DOI:
10.1039/D3RA02813E
(Paper)
RSC Adv., 2023,
13, 19039-19045
Enhanced stability and tunable photoluminescence in Mn2+-doped one-dimensional hybrid lead halide perovskites for high-performance white light emitting diodes†
Received
28th April 2023
, Accepted 19th June 2023
First published on 22nd June 2023
Abstract
Organic–inorganic hybrid low-dimensional lead halides have garnered significant interest in the realm of solid-state optical materials due to their unique properties and potential applications. In this study, we report the synthesis, characterization and application of Mn2+-doped one-dimensional (1D) [AEP]PbCl5·H2O hybrid lead halide perovskites with tunable photoluminescence properties. The Mn2+ doping leads to a redshift of the dominant emission wavelength from 463 nm to 630 nm, with the optimal doping concentration resulting in an enhanced photoluminescence quantum yield (PLQY) from less than 1% to 8.96%. The structural and optical stability of these doped perovskites have been thoroughly investigated revealing excellent performance under humid and high-temperature conditions. Perovskite–PVP composite films exhibit high crystallization and bright orange-red emission under UV excitation. Furthermore, we demonstrate the successful fabrication of a white LED device using the Mn2+-doped perovskite in combination with commercial green and blue phosphors. The fabricated LED exhibits a high color rendering index (CRI) of 87.2 and stable electroluminescence performance under various operating currents and extended operation times. Our findings highlight the potential of Mn2+-doped 1D hybrid lead halide perovskites as efficient and stable phosphors for high-performance white light emitting diodes and other optoelectronic applications.
Introduction
Lead trihalide-based three-dimensional (3D) perovskite materials (APbX3, A = Cs+, methylammonium; X = Cl, Br, I) have garnered significant research interest for various optoelectronic devices, including high-efficiency solar cells, color-tunable and narrow linewidth light-emitting diodes (LEDs), lasers, and photodetectors.1–8 These materials represent a new paradigm for renewable energy sources due to their exceptional charge carrier mobilities, low density of midgap trap states, and defect-tolerant nature. Furthermore, 3D APbX3-based materials offer potential applications in solid-state lighting and display technologies, owing to their attractive optical properties, such as high PLQY, tunable bandgaps, emission wavelengths spanning the entire visible spectrum, narrow linewidths and rapid radiation decay rates.9–12 Nonetheless, 3D perovskites have some remaining drawbacks including moisture absorption, water decomposition, and limited thermal stability, which impede their use in optoelectronics. Low-dimensional hybrid metal halide perovskites present an alternative to 3D perovskites, offering benefits such as tunable emission wavelength, enhanced PLQY, improved moisture resistance, and thermal stability.13–22
The numerous advantages of low-dimensional perovskites have driven researchers to optimize their luminescent properties through various modulation strategies. Among these, replacing lead with non-toxic metal ions has emerged as a promising approach for tuning photoluminescent properties.23–28 Metal ions such as Mn2+, Zn2+, Cd2+, and rare earth ions have successfully replaced some Pb ions, with Mn2+ being particularly attractive due to its high luminescence efficiency and non-toxicity.29–34 Mn2+ doping has been extensively studied in 0D, 2D, 3D and bimetallic perovskites showcasing great potential for optoelectronic device applications like light-emitting diodes, thanks to their enhanced stability and exciton binding energy. Recent research has demonstrated the successful incorporation of Mn ions into lead perovskite nanocrystals, revealing the influence on energy transfer between the Mn ion and semiconducting host. The successful single-site synthesis of colloidal Mn-doped cesium halide lead perovskite nanocrystals has resulted in intensive Mn2+ associated luminescence, suggesting that CsPbX3 nanocrystals could serve as a novel platform for magnetically doped quantum dots.35–37 These achievements in 3D perovskites inspire us to employ similar methods to optimize the luminescent properties of low-dimensional perovskites.
