DOI:
10.1039/D3RA02735J
(Paper)
RSC Adv., 2023,
13, 29242-29251
3-Hydroxyflavone is a mildly active and safe cobalt chelator while cobalt markedly enhances baicalein toxicity toward erythrocytes†
Received
25th April 2023
, Accepted 21st September 2023
First published on 5th October 2023
Abstract
Cobalt intoxication can occur after its release from metal-based prostheses, which is generally clinically severe. Therefore, there is a need for the development of a cobalt chelator since there are currently no approved drugs for cobalt intoxication. As flavonoids are known for their metal chelating properties and safety, the screening of cobalt chelating properties was performed in a total of 23 flavonoids by our recently developed new spectrophotometric assay. Further assessment of positive or negative consequences of cobalt chelation was performed both in vitro and ex vivo. Six and thirteen flavonoids significantly chelated cobalt ions at pH 7.5 and 6.8, respectively. Baicalein demonstrated a significant activity even at pH 5.5; however, none of the flavonoids showed chelation at pH 4.5. In general, baicalein and 3-hydroxyflavone were the most active. They also mildly decreased the cobalt-triggered Fenton reaction, but baicalein toxicity toward red blood cells was strongly increased by the addition of cobalt. Quercetin, tested as an example of flavonoid unable to chelate cobalt ions significantly, stimulated both the cobalt-based Fenton reaction and the lysis of erythrocytes in the presence of cobalt. Therefore, 3-hydroxyflavone can serve as a potential template for the development of novel cobalt chelators.
1 Introduction
Cobalt is an essential trace element, forming an integral part of vitamin B12. Its content in the human body is very low of approximately 1.1 mg,1 and this is reflected by its normal levels in the blood ranging from 0.04 to 0.9 μg L−1 (0.7–15.3 nM), with 95% of the population having below 0.6 μg L−1 (10.2 nM).2 Furthermore, cobalt can be very toxic when present in excess. Although its toxicity is rare, very severe intoxications have been recently observed due to its release from cobalt-containing hip prostheses.1–6 In two cases of such inadvertent cobalt release, the measured blood levels were in the range of approximately 400–500 μg L−1 (6.8–8.5 μM). Such levels are not merely associated with symptoms but might have even fatal consequences.3,6 The clinical manifestation of cobalt intoxication includes various symptoms, such as skin reactions, hypothyroidism, testicular atrophy, and CNS toxicity, including vision and hearing impairment at higher concentrations as well as reversible but rapidly progressive cardiomyopathy with a high short-term mortality.5,7–12 In addition, possible cancer development,13,14 and autoimmune, haematological and infectious diseases15 were associated with high cobalt levels. The inhalation of cobalt-containing dust was related to occupational asthma, heavy metal pneumoconiosis, pulmonary fibrosis, and the aforementioned cardiomyopathy.1,2,16 It should also be mentioned that isotope 60Co released by nuclear detonation has a half-life longer than 5 years and could hence negatively impact human health too.
Cobalt kinetics in the human body is rather disadvantageous as cobalt excretion is a very slow process during which only about 20% of cobalt is eliminated within the first 24 hours. The remainder bound to tissues and plasma albumin is excreted more slowly with an elimination half-life ranging from 6 to 800 days.1,17 Therefore, for the efficient treatment of cobalt toxicity, the acceleration of its elimination is a reasonable modality. Such approach requires an efficient and ideally selective cobalt chelator. However, there are currently no approved cobalt chelators by medicine agencies such EMA or FDA. The available studies report that chelation treatment of cobalt toxicity employing either 2,3-dimercapto-1-propanesulfonic acid6 or ethylenediaminetetraacetic acid (EDTA)18,19 did not give satisfactory results, as it suffered from adverse effects due to insufficient cobalt selectivity and slow cobalt elimination. Therefore, still there is a need to find more suitable and safe cobalt chelators.
