle and QD size. Our investigation on the size- and interlayer twist-mediated electric structure, polarizability and charge transfer characteristics in TBG QDs demonstrates that controlling the stacking pattern of layered-materials is a serviceable pathway to realize fascinating physicochemical and biological properties. Finally, similar twist-driven variations are noted for the coronene- and phenalenyl-based models. This study provides a deep theoretical insight on design and optimization of novel graphene-based devices to further extend their applications.
Author contributions
Xiangyue Liu: conceptualization, methodology, software, validation, writing – original draft, investigation, writing – review & editing, visualization. Xian Wang: investigation, writing – original draft. Shengping Yu: methodology, resources, project administration. Guangzhao Wang: investigation, methodology, supervision. Bing Li: resources, data curation, writing – review & editing, supervision. Tiantian Cui: investigation, methodology. Zhaoyang Lou: resources, data curation, writing – review & editing, supervision. Hong Ge: supervision, resources, project administration, funding acquisition, writing – review & editing.
Conflicts of interest
The authors declare no competing interests.
Acknowledgements
We acknowledge the support from the Scientific and Technological Project in Henan Province (grant no. 222102310015), and The Medical Science and Technology Project in Henan Province (grant no. SBGJ202102056).
References
- H. Min and A. H. MacDonald, Prog. Theor. Phys. Suppl., 2008, 176, 227–252 CrossRef CAS.
- L. Banszerus, B. Frohn, A. Epping, D. Neumaier, K. Watanabe, T. Taniguchi and C. Stampfer, Nano Lett., 2018, 18, 4785–4790 CrossRef CAS PubMed.
- M. Eich, R. Pisoni, A. Pally, H. Overweg, A. Kurzmann, Y. Lee, P. Rickhaus, K. Watanabe, T. Taniguchi, K. Ensslin and T. Ihn, Nano Lett., 2018, 18, 5042–5048 CrossRef CAS PubMed.
- G. W. Burg, E. Khalaf, Y. Wang, K. Watanabe, T. Taniguchi and E. Tutuc, Nat. Mater., 2022, 21, 884–889 CrossRef CAS PubMed.
- J. M. Park, Y. Cao, L.-Q. Xia, S. Sun, K. Watanabe, T. Taniguchi and P. Jarillo-Herrero, Nat. Mater., 2022, 21, 877–883 CrossRef CAS PubMed.
- Y. Zhang, R. Polski, C. Lewandowski, A. Thomson, Y. Peng, Y. Choi, H. Kim, K. Watanabe, T. Taniguchi, J. Alicea, F. von Oppen, G. Refael and S. Nadj-Perge, Science, 2022, 377, 1538–1543 CrossRef CAS PubMed.
- Y. Cao, D. Rodan-Legrain, O. Rubies-Bigorda, J. M. Park, K. Watanabe, T. Taniguchi and P. Jarillo-Herrero, Nature, 2020, 583, 215–220 CrossRef CAS PubMed.
- Y. Cao, J. Luo, V. Fatemi, S. Fang, J. Sanchez-Yamagishi, K. Watanabe, T. Taniguchi, E. Kaxiras and P. Jarillo-Herrero, Phys. Rev. Lett., 2016, 117, 116804 CrossRef CAS PubMed.
- K. Kim, A. DaSilva, S. Huang, B. Fallahazad, S. Larentis, T. Taniguchi, K. Watanabe, B. J. LeRoy, A. H. MacDonald and E. Tutuc, Proc. Natl. Acad. Sci. U. S. A., 2017, 114, 3364–3369 CrossRef CAS PubMed.
- A. Kerelsky, L. McGilly, D. Kennes, L. Xian, M. Yankowitz, S. Chen, K. Watanabe, T. Taniguchi, J. Hone, C. Dean, A. Rubio and A. Pasupathy, Nature, 2019, 572, 95 CrossRef CAS PubMed.
- M. V. Hosseini and M. Zareyan, Phys. Rev. B: Condens. Matter Mater. Phys., 2012, 86, 214503 CrossRef.
- H. C. Po, L. Zou, A. Vishwanath and T. Senthil, Phys. Rev. X, 2018, 8, 031089 CAS.
- R. Bistritzer and A. H. MacDonald, Proc. Natl. Acad. Sci. U. S. A., 2011, 108, 12233–12237 CrossRef CAS PubMed.
- T. Ohta, A. Bostwick, T. Seyller, K. Horn and E. Rotenberg, Science, 2006, 313, 951–954 CrossRef CAS PubMed.