Liu and colleagues introduce a novel family of doped nanocrystals (NCs) that offer valuable insights into the chemical mechanisms of doping and the fundamental interactions between dopant and semiconductor hosts. Specifically, they have developed an effective method for incorporating Mn ions into lead halide perovskite NCs by examining the role of relative bond strengths within the lattice of precursors and hosts. They observed a striking impact of halide properties on the relative strength of intrinsic band edges and Mn emission bands, which they attribute to the effect of the energy difference between corresponding transitions on the energy transfer properties between the Mn ion and the semiconductor host.38
David and his colleagues report the single-site synthesis of colloidal Mn-doped cesium halide lead (CsPbX3) perovskite NCs, resulting in effective intraparticle energy transfer between excitons and dopant ions, leading to intense sensitized Mn luminescence. At a doping concentration of 0.2%, the Mn-doped CsPbCl3 and CsPb(Cl/Br)3 nanocrystals retained the same lattice structure and crystallinity as their undoped counterparts, with nearly identical lattice parameters and no signs of phase separation. They found that CsPbX3 NCs possess numerous superior optical and electronic properties, potentially serving as a new platform for magnetically doped quantum dots and extending the range of optical, electronic, and magnetic functions. The significant achievement of manganese doping in 3D perovskites inspired the researchers to adopt similar methods for optimizing the luminescence properties of low-dimensional perovskites.39
In this study, we synthesized a 1D bulk crystal [AEP]PbCl5·H2O using the ambient temperature volatilization method, but the luminescence is extremely weak. To enhance the PLQY of this compound, we doped Mn2+ into the synthesized 1D bulk crystal. We found that the Mn2+-doped 1D perovskite exhibited efficient and tunable broadband luminescence from the blue to red spectral range, with the highest PLQY reaching 8.96%. DFT calculation reveal that the energy transfer between the host lattice and Mn2+ ions, referred to as Förster Resonance Energy Transfer (FRET), plays a crucial role in the observed redshift. This energy transfer process involves the absorption of photons by the host lattice, followed by a non-radiative transfer of energy to the Mn2+ ions and then radiative recombination from Mn2+ center. Moreover, systematic studies revealed that these Mn2+-doped perovskites demonstrated excellent structural and optical stability under both humid atmospheric and high-temperature conditions. This research not only deepened our understanding of the Mn doping strategy but also offered a novel approach for improving the luminescence performance of perovskite materials.
Experimental section
Materials
PbCl2 (99.7%, Aladdin), HCl (37%, Aladdin), MnBr2·4H2O (Aladdin), 1-(2-aminoethyl)piperazine (99.9%, Aladdin). All chemicals were purchased and used without any further purification.
Synthesis of [AEP]PbCl5·H2O (compound 1)
A mixture of PbCl2 (0.5 mmol, 0.1391 g) and 1-(2-aminoethyl)piperazine (0.6 mmol, 0.08 g) was dissolved in 5 mL of HCl under vigorous stirring, followed by heating to 100 °C for approximately 5 minutes until a clear solution formed. The reactants were then cooled to room temperature at a cooling rate of 5 °C min−1, and transparent crystals were obtained after maintaining the solution at room temperature for 5 days. The crystals were filtered from the solution and washed three times with ethanol, resulting in the colorless crystal of [AEP]PbCl5·H2O with a yield of 45%. Elemental analysis calculations for C12N6H38OPb2Cl10 showed C, 13.71%, N, 7.99%, H, 3.64%, while the obtained results were: C, 13.15%, N, 8.24%, H, 3.94%.
Synthesis of [AEP]Pb1−xMnxCl5·H2O
To prepare a series of [AEP]Pb1−xMnxCl5·H2O phases (compound 2–9), MnCl2·4H2O was used as the source of Mn2+ and doped into compound 1 using the above method. To rationally manage the doping concentration of Mn2+, the Mn2+/Pb2+ dosage ratio was continuously controlled from 0
:
1 to 20
:
1. The concentrations of dopant (manganese ions) in the [AEP]Pb1−xMnxCl5·H2O phases (compound 2–9) are listed in Table S4.†
X-ray crystallography
Single-crystal X-ray diffraction data of [AEP]PbCl5·H2O were collected using a Bruker D8 Quest diffractometer with graphite-monochromated Mo Kα radiation (λ = 0.71073 Å) at 293 K. The structure was solved and refined by the SHELXL-2018/3 program within OLEX2, with all atoms being refined with anisotropic atomic displacement parameters, except the H atoms, which were placed in idealized positions and allowed to ride on the relevant carbon atoms. Detailed crystal data and structural refinement for compound 1 are summarized in Table S1.† Selected bond lengths (Å) and bond angles (°) are listed in Table S2,† and hydrogen bond data are collected in Table S3.†
Characterization methods
The powder X-ray diffraction (PXRD) pattern was collected using a Rigaku MiniFlex II diffractometer, operating at 40 kV and 40 mA with Cu Kα radiation (λ = 1.5406 Å). The diffraction pattern was scanned over the angular range of 5–60° (2θ) with a step size of 5° min−1 at room temperature. Thermogravimetric analysis (TGA) was carried out using a Netzsch STA 449C thermal system, with samples heated from 35 to 800 °C at a rate of 20 °C min−1 under an N2 atmosphere. Solid-state UV-vis optical absorption spectra were performed on a PE Lambda 900 UV-vis spectrophotometer. X-ray photoelectron spectroscopy (XPS) spectra were tested using the Escalab XI+ instrument, USA. SEM and EDX images were obtained using the Hitachi SU-8010 field emission scanning electron microscopy.