Flavonoids are natural compounds with well-described metal chelation activities toward many pathophysiologically and toxicologically important metals including aluminium, cadmium, copper, iron, lead, nickel, and zinc.20 Notwithstanding extensive research which has been devoted to flavonoids, only few studies reported adverse reactions.21 They are hence considered safe and therefore reasonable candidates. Moreover, a lot of in vitro and in vivo studies have reported various health benefits of flavonoids.22,23 Historically, their profit was solely associated with their direct antioxidant activity. Currently, it is known that the antioxidant activity of flavonoids comprises not only reactive oxygen species (ROS) scavenging (direct antioxidant effect) but also the mentioned and repeatedly observed metal chelation and interferences with enzymes forming reactive oxygen species.24–26
Literature contains several reports describing that certain flavonoids can form complexes with cobalt,27–29 protecting various cell cultures against cobalt toxicity in vitro29–33 and also animals from the consequences of cobalt intoxication when given orally.34–38 These data are however insufficient for different reasons: (1) direct proofs of cobalt chelation are available for few compounds (quercetin, its glycoside rutin and 3-hydroxyflavone) or reported for mixed flavonoid–amine ligands,39 (2) these experiments were performed only under non-competitive conditions28,29 and did not report stability of those complexes, (3) besides its role as a direct pro-oxidant via participation in the Fenton reaction, cobalt stabilizes hypoxia-inducible factor 1α (HIF-1α) with subsequent induction of the synthesis of many proteins,1,4 and (4) orally administered flavonoids have a very low bioavailability.40 Consequently, current data are not able to prove that flavonoids are sufficiently strong cobalt chelators. Moreover, their protective effects in performed cell experiments might not only be based on cobalt chelation as they can be mediated by other mechanism(s). In addition, metal reduction by flavonoids can promote the Fenton reaction41,42 but such data are not available for cobalt. As far as we know, only one study tested the effect of a single flavonoid, quercetin, on that reaction but purely at a non-physiological basic pH.43
For the aforementioned reasons, the aim of our study was to analyse the interaction of 23 structurally related flavonoids with cobalt via a complex methodological approach developed by us.44 First, their ability to chelate cobalt ions was compared under four (patho)physiologically relevant pH conditions. To cover different structural features, we involved a relatively large series of flavonoids including some that were not expected to chelate cobalt. In the second step, their impact on the cobalt-based Fenton reaction was assessed, and in the third step, their impact on red blood cell lysis in the presence of cobalt was tested. The latter was selected as a suitable model since erythrocytes do not possess the nucleus, and hence, possible anti- or prooxidant effects are not affected by adaptation due to gene expression.
2 Materials and methods
2.1 Reagents, solutions, and equipment
Cobalt chloride, 1-nitroso-2-naphthol-3,6-disulfonic acid disodium salt (NNDSA), nitroxoline, disodium salt of ethylenediaminetetraacetic acid (EDTA), sodium acetate, acetic acid, 4-(2-hydroxyethyl)-1-piperazineethanesulfonic acid (HEPES), HEPES sodium salt, catechol, 2,3-dihydroxybenzoic acid (2,3-DHBA), 2,5-dihydroxybenzoic acid (2,5-DHBA), salicylic acid, H2O2 (30%), orthophosphoric acid (H3PO4), potassium phosphate monobasic (KH2PO4), polyethylene glycol tert-octylphenyl ether (Triton X-100; 10% solution), D,L-dithiothreitol, tris(hydroxymethyl)aminomethane hydrochloride (TRIS), sodium lactate and all flavonoids were purchased from Sigma-Aldrich (St. Louis, MO, USA). Acetonitrile, methanol and dimethylsulfoxide (DMSO) were purchased from Thermo Fisher Scientific (Fisher Scientific s.r.o., Pardubice, Czech Republic), potassium phosphate dibasic (K2HPO4) from Penta (Penta s.r.o., Prague, Czech Republic), nicotinamide adenine dinucleotide (NAD+) from Toronto Research Chemicals (Toronto, Ontario, Canada), saline from B. Braun (B. Braun Medical s.r.o., Prague, Czech Republic), and heparin from Zentiva (Zentiva, k.s., Prague, Czech Republic). Ultrapure water was obtained using a Millipore Milli-Q system (Merck Millipore, MA, USA).
