DOI:
10.1039/D3RA02988C
(Paper)
RSC Adv., 2023,
13, 15805-15809
Stille vs. Suzuki – cross-coupling for the functionalization of diazocines†
Received
5th May 2023
, Accepted 17th May 2023
First published on 30th May 2023
Abstract
Diazocines are azobenzene derived macrocyclic photoswitches with well resolved photostationary states for the (E)- and (Z)-isomers, which improves their addressability by light. In this work, effective procedures for the stannylation and borylation of diazocines in different positions are reported. Their use in Stille cross-coupling and Suzuki cross-coupling reactions with organic bromides is demonstrated in yields of 47–94% (Stille cross-coupling) and 0–95% (Suzuki cross-coupling), respectively.
Introduction
In the last few years, ethylene-bridged azobenzenes, diazocines, have garnered interest because of their photochemical properties.1 The bent (Z)-isomer is the thermodynamically favoured state, which can be switched to the stretched (E)-configuration using violet light (400 nm). The re-isomerization takes place upon irradiation with green light (520 nm) or thermally. The n–π* absorption maxima of both isomers are well separated, which improves their specific addressability by light.1a Thus, diazocines are promising candidates for a wide range of applications, e.g. molecular imaging,2 optical data storage2b,3 and photodynamic therapy.4 They also have been incorporated into functional polymers.2a,c,3,5 However, the synthesis of functionalized diazocines is more demanding than of similarly substituted azobenzenes.6 Since the discovery of photoswitchable properties of diazocine in 2009,1a the main focus has been on the development of efficient synthetic routes to obtain diazocines with various functional groups.6 Several strategies with a broad substrate scope have been published, e.g. reduction of dinitro compounds,7 oxidation of dianiline derivatives4a,8 or a cross-coupling strategy.9 With those in hand, the number of accessible diazocines was greatly enhanced: precursors with more complex substitution patterns were used and unsymmetrical diazocines could be obtained.4b,10
However, one common tool in organic chemistry for late-stage functionalization, namely cross-coupling reactions, has only been used sporadically, with diazocine as the formally electrophilic component.4a,8a,11 and one single example with a stannylated diazocine.12 In those examples, the cross-coupling reactions were not further investigated (Scheme 1a). We were interested in a more general cross-coupling protocol that tolerates a broad variety of functional groups, and which proceeds via easily accessible and storable diazocine precursors. They necessitated a high yielding synthesis of stannylated and borylated diazocines, which serve as new synthetic reagents in efficient protocols for Stille and Suzuki cross-coupling reactions. As with azobenzenes,13 it is much rarer to be able to prepare the photoswitch as the formally nucleophilic reagent, because of the risk of reduction of the N
N double bond,14 e.g. reductions of the azo group with bis(pinacolato)diboron have been reported.15. However, if organic bromides can be used as coupling partners, the range of accessible products is much broadened (Scheme 1b).
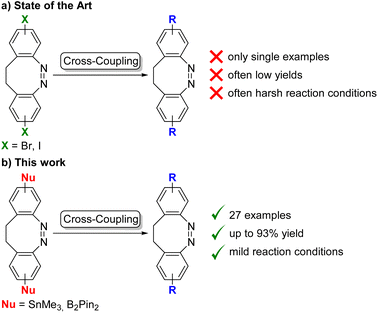 |
| Scheme 1 Cross-coupling reaction of diazocines. | |
Results and discussion
The iodinated diazocines 1 and 2 were synthesized according to optimized literature procedures in yields of 71% and 37%, respectively (see ESI†).1a,11g,12 These were to serve as starting materials for the stannylated (3–4) or borylated diazocines (5–6) as key reagents (Scheme 2). We elected those two types of cross-coupling reaction because of the high bench stability of the corresponding metallated derivatives. Moreover, there was a high potential for the optimization of the Stille reaction as the reported examples led to very low yields of the cross-coupling (10–31%).11b,c,12 To our knowledge, no Suzuki cross-coupling of diazocines has previously been reported.