Photoluminescence characterization
The PL spectrum was measured using an Edinburgh FLS1000 fluorescence spectrometer. The CIE chromaticity coordinates were calculated using CIE calculator software based on the emission spectra. The PLQY was acquired using an Edinburgh FLS980 fluorescence spectrometer equipped with a xenon lamp and a calibrated integrating sphere. PLQYs were calculated using the equation: ηQE = IS/(ER − ES), where IS represents the luminescence emission spectrum of the sample, ER is the spectrum of the excitation light from the empty integrated sphere (without the sample), and ES is the excitation spectrum for exciting the sample. Time-resolved attenuation data were collected using the Edinburgh FLS980 fluorescence spectrometer and a picosecond pulsed diode laser.
Fabrication of WLED
The red [AEP]Pb1−xMnxCl5·H2O phosphor, commercial green phosphor of (Ba, Sr)SiO4:Eu2+ (Shenzhen Looking Long Technology Co., Ltd, China), commercial blue phosphor of BaMgAl10O17:Eu2+ (Shenzhen Looking Long Technology Co., Ltd, China), and UV chip (365 nm, 3 V, 300 mA, Shenzhen Looking Long Technology Co., Ltd, China) were thoroughly mixed using an epoxy resin. The resulting mixture was then coated on the LED chip. The electrical and photoelectric properties, such as the emission spectrum, color temperature (CCT), color rendering index (Ra), and CIE color coordinates of the LED, were measured using an integrating sphere spectroradiometer system (EVERFINE HAAS-2000). The as-fabricated white LEDs were operated at 3 V with varied drive currents ranging from 20 to 120 mA.
Preparation of perovskite–PVP composites
To prepare the composites, 0.30 g of sample powder was dissolved in 1 mL of ethanol (EA) to obtain a homogeneous suspension solution. Next, 0.5 g of polyvinylpyrrolidone (PVP) was added to the solution and stirred at room temperature for 4 hours, resulting in a white viscous liquid. Finally, a thin film of [AEP]Pb1−xMnxCl5·H2O was obtained by spin-coating 50 μL of the viscous liquid onto a glass substrate at a speed of 2100 rpm.
Results and discussion
Crystal structure
Bulk single crystals of 1D [AEP]PbCl5·H2O (1) were obtained through the facile solution evaporation method using 1-(2-aminoethyl)piperazine and PbCl2 as precursors in hydrobromic acid (Fig. 1a). The single crystal structure of compound 1 was determined by X-ray single-crystal diffraction, and it crystallized in the monoclinic space group P21/c. The asymmetric unit of compound 1 comprises a 1D infinite zigzag [PbCl5]3− chain, two [AEP]3+ cations, and a guest water molecule. The octahedral [PbCl6] structure exhibits slight distortion with the six Pb–Cl bond lengths ranging from 2.7713(10) to 3.05845(17) Å (Fig. 1b). The Pb(1)Cl6 and Pb(2)Cl6 units are interlinked by sharing two adjacent Cl atoms from each [PbCl6] octahedron to form a 1D zigzag chain, which is distinct from the approximately linear [PbCl5]3− chain in (2.6-dmpz)3Pb2Cl10 (Fig. 1c).40,41 The neighboring 1D [PbCl5]3− chains stack closely in the [010] plane and are separated and surrounded by bulk [AEP]3+ cations through weak N–H⋯Cl hydrogen bonds, forming a 3D H-bonding network. Consequently, this hybrid perovskite represents an ideal inorganic–organic hybrid phase, where 1D chains are individually encapsulated within a wide bandgap organic matrix. This unique structure allows the hybrid to exhibit distinct physical properties associated with the inorganic [PbCl5]3− species. The powder X-ray diffraction (PXRD) pattern of the bulk crystals aligns with the simulation derived from single-crystal data, confirming the consistency of the crystal lattice and the high purity of the sample (Fig. S1†).
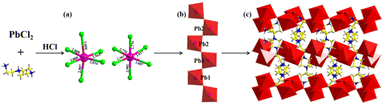 |
| Fig. 1 Schematic diagram of the synthesis of [AEP]PbCl5·H2O (1): (a) the coordination environments of [PbCl6]4− units; (b) the structure of 1D [PbCl5]3− chain; (c) the packing crystal structure of [AEP]PbCl5·H2O viewed along the c-axis. | |
A series of [AEP]Pb1−xMnxCl5·H2O phases (compounds 2–8) were synthesized by doping Mn2+ into compound 1. The Mn2+-doped compounds were systematically characterized using powder X-ray diffraction (PXRD), X-ray photoelectron spectroscopy (XPS), energy-dispersive X-ray spectroscopy (EDX), and elemental mapping. The PXRD patterns of the [AEP]Pb1−xMnxCl5·H2O series with varying Mn2+ concentrations are consistent with that of the undoped [AEP]PbCl5·H2O, indicating the same crystal structure without the presence of unwanted secondary phases. The diffraction peaks feature slight shift toward high angle with the increasing of Mn2+ content, which corresponds to lattice shrinkage and demonstrates success of Mn2+ doping (Fig. 2a). XPS analysis verifies the presence of C, N, Pb, and Cl elements, and the distinct peaks of Pb 4f, Cl 2p, C 1s, and N 1s orbitals suggest a single species for all components (Fig. 2b–f). The Mn 2p3/2 orbital energy is observed in the range of 641.05–645.95 eV, corresponding to the Mn2+ ion, which demonstrates the successful incorporation of Mn2+ ions into the 1D [AEP]PbCl5·H2O structure (Fig. 2g). Furthermore, the EDX analysis indicates that the Mn element has been successfully doped into compound 1 (Fig. S2†).