Stock solutions of cobalt chloride (5 mM) and NNDSA (5 mM) for chelation testing were prepared in ultrapure water. For red blood cell lysis experiments, cobalt chloride was dissolved in saline (5 mM). The flavonoids were dissolved in DMSO for chelation testing and haemolysis experiments. For the HPLC method, cobalt chloride was dissolved in ultrapure water (5 mM) and freshly diluted with ultrapure water to obtain the working solution (40 μM), while flavonoids were dissolved in methanol (5 mM).
For chelation testing, acetate buffers (15 mM of sodium acetate and 27.3 or 2.7 mM of acetic acid) were used for the pH values of 5.5 and 4.5, respectively, whereas HEPES buffers (15 mM of sodium HEPES and 71.7 and 14.3 mM of HEPES) were employed for pH values of 6.8 and 7.5, respectively. For the HPLC method, 5 mM solution of TRIS was used for the preparation of buffers of pH values 6.8 and 7.5, as a HEPES buffer that can act as an antioxidant itself could not be used in this case.45 For erythrocyte haemolysis testing, a phosphate buffer was prepared as a mixture of KH2PO4 and K2HPO4 (2.72 g/100 mL KH2PO4 + 3.48 g/100 mL K2HPO4) at a pH of 7.8, and a 0.1 M solution of TRIS was used to prepare a buffer at a pH of 8.9.
Competitive chelation experiments and red blood cell lysis determination were performed via measuring lactate dehydrogenase activity in 96-well microplates (BRAND GmbH&Co. KG, Wertheim, Germany) using a Hidex Sense Beta Plus Microplate Reader (Hidex, Turku, Finland). Experiments for the stability constant determination and non-competitive cobalt chelation measurements were performed in semimicro polystyrene cuvettes (BrandTech Scientific Inc., Essex, CT, USA), and the absorbance was measured using a Helios Gamma spectrophotometer equipped with the VisionLite 2.2 software (ThermoFisher Scientific Inc., Waltham, MA, USA). For performing the Fenton chemistry analysis, a chromatographic system consisting of an ESA Model 582 Solvent Delivery Module and an ESA coulometric Coulochem III detector was used (both ESA, Chelmsford, MA, USA), equipped with a high-sensitivity analytical cell with two porous graphite working electrodes placed in series in the same cell. An Onyx Monolithic RP C8 column 100 × 4.6 mm (Phenomenex, Torrance, CA, USA) was employed as the stationary phase. The data integration system was the DataApex (Prague, Czech Republic) Chromatography Station software (version CSW 1.7).
2.2 Methodological approach
2.2.1 Competitive spectrophotometric testing of cobalt chelation. The degree of cobalt chelation was determined by a spectrophotometric method with the indicator NNDSA, as much recently reported by us.44 The principle of this method is that if a flavonoid is able to chelate cobalt, the amount of free cobalt ions in the solution decreases and these free, i.e., non-chelated, cobalt ions bind to the indicator NNDSA. Then, this complex of NNDSA-Co was measured spectrophotometrically. Briefly, 100 μL of various buffers were first mixed with 50 μL DMSO, the solvent of flavonoids (for better solubility), and then 50 μL DMSO or the same volume of tested flavonoids dissolved in DMSO were added. A solution of cobalt ions (50 μL, 300 μM) or ultrapure water in the case of blank was added, and the mixture was stirred for 2 min at room temperature. Afterwards, ultrapure water or an aqueous solution of 1.8 mM NNDSA was added, and the absorbance was measured at 0 and 5 minutes at 490 and 540 nm.
2.2.2 Non-competitive spectrophotometric assessment of cobalt chelation. Absorption spectra of baicalein and 3-hydroxyflavone with/without cobalt and with the indicator NNDSA were recorded from 300 to 600 nm in 0.5 nm increments. The experiments were carried out at the same concentrations and ratios as discussed in the previous Section 2.2.1. Shortly, 300 μL of various buffers were first mixed with 150 μL DMSO, and then 150 μL DMSO or the same volume of tested flavonoids dissolved in DMSO (1.5 mM) were added. A solution of cobalt ions (150 μL, 300 μM) or ultrapure water in the case of blank was added, and the mixture was stirred at room temperature for 2 min. Afterwards, 150 μL of ultrapure water or an aqueous solution of 1.8 mM NNDSA was added and spectra were recorded.