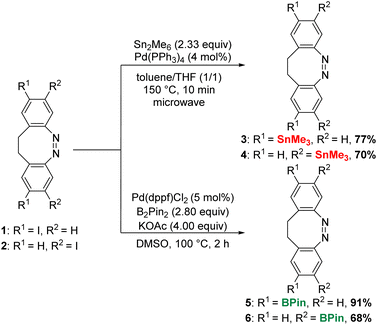 |
| Scheme 2 Synthesis of stannylated 3–4 and borylated diazocines 5–6. | |
The stannylated diazocines could be prepared by a microwave assisted Stille–Kelly cross-coupling:13a In this fashion, the coupling of 1 with hexamethyldistannane provided 3 in 77% and the meta-derivative 4 was obtained in a similar yield of 70%. In comparison to the previously published meta-mono-stannylated diazocine (51%),12 the efficiency of the cross-coupling procedure was significantly higher.
However, the borylation was less straightforward: to synthesize the borylated precursors 5 and 6 Miyaura borylation was used as the starting point.16 Unfortunately, this led to the formation of a reduced species in significant amounts (see ESI†). We attempted to suppress the reduction by applying a range of other catalysts, solvents or bases (see ESI†). However, it emerged that to obtain 5 in high yields up to 91%, the reaction time was the most important parameter (Scheme 2). Therefore, monitoring the reaction progress was crucial. Finally, the Miyaura borylation of 2 furnished 6 in a yield of 68%.
With the diazocine derivatives 3–6 in hand, we investigated their ability to serve as the formally nucleophilic coupling partner in Stille and Suzuki cross-coupling reactions (Scheme 3). The Stille reaction required significant optimization efforts regarding the catalytic systems, additives and reaction temperature (see ESI†). Eventually, a modified procedure by Buchwald17 using a combination of Pd(OAc)2 and XPhos as catalytic system and CsF as additive proved to be superior in the test reaction of 3 with 4-bromotoluene (92% yield after 4 h).
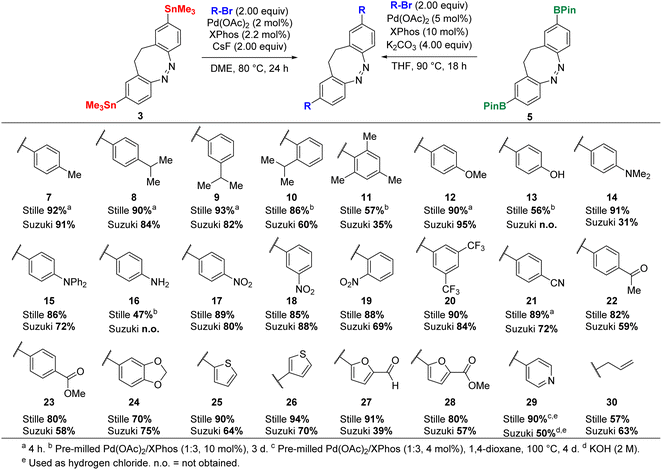 |
| Scheme 3 Scope of Stille and Suzuki cross-coupling reactions for para-substituted diazocines 3 and 5. | |
For the Suzuki cross-coupling, several catalysts and ligands were investigated (see ESI†). We found that the catalytic system involving Pd(OAc)2 and XPhos also yielded the best result for the Suzuki cross-coupling in the coupling reaction between 5 and 4-bromotoluene (91%).
Subsequently, this catalytic system was tested with an electron rich and an electron deficient aromatic electrophile: the coupling of the electron rich 4-bromoanisole performed in a yield of 90% for the Stille coupling and 95% for the Suzuki coupling. The reaction with the electron deficient 1-bromo-4-nitrobenzene yielded 17 in 89% (Stille) and 80% (Suzuki).
Attaching para-, meta- and ortho-isopropyl benzene to obtain 8, 9 and 10 was possible in high yields except for the Suzuki coupling of 1-bromo-2-isopropylbenzene. In this case, the yield was significantly lower with 60% compared to the Stille coupling reaction with 86%. Steric hindrance seemed to influence the Suzuki cross-coupling more strongly than the Stille cross-coupling. This observation was supported by the synthesis of mesityl-diazocine 11 and o-nitrophenyl-diazocine 19. However, to gain the sterically demanding di-functionalized diazocines 10 and 11 in a sufficient yield via the Stille reaction, the amount of catalyst needed to be increased: while the standard reaction conditions mainly led to the mono-coupled diazocines, using 10 mol% of a 1
:
3 mixture of Pd(OAc)2/XPhos furnished 10 in 86% and 11 in 57% yield. Increasing the catalyst load (up to 20 mol%) for the Suzuki cross-coupling, did not improve the yield of 11.