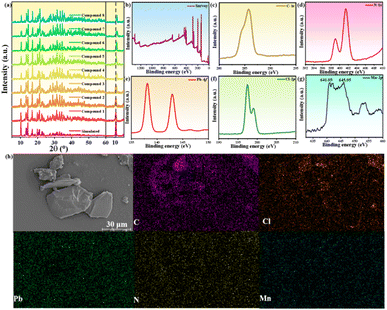 |
| Fig. 2 Characterizations of Mn2+-doped [AEP]PbCl5·H2O: (a) normalized XRD patterns; (b–g) XPS spectra; (h) SEM elemental mapping images of C, N, Pb, Cl and Mn for compound 8. | |
The elemental mapping images further reveal that the elements C, N, Mn, Pb, and Cl are homogeneously distributed on the surface of the doped phase, confirming the composition uniformity (Fig. 2h). Following comprehensive characterization, it has been established that Mn2+ has successfully replaced a portion of Pb2+ in [AEP]PbCl5·H2O, leading to the formation of a doped 1D perovskite with tunable Mn2+ concentrations (Fig. S3†). Notably, this doped perovskite retains an identical crystal structure and uniform elemental distribution, offering an exceptional structural platform for investigating the dynamic photophysical properties of the Mn2+-doped 1D perovskite.
Luminescence properties
The luminescence properties of compound 1 were investigated using solid-state absorption, steady-state photoluminescence (PL) excitation and emission, and time-resolved spectroscopy at 300 K. The absorption spectrum of compound 1 exhibits a strong excitonic peak in the range of 200–350 nm (Fig. S4†). Under 365 nm UV light irradiation, bulk crystals of 1D [AEP]PbCl5·H2O emit a faint blue-white light, similar to previous reports (Fig. 3a).42 The PL excitation spectrum features a sharp peak at 360 nm, which is close to the maximum absorption peak of compound 1, indicating intrinsic excitonic emission. Upon 365 nm UV light excitation, compound 1 emits a broadband blue-white light with a single maximum at 463 nm, wide full-width at half-maximum (FWHM) of 152.9 nm, large Stokes shift of 103 nm, and CIE chromaticity coordinates of (0.254, 0.303) (Fig. 3b and c). The weak blue-white light emission is verified by the measured PLQY of less 1% (Fig. S5†). The PL decay curve monitored at 463 nm gives an average lifetime of 1.36 ns at 300 K (Fig. 3d).
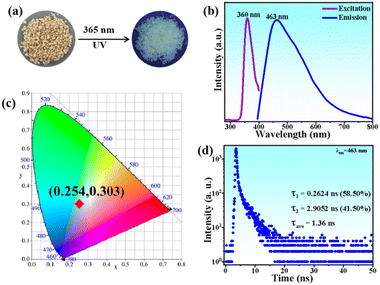 |
| Fig. 3 PL characterizations of compound 1 at 300 K: (a) photo images of bulk crystals under ambient light and UV light; (b) excitation and emission spectra; (c) Commission Internationale de l'Eclairage (CIE) chromaticity coordinates; (d) PL decay curve monitored at 463 nm. | |
The emission color of the compound 1 changes from faint blue-white to bright orange-red under UV light illumination as Mn2+ is continuously doped (Fig. 4a). As the Mn2+ content increases, the compounds exhibit broad absorption bands in the 400–550 nm range, which is attributed to the electron transition within d5 orbitals of Mn2+ ions (Fig. S4†). The steady-state PL emission spectra showed a red-shift to the dominant emission wavelength from 466 nm to 630 nm with increasing Mn2+ addition (Fig. 4b). The luminescence transformation from blue-white (0.20, 0.22) to red light (0.59, 0.36) is evident in the CIE chromaticity coordinates (Fig. 4c). To evaluate emission intensity and energy-transfer efficiency, we measure the PLQYs of serial Mn2+-doped [AEP]PbCl5·H2O (Fig. S5†). The PLQY increases monotonously to 8.96% as the Mn2+ content increases up to 3.13% (Fig. 4d). After elevating the dopant concentration to 4.62%, the emission intensity began to decline resulting from the concentration quenching effect triggered by the nonradiative energy transfer between the dopants. The critical distance between the adjacent dopants can be employed to justify the concentration quenching mechanism and it can be calculated through the following expression:43
|
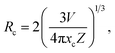 | (1) |
where
Rc,
V,
xc, and
Z describe the critical distance, volume of unit cell, critical doping content, and the amount of host cations in the unit cell, respectively. In this case, the values of
V,
xc, and
Z were 3050.3, 0.0313, and 4, respectively. Utilizing
eqn (1), one finds that the critical distance of Mn
2+ ions in the compound
9 was 35.97 Å. Usually, the concentration quenching mechanism will be contributed by the exchange interaction when the critical distance between the dopants is less than 5 Å, otherwise, it will be pertained by the multipole–multipole interaction when
Rc > 5 Å. As the critical distance of Mn
2+ ions in the compound
9 host lattice were much larger than 5 Å, it is reasonable to conclude that the multipole–multipole interaction would contribute to the aforementioned concentration quenching mechanism. Time-resolved PL decay curve monitoring at 619 nm is fitted with a double-exponential function, yielding an average lifetime of 0.2879 μs for compound
8 (Fig S6
†).