2.2.4 HPLC monitoring of the hydroxyl radical generation from the cobalt-triggered Fenton reaction. High-performance liquid chromatography with electrochemical (coulometric) detection was used to evaluate the effect of the selected chelators on the cobalt-triggered Fenton reaction. The degree of hydroxyl radical production was determined by comparing the generation of hydroxylation products in the Fenton reaction mixtures with and without tested flavonoids. The basis of this method is the detection and quantitation of three products of salicylate hydroxylation mediated by hydroxyl radicals (˙OH): catechol, 2,3-DHBA and 2,5-DHBA. The complete methodology has been previously published.44 Two pathologically/physiologically relevant pH values (pH 6.8 and 7.5) were assessed. Shortly, 700 μL of TRIS buffer (pH 6.8 and 7.5) was mixed with 200 μL of methanol (blank), or different concentrations of a tested compound. Then, 50 μL of a 40 μM Co2+ solution was added, so that the molar concentration ratios ranged from 1
:
200 to 100
:
1 (flavonoid
:
cobalt). After vortexing, 45 μL of salicylic acid (the substrate, 66.67 mM) was added. In the last step, the Fenton reaction was launched by adding 5 μL of 30% H2O2. The reaction was run at room temperature for 2 minutes. Afterwards, the analysis was performed using the following parameters: in the analytical cell, potential 1 was set to −200 mV and potential 2 to +450 mV, sensitivity 200 nA. The mobile phase was composed of 5 mM H3PO4 buffer with 1 mM EDTA (pH of this mixture adjusted to 2.85) and acetonitrile (93/7, v/v). An HPLC analysis was performed in the isocratic mode at a flow rate of 1.0 mL min−1 and an injection volume of 20 μL was used.
2.2.5 Erythrocyte haemolysis testing. This experiment was performed according to previous studies47 with some modifications.44 Blood was obtained from adult rats (Wistar Han, Velaz, s.r.o., Czech Republic) by exsanguination into heparinized tubes. The exsanguination was performed by a trained researcher in accordance with The Guide for the Care and Use of Laboratory Animals published by the American National Institutes of Health (8th edition, revised 2011, ISBN-13: 978-0-309-15400-0). Blood was collected from the rat aorta into heparinized test tubes as a by-product of other experiments (approved by the Czech Ministry of Health No. MSMT-34121_2017-2). The blood was then centrifuged at 2770 g and the plasma was removed. The erythrocyte fraction was washed by adding a saline solution and centrifuged again. Additional heparin at a final concentration of 10 IU mL−1 was added, and the mixture was diluted 10-fold with a 1 mM glucose solution in saline. The average erythrocyte concentration obtained using a TC20 Automated Cell Counter (Bio-Rad, UK) was 1.8 ± 0.3 × 1010 per millilitre.First, 940 μL of this suspension was slowly and continuously mixed with 10 μL of tested flavonoid at various concentrations dissolved in DMSO, and with 50 μL of 5 mM CoCl2 solution dissolved in saline at 37 °C for 4 hours. After incubation, the samples were centrifuged at 1950 g for 10 minutes, and 250 μL of the supernatant was used to determine the lactate dehydrogenase (LDH) activity, a marker associated with possible erythrocyte lysis. The remaining liquid was removed and a lysis buffer (a phosphate buffer pH 7.8 with 1% Triton X-100, 2 mM EDTA and 1 mM D,L-dithiothreitol) was added to the sediment in the same volume as the removed supernatant. After 20 minutes of incubation at room temperature, the samples were centrifuged again for 10 minutes and processed similarly to the supernatant. This was considered a marker of the remaining erythrocytes that did not undergo lysis during the 4 hour incubation. The LDH activity was determined kinetically using a reagent solution (a mixture of NAD+, 0.1 M TRIS buffer at pH 8.9 and a 40 mM lactate solution) in 96-well microplates (Hidex Sense Beta Plus Microplate reader). The results were calculated as the percentage of lysed erythrocytes and compared to a positive control, where the solvent (DMSO) was used instead of the flavonoid. Saline was added to the negative control instead of cobalt; it was otherwise processed just like the other samples.