- Y. Zhang, T.-T. Tang, C. Girit, Z. Hao, M. C. Martin, A. Zettl, M. F. Crommie, Y. R. Shen and F. Wang, Nature, 2009, 459, 820–823 CrossRef CAS PubMed.
- S. Latil and L. Henrard, Phys. Rev. Lett., 2006, 97, 036803 CrossRef PubMed.
- A. Gevaerd, C. E. Banks, M. F. Bergamini and L. H. Marcolino-Junior, Electroanalysis, 2019, 31, 838–843 CrossRef CAS.
- D. Pan, J. Zhang, Z. Li and M. Wu, Adv. Mater., 2010, 22, 734–738 CrossRef CAS PubMed.
- K. A. Ritter and J. W. Lyding, Nat. Mater., 2009, 8, 235–242 CrossRef CAS PubMed.
- B. D. Mansuriya and Z. Altintas, Sensors, 2020, 20, 1072 CrossRef CAS PubMed.
- P. Tian, L. Tang, K. S. Teng and S. P. Lau, Mater. Today Chem., 2018, 10, 221–258 CrossRef CAS.
- S. Chung, R. A. Revia and M. Zhang, Adv. Mater., 2021, 33, 1904362 CrossRef CAS PubMed.
- M. R. Younis, G. He, J. Lin and P. Huang, Front. Chem., 2020, 8, 424 CrossRef CAS PubMed.
- M. Nurunnabi, Z. Khatun, K. M. Huh, S. Y. Park, D. Y. Lee, K. J. Cho and Y.-K. Lee, ACS Nano, 2013, 7, 6858–6867 CrossRef CAS PubMed.
- N. Abdullah Al, J. E. Lee, I. In, H. Lee, K. D. Lee, J. H. Jeong and S. Y. Park, Mol. Pharmaceutics, 2013, 10, 3736–3744 CrossRef PubMed.
- A. Xu, P. He, T. Huang, J. Li, X. Hu, P. Xiang, D. Chen, S. Yang, G. Wang and G. Ding, Synth. Met., 2018, 244, 106–112 CrossRef CAS.
- L. Lin, X. Song, Y. Chen, M. Rong, T. Zhao, Y. Wang, Y. Jiang and X. Chen, Anal. Chim. Acta, 2015, 869, 89–95 CrossRef CAS PubMed.
- S. M. Ghafary, M. Nikkhah, S. Hatamie and S. Hosseinkhani, Sci. Rep., 2017, 7, 9552 CrossRef PubMed.
- J. Ge, M. Lan, B. Zhou, W. Liu, L. Guo, H. Wang, Q. Jia, G. Niu, X. Huang, H. Zhou, X. Meng, P. Wang, C. S. Lee, W. Zhang and X. Han, Nat. Commun., 2014, 5, 4596 CrossRef CAS PubMed.
- F. Khodadadei, S. Safarian and N. Ghanbari, Mater. Sci. Eng., C, 2017, 79, 280–285 CrossRef CAS PubMed.
- X. Wang, X. Sun, J. Lao, H. He, T. Cheng, M. Wang, S. Wang and F. Huang, Colloids Surf., B, 2014, 122, 638–644 CrossRef CAS PubMed.
- Y. Yang, L. Zhang, J. Cai, X. Li, D. Cheng, H. Su, J. Zhang, S. Liu, H. Shi, Y. Zhang and C. Zhang, ACS Appl. Mater. Interfaces, 2016, 8, 1718–1732 CrossRef CAS PubMed.
- J. Ruan, Y. Wang, F. Li, R. Jia, G. Zhou, C. Shao, L. Zhu, M. Cui, D.-P. Yang and S. Ge, ACS Appl. Mater. Interfaces, 2018, 10, 14342–14355 CrossRef CAS PubMed.
- F.-I. Tung, L.-J. Zheng, K.-T. Hou, C.-S. Chiang, M.-H. Chen and T.-Y. Liu, Nanoscale, 2020, 12, 8809–8818 RSC.
- B. Trauzettel, D. V. Bulaev, D. Loss and G. Burkard, Nat. Phys., 2007, 3, 192–196 Search PubMed.
- S. Zhu, J. Zhang, C. Qiao, S. Tang, Y. Li, W. Yuan, B. Li, L. Tian, F. Liu, R. Hu, H. Gao, H. Wei, H. Zhang, H. Sun and B. Yang, Chem. Commun., 2011, 47, 6858–6860 RSC.
- A. Kurzmann, M. Eich, H. Overweg, M. Mangold, F. Herman, P. Rickhaus, R. Pisoni, Y. Lee, R. Garreis, C. Tong, K. Watanabe, T. Taniguchi, K. Ensslin and T. Ihn, Phys. Rev. Lett., 2019, 123, 026803 CrossRef CAS PubMed.