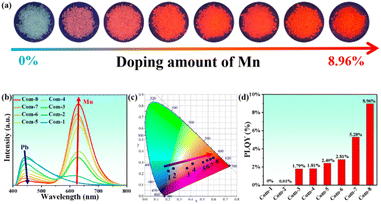 |
| Fig. 4 PL characterizations of serial Mn2+-doped [AEP]PbCl5·H2O at 300 K: (a) photo images of bulk crystals under UV light; (b) consecutive tunable PL emission spectra; (c) CIE chromaticity coordinates, and (d) PLQYs for selected compounds 1–8. | |
According to the PXRD patterns, the crystal structure of Mn2+-doped 1D [AEP]PbCl5·H2O were not changed (Fig. 5a and d). According the band structure and density of state by DFT calculation (Fig. 5b–f), the redshift of PL emission of Mn2+-doped 1D [AEP]PbCl5·H2O is mainly due to the energy transfer between the host material and Mn2+ ions, and the increased incorporation of Mn2+ ions into the lattice. When Mn2+ ions are introduced into the 1D [AEP]PbCl5·H2O lattice, they act as dopants and occupy the Pb2+ sites. This substitution leads to the formation of Mn2+-related energy levels within the host material's bandgap (Fig. 5f). The energy transfer between the host lattice and Mn2+ ions, often referred to as Förster Resonance Energy Transfer (FRET), plays a crucial role in the observed redshift. This energy transfer process involves the absorption of photons by the host lattice, followed by a non-radiative transfer of energy to the Mn2+ ions. The Mn2+ ions then relax back to their ground state, emitting photons at a lower energy (longer wavelength) than the initially absorbed photons. This phenomenon results in the observed redshift of the photoluminescence in the Mn2+-doped 1D [AEP]PbCl5·H2O material (Fig S6†).
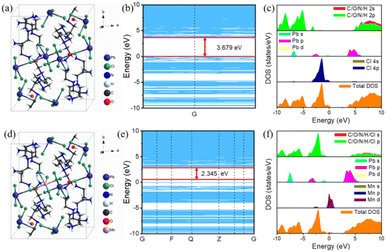 |
| Fig. 5 DFT calculation for pristine and Mn2+-doped 1D [AEP]PbCl5·H2O. (a) Unit cell structure, (b) the calculated band structures, and (c) the total and partial density of states for 1D [AEP]PbCl5·H2O. (d) Unit cell structure, (e) the calculated band structures, and (f) the total and partial density of states for Mn2+-doped 1D [AEP]PbCl5·H2O. | |
As the concentration of Mn2+ ions increase, the probability of FRET occurring also increases. Consequently, the steady-state PL emission spectra exhibit a gradual redshift of the dominant emission wavelength from 463 nm to 630 nm with the increasing addition of Mn2+ precursor. Additionally, as the Mn2+ doping concentration increases, the local crystal field around the Mn2+ ions may change, further influencing the emission wavelength and causing a redshift. It is important to note that the energy transfer process can also lead to luminescence quenching if the Mn2+ doping concentration is too high. This is due to the increased likelihood of non-radiative relaxation processes taking place, which can reduce the overall photoluminescence quantum yield. In this study, the highest PLQY of 8.96% was achieved by optimizing the Mn2+ doping concentration to balance the redshift and emission intensity.
Stability property
Stability is a crucial factor for luminescent perovskite materials in photoelectric applications, leading to extensive research in this area. After investigating the photoluminescence (PL) properties of compounds 1–8, we focused on their structural and optical stability in various chemical and physical environments. To assess the stability of the samples in humid environments, we stored them in ambient air with a high humidity of 90% for two months. Comparing the PL emission spectra of compound 8 before and after storage, we found that the PL spectra remained consistent with nearly identical emission intensity (Fig. S7†). This suggests that these compounds exhibit good stability in humid environments.