2.3 Mathematical and statistical analysis
The results are presented as mean ± SD. As a rule, three independent fresh solutions with at least two technical duplicates were tested.
2.3.1 Spectrophotometric determination of cobalt chelators. The amount of non-chelated cobalt was calculated from the difference in absorbance between the tested (with the indicator) and blank samples (without the indicator) divided by the difference in absorbance of the control sample (the known amount of cobalt without the tested substance) and its blank (i.e., cobalt without the indicator, from which the differences between the absorbance of negative samples with and without the indicator were subtracted).
2.3.2 HPLC. The percentage of decrease (an antioxidant effect) or increase (a pro-oxidant effect) in hydroxyl radical production was calculated from the sum of concentrations of catechol, 2,3-DHBA and 2,5-DHBA between the tested sample and the positive blank, which contained only solvent instead of the tested compound.
2.3.3 Erythrocyte haemolysis. The total percentage of erythrocyte lysis was determined from the reaction slopes (k) obtained by measuring the LDH activity according to the formula:
, where BL is the sample “before” lysis (diluted 1
:
1) and AL is the sample “after” lysis (diluted 1
:
5). Subsequently, the lysis in those samples containing the tested flavonoids was compared with the corresponding positive or negative blanks (i.e., either a solution of Co2+ in saline or just saline) according to the formula:
. This determined whether the tested substance affected erythrocyte lysis or not.
2.3.4 Statistical analysis. A statistical comparison was performed using the ANOVA test followed by the Bonferroni post-hoc test or the Student's T-test using GraphPad Prism version 9.0 for Windows (GraphPad Software, USA).
3 Results
3.1 Screening of cobalt chelation
In the first step, the chelation activity of flavonoids (for structures see ESI Fig. 1†) was assessed in a 5-time excess of flavonoid over cobalt and compared with the negative (blank, the solvent DMSO) and positive controls (known chelator nitroxoline). Out of the 23 flavonoids tested, six were able to significantly chelate cobalt ions at a neutral pH, while 13 compounds were at least partly active at pH 6.8 (Fig. 1). At pH 5.5, flavonoids were not active with the exception of baicalein and 7,8-dihydroxyflavone, whereas none of them was active at pH 4.5 (data not shown). None of the flavonoids was more active than the standard chelator nitroxoline. The most potent compounds, baicalein and 3-hydroxyflavone, were further tested for the construction of concentration-dependent curves at a pH ranging from 7.5 to 5.5. Baicalein chelated cobalt ions with a similar potency at pH 5.5–7.5 (Fig. 2A). Regardless, it was never able to achieve complete cobalt chelation. Contrarily, 3-hydroxyflavone achieved complete chelation of cobalt ions at pH 7.5, but these complexes were not stable in time (Fig. 2B) in contrast to its complexes at pH 6.8 or complexes of cobalt with baicalein (Figs. 2C and 3). Quercetin is shown for comparison as it is the most investigated flavonoid. It was inactive even at a high ratio (33
:
1, Fig. 2D). As flavonoids can absorb at a wavelength around 490 nm, data were also analysed at 540 nm. Both wavelengths were selected based on our previous study.44 The results were essentially similar to few small differences (ESI Fig. 2†).