- J. Berashevich and T. Chakraborty, Phys. Rev. B: Condens. Matter Mater. Phys., 2011, 84, 033403 CrossRef.
- M. Mirzakhani, F. M. Peeters and M. Zarenia, Phys. Rev. B, 2020, 101, 075413 CrossRef CAS.
- J. M. B. Lopes dos Santos, N. M. R. Peres and A. H. Castro Neto, Phys. Rev. Lett., 2007, 99, 256802 CrossRef CAS PubMed.
- J. B. Oostinga, H. B. Heersche, X. Liu, A. F. Morpurgo and L. M. K. Vandersypen, Nat. Mater., 2008, 7, 151–157 CrossRef CAS PubMed.
- E. V. Castro, K. S. Novoselov, S. V. Morozov, N. M. R. Peres, J. M. B. L. dos Santos, J. Nilsson, F. Guinea, A. K. Geim and A. H. C. Neto, Phys. Rev. Lett., 2007, 99, 216802 CrossRef PubMed.
- M. Yankowitz, S. Chen, H. Polshyn, Y. Zhang, K. Watanabe, T. Taniguchi, D. Graf, A. F. Young and C. R. Dean, Science, 2019, 363, 1059–1064 CrossRef CAS PubMed.
- B. Liu, Y. Sheng, S. Huang, Z. Guo, K. Ba, H. Yan, W. Bao and Z. Sun, Chem. Mater., 2019, 31, 6105–6109 CrossRef CAS.
- M. Huang and R. S. Ruoff, Acc. Chem. Res., 2020, 53, 800–811 CrossRef CAS PubMed.
- D. Pan, L. Guo, J. Zhang, C. Xi, Q. Xue, H. Huang, J. Li, Z. Zhang, W. Yu, Z. Chen, Z. Li and M. Wu, J. Mater. Chem., 2012, 22, 3314–3318 RSC.
- S. Jindal and S. M. Giripunje, Synth. Met., 2018, 239, 36–42 CrossRef CAS.
- Y. Yang, J. Li, J. Yin, S. Xu, C. Mullan, T. Taniguchi, K. Watanabe, A. K. Geim, K. S. Novoselov and A. Mishchenko, Sci. Adv., 2020, 6, eabd3655 CrossRef CAS PubMed.
- X. Wang, L. Zhang, S. Yu, M. Yang and K. A. Jackson, Phys. Rev. B, 2021, 104, 155411 CrossRef CAS.
- X. Wang and M. Yang, Appl. Surf. Sci., 2022, 600, 154148 CrossRef CAS.
- Y. Jiang, X. Lai, K. Watanabe, T. Taniguchi, K. Haule, J. Mao and E. Y. Andrei, Nature, 2019, 573, 91–95 CrossRef CAS PubMed.
- B. Sahu, H. Min, A. H. MacDonald and S. K. Banerjee, Phys. Rev. B: Condens. Matter Mater. Phys., 2008, 78, 045404 CrossRef.
- Q. Gao, Z. Zhang, X. Xu, J. Song, X. Li and Y. Wu, Nat. Commun., 2018, 9, 4778 CrossRef PubMed.
- X. Wang, Y. Cui, L. Zhang and M. Yang, Nano Res., 2021, 14, 3935–3941 CrossRef CAS.
- T. Yanai, D. P. Tew and N. C. Handy, Chem. Phys. Lett., 2004, 393, 51–57 CrossRef CAS.
- S. Grimme, J. Antony, S. Ehrlich and H. Krieg, J. Chem. Phys., 2010, 132, 154104 CrossRef PubMed.
- M. J. Frisch, J. A. Pople and J. S. Binkley, J. Chem. Phys., 1984, 80, 3265–3269 CrossRef CAS.
- F. L. Hirshfeld, Theor. Chim. Acta, 1977, 44, 129–138 CrossRef CAS.
- A. Sagar, E. J. Lee, K. Balasubramanian, M. Burghard and K. Kern, Nano Lett., 2009, 9, 3124–3128 CrossRef CAS PubMed.
- V. Perebeinos, J. Tersoff and P. Avouris, Phys. Rev. Lett., 2012, 109, 236604 CrossRef CAS PubMed.
- Z.-H. Cui, H. Lischka, H. Z. Beneberu and M. Kertesz, J. Am. Chem. Soc., 2014, 136, 5539–5542 CrossRef CAS PubMed.
|
This journal is © The Royal Society of Chemistry 2023 |
Click here to see how this site uses Cookies. View our privacy policy here.