We also studied the thermal stability of the perovskites, as optoelectronic devices often operate continuously at high temperatures. Thermogravimetric analysis revealed that these 1D perovskites could be stabilized below 160 °C (Fig. S8†). To further investigate their thermal stability, we analyzed the temperature-dependent PXRD patterns of samples after constant heating (Fig. S9 and S10†). The unchanged PXRD patterns and PL spectra indicate the sufficient thermal stability. We then prepared perovskite–PVP composites by mixing compound 8 and polyvinylpyrrolidone in ethanol, and the film was deposited by one-step spin-coating from the precursor solution (Fig. S11†). The deposited perovskite–PVP film showed excellent crystallization and emitted a bright orange-red light under UV excitation with a broadband emission spectrum similar to compound 8 (Fig. S11b–d†). The PXRD pattern of the deposited film matched the simulated result from single-crystal structural data, indicating a high degree of crystallization. In summary, the remarkable stability of compound 8 offers possibilities for its application in photoelectric performance.
Applications in solid-state LEDs
The successful assembly of various luminescent devices based on compound 8 has inspired us to further explore its application in solid-state LEDs. To demonstrate this performance, we fabricated a white LED by coating a mixture of red-light emitter (compound 8) with commercial green ((Ba, Sr) SiO4:Eu2+) and blue (BaMgAl10O17:Eu2+) phosphors on a 365 nm UV chip. Driven by a 20 mA current, the white LED device emits bright white light, further characterized by electroluminescence (EL) spectroscopy at room temperature (Fig. 6a). The EL emission spectrum spans the entire visible region, with CIE chromaticity coordinates of (0.315, 0.336) and a correlated color temperature (CCT) of 6327 K, corresponding to “warm” white light. Moreover, even without precise control of the mixing ratio of the three phosphors, the broadband EL emission spectrum of the white LED achieves a high color rendering index (CRI) of 87.2, surpassing commercial white LEDs based on YAG:Ce3+ (CRI < 80). Operating current-dependent EL was further performed to evaluate the spectral stability of the white LED. The EL emission intensity steadily increases with the enhanced current from 20 to 120 mA, indicating the absence of energy transfer between different phosphors and potential application in high-power optoelectronic devices (Fig. 6b and c). Surprisingly, under constant drive for at least 360 minutes, the fabricated white LED still exhibits high emission intensity without significant reduction (Fig. 6d), further demonstrating the excellent thermal and optical stability of the fabricated white LED device.
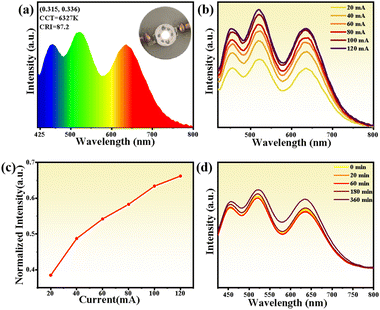 |
| Fig. 6 (a) The PL spectra of the white LED fabricated using compound 8 at a 20 mA drive current (inset: photograph of the fabricated white LEDs); (b) drive current dependent PL spectra of the fabricated white LED device; (c) normalized peak intensity variation of the fabricated white LED under various operating currents; (d) operating time-dependent PL spectra of the fabricated white LED devices. | |
Conclusions
In conclusion, we demonstrate the synthesis and characterization of Mn2+-doped one-dimensional [AEP]PbCl5·H2O hybrid lead halide perovskites. The incorporation of Mn2+ ions as dopants in the perovskite lattice leads to tunable photoluminescence properties, resulting in a redshift of the dominant emission wavelength. The optimized doping concentration yields an enhanced PLQY from less than 1% to 8.96%, making these materials promising candidates for optoelectronic applications. The structural and optical stability of the doped perovskites under humid and high-temperature conditions have been thoroughly investigated, revealing their excellent performance under adverse environmental conditions. The perovskite–PVP composite films also exhibit high crystallization and bright orange-red emission under UV excitation. Furthermore, a WLED device has been fabricated using the Mn2+-doped perovskite in combination with commercial green and blue phosphors. The fabricated LED exhibits a high color rendering index of 87.2 and stable electroluminescence performance under various operating currents and extended operation times. This study highlights the potential of Mn2+-doped one-dimensional hybrid lead halide perovskites as efficient and stable phosphors for high-performance white light emitting diodes and other optoelectronic applications.
Conflicts of interest
There are no conflicts to declare.
Acknowledgements
We express thanks for the financial supports from the National Nature Science Foundation of China (No. 22201099 and 21601104) and the Shandong Provincial Natural Science Foundation (ZR2021QB204).
References
- H. B. Cho, J. W. Min, H. J. Kim, N. S. M. Viswanath, T. Samanta, J. H. Han, Y. M. Park, S. W. Jang and W. B. Im, ACS Appl. Electron. Mater., 2023, 5, 66–76 CrossRef CAS.