Further cross-coupling reactions of electron deficient compounds provided 18, 20 and 21 in high yields for both cross-coupling types tolerating nitro, nitrile and trifluoromethyl groups. The coupling of stronger coordinating compounds with free electron pairs, e.g. aryl aldehyde (22), aryl ester (23) and furan heterocycles (27 and 28), proceeded in low to moderate yields (39–59%) for the Suzuki cross-coupling, while the Stille cross-coupling gave high yields (80–91%). The same trend became apparent upon the coupling of other heterocycles such as thiophenes (25 and 26) or pyridine (29): the Stille coupling yielded excellent results (90–94%), whereas moderate yields were obtained with the Suzuki coupling (57–66%). However, the functionalization with 4-bromopyridine proved to be challenging for both cross-coupling types.
For the Stille reaction of 3 with 4-bromopyridine it was necessary to increase the temperature to 100 °C and thus the solvent had to be changed to the higher boiling DMSO. For the Suzuki coupling no product could be isolated applying the standard conditions. The desired compound 29 was only obtained with potassium hydroxide a stronger base in aqueous mixture with THF instead of potassium carbonate.
Other electron rich aryl bromides were coupled with 3 and 5 yielding tertiary amines 14 and 15 as well as benzo-dioxole 24 in good to excellent yields. Only compound 14 was isolated in a lower yield of 31% for the Suzuki coupling. Moreover, it was possible to react the unprotected 4-bromophenol and aniline via the Stille reaction using 10 mol% of a 1
:
3 mixture of Pd(OAc)2/XPhos. The corresponding diazocines 13 and 16 were obtained in 56% and 47% yield, respectively.
For the corresponding Suzuki cross-couplings a multitude of different species was observed (Scheme 4). In both cases, the unsubstituted diazocine 31 was obtained as the main product. Besides the monosubstituted side products 32 and 33, we could also identify secondary amine 34 as a coupling product with 4-bromoaniline, which suggests a competing Buchwald–Hartwig cross-coupling reaction. In order to solve the problem, we increased the catalyst load to 20 mol% and changed the base (2 M KOH) but neither changing one condition nor changing both at the same time led to the desired products 13 and 16.
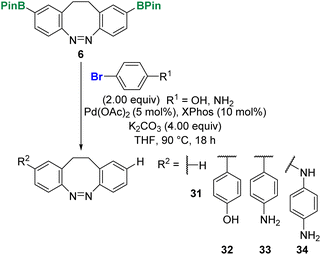 |
| Scheme 4 Side reactions of Suzuki cross-coupling. | |
Lastly, it was also possible to react diazocines 3 and 5 with a non-aromatic bromide. Allyl bromide was coupled to yield 30 in 57% (Stille) and 63% (Suzuki), which could be of use as crosslinker for polymerization reactions (Scheme 3).
It was also investigated if the substitution position of the diazocine influences the cross-coupling reactions. In line with the previous test reactions for para-substituted diazocines 3 and 5, 4-bromotoluene, 4-bromoanisole and 1-bromo-4-nitrobenzene were therefore coupled as model substrates with 4 and 6 (Scheme 5). In all cases, good yields were obtained for the Stille (71–84%) and Suzuki coupling (77–83%). In comparison with the corresponding reactions with para-substituted diazocines 3 and 5, the yields were generally lower; e.g. the coupling of 4-bromoanisole with para-substituted diazocines 3 and 5 proceeded in 90% (Stille) and 95% (Suzuki) but with meta-substituted diazocines 4 and 6 in 71% (Stille) and 78% (Suzuki).
 |
| Scheme 5 Scope of Stille and Suzuki cross-coupling reactions for meta-substituted diazocines 4 and 6. | |
Experimental
General procedure for the Stille–Kelly cross-coupling reaction
Under inert conditions, the corresponding di-iodinated diazocine (345 mg, 750 μmol, 1.00 equiv.), hexamethyldistannane (573 mg, 1.75 mmol, 2.33 equiv.) and [Pd(PPh3)4] (34.8 mg, 30.0 μmol, 4 mol%) were dissolved in toluene (2.5 mL) and THF (2.5 mL) in a microwave reaction vessel. The reaction mixture was stirred for 10 min at 150 °C under microwave irradiation and subsequently filtered through Celite®. Then, the solvent and excess hexamethyldistannane were removed under reduced pressure (9.5 × 10–2 mbar, 80 °C). The residue was purified via column chromatography on silica.