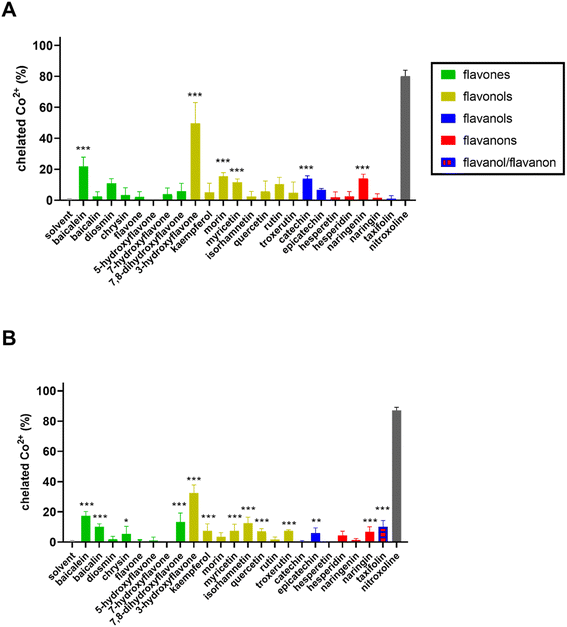 |
| Fig. 1 Chelation ability of the tested flavonoids at pH 7.5 (A) and at pH 6.8 (B). The proportion of tested flavonoids : cobalt was 5 : 1. Data were analysed from the measurements taken at a wavelength of 490 nm. Nitroxoline was used as a positive control and the solvent DMSO as a negative control. All compounds were less active than nitroxoline under both conditions (p < 0.001). Only compounds with a symbol of a star/stars (*p < 0.05 vs. solvent, **p < 0.01 vs. solvent, ***p < 0.001 vs. solvent) were active according to the statistical comparison with the solvent itself. | |
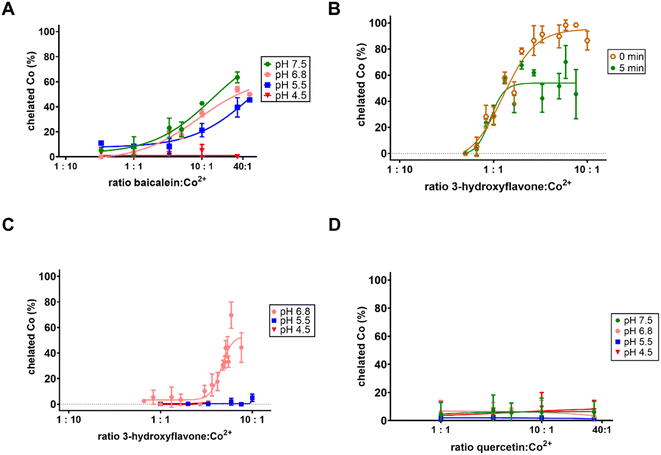 |
| Fig. 2 Cobalt chelation ability of the two most active flavonoids and quercetin: (A) baicalein; (B and C): 3-hydroxyflavone ((B) pH 7.5; (C) pH 4.5, 5.5 and 6.8); D: quercetin. The degree of chelation was calculated according to the absorbance at 490 nm after 5 minutes with the exception of 3-hydroxyflavone, pH 7.5, where the chelation was calculated both immediately (0 min) and after 5 minutes to demonstrate the instability of complex. | |
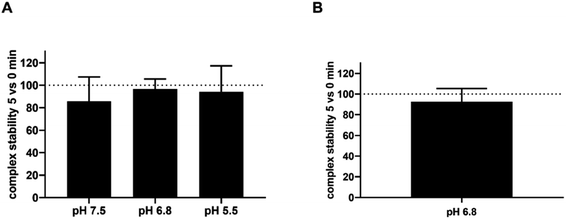 |
| Fig. 3 Stability of complex flavonoid–cobalt in time: (A) baicalein; (B) 3-hydroxyflavone. Stability was calculated from the measurement at 490 nm as the percentage of chelation after 5 minutes vs. immediate measurement. Data are shown only for pH conditions where efficient chelation was achieved with the exception of pH 7.5 and 3-hydroxyflavone, where the complexes were unstable (see Fig. 2B). | |
To further confirm the activity of the two most active flavonoids, baicalein and 3-hydroxyflavone, their spectra with/without cobalt and with the indicator NNDSA were measured (ESI Fig. 3†), and the stability constants (Table 1) were calculated. Based on the spectra of flavonoids and their complexes with cobalt, no interference with the NNDSA competitive method was noticed, as there was no absorption at 490 or 540 nm. A clear modification of the flavonoid spectra after addition of cobalt was noted solely at pH 7.5. In case of 3-hydroxyflavone, a new peak appeared at 413 ± 0 nm after addition of cobalt ions, whereas the peak at 346 ± 0 nm remained. In the case of baicalein, the first peak was not significantly modified (363 ± 1 nm vs. 364 ± 0) again. In contrast to 3-hydroxyflavone, a clear second peak was not detected but a shoulder appeared in the area of 420–480 nm. Interestingly, under lower pH conditions, addition of cobalt ions did not result in a clear modification of the spectra and the chelation can be confirmed only with the modification of spectra with NNDSA in time in the case of baicalein.