- J. K. Meyers, S. Kim, D. J. Hill, E. E. M. Cating, L. J. Williams, A. S. Kumbhar, J. R. McBride, J. M. Papanikolas and J. F. Cahoon, Nano Lett., 2017, 17, 7561–7568 CrossRef CAS PubMed.
- C. Xie, C. K. Liu, H. L. Loi and F. Yan, Adv. Funct. Mater., 2020, 30, 1903907 CrossRef CAS.
- J. Y. Li, Z. Y. Han, Y. Gu, D. J. Yu, J. X. Liu, D. W. Hu, X. B. Xu and H. B. Zeng, Adv. Funct. Mater., 2021, 31, 2008684 CrossRef CAS.
- S. Y. Zhao, W. S. Cai, H. X. Wang, Z. G. Zang and J. Z. Chen, Small Methods, 2021, 5, 2001308 CrossRef CAS PubMed.
- W. Ma, J. L. Yin, X. F. Chen, C. Sun, X. L. Song and H. H. Fei, Chem. Mater., 2022, 34, 4403–4413 CrossRef CAS.
- F. F. Gao, X. Li, Y. Qin, Z. G. Li, T. M. Guo, Z. Z. Zhang, G. D. Su, C. Y. Jiang, M. Azeem, W. Li, X. Wu and X. H. Bu, Adv. Opt. Mater., 2021, 9, 2100003 CrossRef CAS.
- J. H. Fan, Y. Qin, M. Azeem, Z. Z. Zhang, Z. G. Li, N. Sun, Z. Q. Yao and W. Li, Dalton Trans., 2021, 50, 2648–2653 RSC.
- S. Mukherjee, J. Selvaraj and T. Paramasivam, ACS Appl. Nano Mater., 2021, 4, 10228–10243 CrossRef CAS.
- I. Lignes, R. M. Maceiczyk, M. V. Kovalenko and S. Stavrakis, Chem. Mater., 2020, 32, 27–37 CrossRef.
- P. Vashishtha, S. Bishnoi, C. H. A. Li, M. Jagadeeswararao, T. J. N. Hooper, N. Lohia, S. B. Shivarudraiah, M. S. Ansari, S. N. Sharma and J. E. Halpert, ACS Appl. Electron. Mater., 2020, 2, 3470–3490 CrossRef CAS.
- F. Palazon, F. D. Stasio, Q. A. Akkerman, R. Krahne, M. Prato and L. Manna, Chem. Mater., 2016, 28, 2902–2906 CrossRef CAS PubMed.
- K. Hong, Q. V. Le, S. Y. Kim and H. W. Jang, J. Mater. Chem. C, 2018, 6, 2189–2209 RSC.
- H. Peng and B. S. Zou, J. Phys. Chem. Lett., 2022, 13, 1752–1764 CrossRef CAS PubMed.
- C. Y. Yue, C. Sun, D. Y. Li, Y. H. Dong, C. L. Wang, H. F. Zhao, H. Jiang, Z. H. Jing and X. W. Lei, Inorg. Chem., 2019, 58, 10304–10312 CrossRef CAS PubMed.
- Y. L. Mao, J. Zhang, Q. Q. Ren, M. S. Molokeev, G. J. Zhou and X. M. Zhang, J. Mater. Chem. C, 2022, 10, 17638–17645 RSC.
- Y. Han, S. J. Yue and B. B. Cui, Adv. Sci., 2021, 8, 2004805 CrossRef CAS PubMed.
- H. M. Cheng, C. Cao, S. Y. Teng, Z. N. Zhang, Y. Zhang, D. Y. Wang, W. S. Yang and R. G. Xie, Dalton Trans., 2023, 52, 1021–1029 RSC.
- Y. L. Jiang, J. l. Yin and H. H. Fei, J. Mater. Chem. C, 2022, 10, 13254–13261 RSC.
- Y. C. Peng, H. W. Lin, S. H. Zhou, J. C. Jin, T. H. Zhuang, A. Ablez, Z. P. Wang, K. Z. Du and X. Y. Huang, Molecules, 2023, 28, 1978 CrossRef CAS PubMed.
- H. M. Yang, J. L. Yin, X. X. Xu and H. H. Fei, J. Mater. Chem. C, 2019, 7, 7090–7095 RSC.
- Y. C. Peng, S. H. Zhou, J. C. Jin, Q. Gu, T. H. Zhuang, L. K. Gong, Z. P. Wang, K. Z. Du and X. Y. Huang, Dalton Trans., 2022, 51, 4919–4926 RSC.
- C. Q. Jing, X. Yin, P. C. Xiao, Y. J. Gao, X. M. Wu, C. Y. Yue and X. W. Lei, Chem.–Eur. J., 2022, 28, e202103043 CrossRef CAS PubMed.