General procedure for the Miyaura borylation
Under inert conditions, the corresponding di-iodinated diazocine (1.00 equiv.), Pd(dppf)Cl2 (5 mol%), B2Pin2 (2.80 equiv.) and KOAc (4.00 equiv.) were dissolved in DMSO (10 mL mmol−1). The mixture was stirred at 100 °C and the reaction progress was monitored by TLC (cyclohexane/DCM/ethyl acetate 55/40/5). After completion (approx. 2 h), the reaction mixture was diluted with ethyl acetate (20 mL mmol−1) and washed with brine (4 × 50 mL mmol−1). The organic phase was dried over sodium sulfate, filtered and the solvent removed in vacuo. The residue was purified by column chromatography on silica (eluent: cyclohexane/DCM/ethyl acetate 55/40/5) to obtain the corresponding diazocine as a yellow solid.
General procedure for Stille cross-coupling reaction
Under inert conditions, the corresponding di-stannylated diazocine (53.4 mg, 100 μmol, 1.00 equiv.), the corresponding brominated coupling partner (200 μmol, 2.00 equiv.), Pd(OAc)2 (449 μg, 2.00 μmol, 2 mol%), XPhos (1.05 mg, 2.20 μmol, 2.2 mol%) and CsF (30.4 mg, 200 μmol, 2.00 equiv.) were dissolved in dry DME (2 mL) in a pressure reaction vial. The mixture was stirred at 80 °C for 24 h. After cooling to 23 °C, the reaction mixture was filtered through Celite® and the solvent was removed under reduced pressure.
General procedure for Suzuki cross-coupling
Under inert conditions, the corresponding di-borylated diazocine 5 (46.0 mg, 100 μmol, 1.00 equiv.), Pd(OAc)2 (1.12 mg, 5 μmol, 5 mol%), XPhos (4.77 mg, 10.0 μmol, 10 mol%), K2CO3 (69.11 mg, 500 μmol, 5.00 equiv.) and the corresponding brominated coupling partner (200 μmol, 2.00 equiv.) were dissolved in THF (5 mL) and sealed in a pressure reaction vial. The mixture was stirred at 90 °C for 18 h. After cooling to 23 °C, the reaction mixture was filtered through Celite® and the solvent was removed under reduced pressure.
Conclusions
In summary, we developed a robust synthetic methodology to bench-stable bis-stannylated and bis-borylated diazocines in meta- and para-position to the azo group. These served precursors for Stille and Suzuki cross-coupling reactions with a broad scope of electrophiles. The catalytic system Pd(OAc)2/XPhos yielded good to excellent results and tolerated a wide range of functional groups. In this way, functionalized diazocines that might be of interest in material science or biological applications were straight-forward synthesized. While steric hindrance and electron lone pairs lowered the yield, this was observed for the Stille reaction to a smaller extent. Here, the reaction conditions could be easily adapted in order to obtain the desired product in a satisfying yield. Moreover, the coupling of anilines or phenols was possible using the Stille cross-coupling, while Buchwald–Hartwig type side reactions were observed under the Suzuki conditions. Overall, the Stille reaction produced better results in terms of isolated yields and in most cases less catalyst was needed. Nevertheless, other factors besides the yield have to be considered when planning a reaction. In fact, the Stille coupling outperformed the Suzuki coupling applying the here described conditions but the higher potential health risk of the Stille coupling due to the involvement of tin compounds should be mentioned. Depending on the scientific question, the use of the borylated compound for Suzuki coupling reactions might be preferred.
Author contributions
Conceptualization, M. W., W. K. and A. S.; methodology, M. W. and W. K.; validation, M. W., W. K. and A. S.; formal analysis, M. W., W. K. and R. R.; investigation, M. W., W. K. and R. R.; resources, A. S.; data curation, M. W. and W. K.; writing—original draft preparation, M. W. and W. K.; writing—review and editing, M. W., W. K., R. R. and A. S.; visualization, M. W. and W. K.; supervision, A. S.; project administration, A. S.; funding acquisition, A. S. all authors have read and agreed to the published version of the manuscript.
Conflicts of interest
There are no conflicts to declare.
Acknowledgements
The authors are grateful to Amelie Sprengel for measuring IR spectra.
Notes and references
-
(a) R. Siewertsen, H. Neumann, B. Buchheim-Stehn, R. Herges, C. Näther, F. Renth and F. Temps, J. Am. Chem. Soc., 2009, 131, 15594–15595 CrossRef CAS PubMed;
(b) C. Deo, N. Bogliotti, R. Métivier, P. Retailleau and J. Xie, Chem.–Eur. J., 2016, 22, 9092–9096 CrossRef CAS PubMed;
(c) M. Hammerich, C. Schutt, C. Stahler, P. Lentes, F. Rohricht, R. Hoppner and R. Herges, J. Am. Chem. Soc., 2016, 138, 13111–13114 CrossRef CAS PubMed.
-
(a) M. H. Burk, S. Schröder, W. Moormann, D. Langbehn, T. Strunskus, S. Rehders, R. Herges and F. Faupel, Macromolecules, 2020, 53, 1164–1170 CrossRef CAS;
(b) M. H. Burk, D. Langbehn, G. Hernández Rodríguez, W. Reichstein, J. Drewes, S. Schröder, S. Rehders, T. Strunskus, R. Herges and F. Faupel, ACS Appl. Polym. Mater., 2021, 3, 1445–1456 CrossRef CAS;
(c) S. Li, R. Colaco and A. Staubitz, ACS Appl. Polym. Mater., 2022, 4, 6825–6833 CrossRef CAS.
- S. Li, G. Han and W. Zhang, Macromolecules, 2018, 51, 4290–4297 CrossRef CAS.
-
(a) G. Cabré, A. Garrido-Charles, À. González-Lafont, W. Moormann, D. Langbehn, D. Egea, J. M. Lluch, R. Herges, R. Alibés, F. Busqué, P. Gorostiza and J. Hernando, Org. Lett., 2019, 21, 3780–3784 CrossRef PubMed;
(b) J. Ewert, L. Heintze, M. Jorda-Redondo, J. S. von Glasenapp, S. Nonell, G. Bucher, C. Peifer and R. Herges, J. Am. Chem. Soc., 2022, 144, 15059–15071 CrossRef CAS PubMed;
(c) M. Reynders, B. S. Matsuura, M. Bérouti, D. Simoneschi, A. Marzio, M. Pagano and D. Trauner, Sci. Adv., 2020, 6, eaay5064 CrossRef CAS PubMed;
(d) S. Samanta, C. Qin, A. J. Lough and G. A. Woolley, Angew. Chem., Int. Ed., 2012, 51, 6452–6455 CrossRef CAS PubMed.
- S. Li, K. Bamberg, Y. Lu, F. D. Sönnichsen and A. Staubitz, Polymers, 2023, 15, 1306 CrossRef CAS PubMed.
- M. Walther, W. Kipke, S. Schultzke, S. Ghosh and A. Staubitz, Synthesis, 2021, 53, 1213–1228 CrossRef CAS.
-
(a) W. Moormann, D. Langbehn and R. Herges, Synthesis, 2017, 49, 3471–3475 CrossRef CAS;
(b) H. Sell, C. Nather and R. Herges, Beilstein J. Org. Chem., 2013, 9, 1–7 CrossRef CAS PubMed;
(c) F. Klockmann, C. Fangmann, E. Zender, T. Schanz, C. Catapano and A. Terfort, ACS Omega, 2021, 6, 18434–18441 CrossRef CAS PubMed;
(d) W. Moormann, D. Langbehn and R. Herges, Beilstein J. Org. Chem., 2019, 15, 727–732 CrossRef CAS PubMed;
(e) D. K. Joshi, M. J. Mitchell, D. Bruce, A. J. Lough and H. Yan, Tetrahedron, 2012, 68, 8670–8676 CrossRef CAS.