- P. Arunkumar, K. H. Gil, S. Won, S. Unithrattil, Y. H. Kim, H. J. Kim and W. B. Im, J. Phys. Chem. Lett., 2017, 8, 4161–4166 CrossRef CAS PubMed.
- L. Hou, Y. H. Zhu, J. R. Zhu, X. Y. Li, F. Zeng, T. Y. Yang, Y. Q. Gong, J. H. Shen and C. Z. Li, Adv. Mater. Interfaces, 2021, 8, 2002175 CrossRef CAS.
- A. Biswas, R. Bakthavatsalam and J. Kundu, Chem. Mater., 2017, 29, 7816–7825 CrossRef CAS.
- S. K. Dutta, A. Dutta, S. D. Adhikari and N. Pradhan, Chem. Mater., 2017, 29, 7816–7825 CrossRef.
- R. Bakthavatsalam, A. Biswas, M. Chakali, P. R. Bangal, B. P. Kore and J. Kundu, J. Phys. Chem. C, 2019, 123, 4739–4748 CrossRef CAS.
- Y. J. Guo, J. Su, L. Wang, Z. H. Lin, Y. Hao and J. J. Chang, J. Phys. Chem. Lett., 2021, 12, 3393–3400 CrossRef CAS PubMed.
- K. Xing, X. Yuan, Y. Wang, J. Li, Y. J. Wang, Y. Fan, L. Yuan, K. Li, Z. J. Wu, H. B. Li and J. L. Zhao, J. Phys. Chem. Lett., 2019, 10, 4177–4184 CrossRef CAS PubMed.
- M. H. P. Usman, R. Bakthavatsalam and J. Kundu, ChemistrySelect, 2018, 3, 6585–6595 CrossRef CAS.
- W. V. D. Stam, J. J. Geuchies, T. Altantzis, K. H. W. V. D. Bos, J. D. Meeldijk, S. V. Aert, S. Bals, D. Vanmaekelbergh and C. D. M. Donega, J. Am. Chem. Soc., 2017, 139, 4087–4097 CrossRef PubMed.
- C. K. Zhou, Y. Tian, O. Khabou, M. Worku, Y. Zhou, J. Hurley, H. R. Lin and B. W. Ma, ACS Appl. Mater. Interfaces, 2017, 9, 40446–40451 CrossRef CAS PubMed.
- A. K. Guria, S. K. Dutta, S. D. Adhikari and N. Pradhan, ACS Energy Lett., 2017, 2, 1014–1021 CrossRef CAS.
- D. Ricciarelli, D. Meggiolaro, P. Belanzoni, A. A. Alothman, E. Mosconi and F. D. Angelis, ACS Energy Lett., 2021, 6, 1869–1878 CrossRef CAS PubMed.
- G. J. Zhou, X. F. Jia, S. Q. Guo, M. Molokeev, J. Y. Zhang and Z. G. Xia, J. Phys. Chem. Lett., 2019, 10, 4706–4712 CrossRef CAS PubMed.
- Z. J. Li, E. Hofman, A. H. Davis, A. Khammang, J. T. Wright, B. Dzikovski, R. W. Meulenberg and W. W. Zheng, Chem. Mater., 2018, 30, 6400–6409 CrossRef CAS.
- W. Y. Liu, Q. L. Lin, H. B. Li, K. F. Wu, I. Robel, J. M. Pietryga and V. I. Klimov, J. Am. Chem. Soc., 2016, 138, 14954–14961 CrossRef CAS PubMed.
- D. Parobek, B. J. Roman, Y. Dong, H. Jin, E. Lee, M. Sheldon and D. H. Son, Nano Lett., 2016, 16, 7376–7380 CrossRef CAS PubMed.
- M. B. H. Salah, N. Mercier, M. Allain, N. Zouari and C. Botta, J. Mater. Chem. C, 2019, 7, 4424–4433 RSC.
- S. A. Fateev, A. A. Petrov, E. I. Marchenko, Y. V. Zubavichus, V. N. Khrustalev, A. V. Petrov, S. M. Aksenov, E. A. Goodilin and A. B. Tarasov, Chem. Mater., 2021, 33, 1900–1907 CrossRef CAS.
- J. Q. Zhao, C. Sun, M. Yue, Y. Meng, X. M. Zhao, L. R. Zeng, G. Chen, C. Y. Yue and X. W. Lei, Chem. Commun., 2021, 57, 1218–1221 RSC.
- P. Du, L. H. Luo and W. Cheng, J. Am. Ceram. Soc., 2019, 1–7 Search PubMed.
Footnote |
† Electronic supplementary information (ESI) available: Experimental, theoretical, analytical and crystallographic details. CCDC 2252459. For ESI and crystallographic data in CIF or other electronic format see DOI: https://doi.org/10.1039/d3ra02813e |
|
This journal is © The Royal Society of Chemistry 2023 |