-
(a) M. S. Maier, K. Hull, M. Reynders, B. S. Matsuura, P. Leippe, T. Ko, L. Schaffer and D. Trauner, J. Am. Chem. Soc., 2019, 141, 17295–17304 CrossRef CAS PubMed;
(b) J. Wang, J. He, C. Zhi, B. Luo, X. Li, Y. Pan, X. Cao and H. Gu, RSC Adv., 2014, 4, 16607–16611 RSC.
- S. Li, N. Eleya and A. Staubitz, Org. Lett., 2020, 22, 1624–1627 CrossRef CAS PubMed.
-
(a) J. B. Trads, K. Hull, B. S. Matsuura, L. Laprell, T. Fehrentz, N. Gorldt, K. A. Kozek, C. D. Weaver, N. Klocker, D. M. Barber and D. Trauner, Angew. Chem., Int. Ed., 2019, 58, 15421–15428 CrossRef CAS PubMed;
(b) N. Preußke, W. Moormann, K. Bamberg, M. Lipfert, R. Herges and F. D. Sönnichsen, Org. Biomol. Chem., 2020, 18, 2650–2660 RSC.
-
(a) M. Jun, D. K. Joshi, R. S. Yalagala, J. Vanloon, R. Simionescu, A. J. Lough, H. L. Gordon and H. Yan, ChemistrySelect, 2018, 3, 2697–2701 CrossRef CAS;
(b) E. R. Thapaliya, J. Zhao and G. C. R. Ellis-Davies, ACS Chem. Neurosci., 2019, 10, 2481–2488 CrossRef CAS PubMed;
(c) Q. Zhu, S. Wang and P. Chen, Org. Lett., 2019, 21, 4025–4029 CrossRef CAS PubMed;
(d) M. Reynders, A. Chaikuad, B. T. Berger, K. Bauer, P. Koch, S. Laufer, S. Knapp and D. Trauner, Angew. Chem., Int. Ed., 2021, 60, 20178–20183 CrossRef CAS PubMed;
(e) T. Ko, M. M. Oliveira, J. M. Alapin, J. Morstein, E. Klann and D. Trauner, J. Am. Chem. Soc., 2022, 144, 21494–21501 CrossRef CAS PubMed;
(f) H. Lee, J. Tessarolo, D. Langbehn, A. Baksi, R. Herges and G. H. Clever, J. Am. Chem. Soc., 2022, 144, 3099–3105 CrossRef CAS PubMed;
(g) S. Schultzke, M. Walther and A. Staubitz, Molecules, 2021, 26, 3916 CrossRef CAS PubMed.
- L. Heintze, D. Schmidt, T. Rodat, L. Witt, J. Ewert, M. Kriegs, R. Herges and C. Peifer, Int. J. Mol. Sci., 2020, 21, 8961 CrossRef CAS PubMed.
-
(a) J. Strueben, P. J. Gates and A. Staubitz, J. Org. Chem., 2014, 79, 1719–1728 CrossRef CAS PubMed;
(b) J. Strueben, M. Lipfert, J.-O. Springer, C. A. Gould, P. J. Gates, F. D. Sönnichsen and A. Staubitz, Chem.–Eur. J., 2015, 21, 11165–11173 CrossRef CAS PubMed.
- A. R. Katritzky, J. Wu and S. V. Verin, Synthesis, 1995, 1995, 651–653 CrossRef.
-
(a) G. Wang, H. Zhang, J. Zhao, W. Li, J. Cao, C. Zhu and S. Li, Angew. Chem., Int. Ed., 2016, 55, 5985–5989 CrossRef CAS PubMed;
(b) M. Song, H. Zhou, G. Wang, B. Ma, Y. Jiang, J. Yang, C. Huo and X. C. Wang, J. Org. Chem., 2021, 86, 4804–4811 CrossRef CAS PubMed.
- T. Ishiyama, M. Murata and N. Miyaura, J. Org. Chem., 1995, 60, 7508–7510 CrossRef CAS.
- J. R. Naber and S. L. Buchwald, Adv. Synth. Catal., 2008, 350, 957–961 CrossRef CAS.
|
This journal is © The Royal Society of Chemistry 2023 |