Table 1 Stability constants of 3-hydroxyflavone-cobalt and baicalein-cobalt complexes at pH 7.5
Flavonoid |
Complex |
Stability constant log K |
3-Hydroxyflavone |
1 : 1 |
4.04 ± 0.14 |
3 : 1 |
12.57 ± 0.99 |
Baicalein |
1 : 1 |
5.40 ± 0.16 |
3 : 1 |
15.25 ± 0.79 |
3.2 Hydroxyl radical generation via the cobalt-triggered Fenton reaction
Since free cobalt ions are able to catalyse the Fenton reaction like iron or copper, it was crucial to determine if the chelators could modify the production of hydroxyl radicals. The most active flavonoids as well as quercetin were selected for this testing under neutral or slightly acidic conditions, where both active flavonoids were able to chelate cobalt ions. Baicalein and 3-hydroxyflavone slightly decreased the production of hydroxyl radicals at low ratios of flavonoid/cobalt ions under both the pH conditions tested but showed neutral effects at higher ratios (Fig. 4A and B). Quercetin acted rather as a weak antioxidant at low ratios of flavonoid
:
cobalt under both the pH conditions tested, but this was significant only at a ratio of 1
:
20 at pH 7.5. However, it started to enhance profoundly the production of hydroxyl radicals from 10
:
1 proportion of flavonoid
:
cobalt at pH 6.8, while it had neutral effects at pH 7.5 (Fig. 4C).
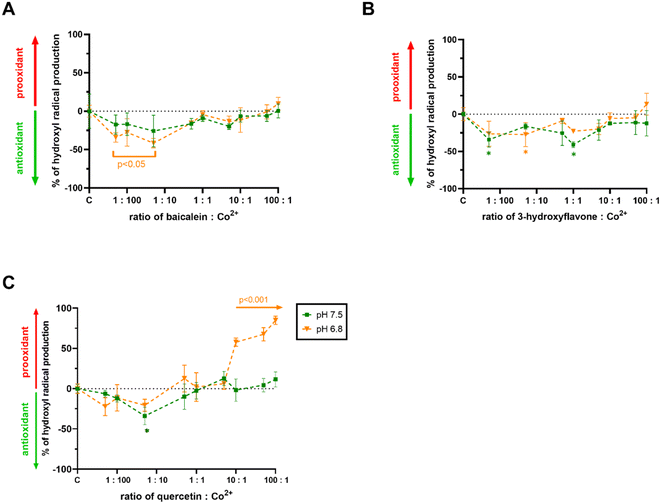 |
| Fig. 4 Effect of flavonoids on the cobalt-triggered Fenton reaction: (A) baicalein; (B) 3-hydroxyflavone; (C) quercetin. The final concentration of cobalt ions was 2 μM. *p < 0.05 vs. a negative control (methanol). | |
3.3. Erythrocyte haemolysis testing
In the last step, all three compounds alone and in the presence of cobalt were tested under biologically relevant conditions with purified rat red blood cells. Cobalt itself is mostly non-toxic to erythrocytes when present in the micromolar range of concentrations.44 Flavonoids alone caused significant cell toxicity solely at very high concentrations (baicalein from 750 μM, 3-hydroxyflavone and quercetin from a concentration of 1 mM, Fig. 5).
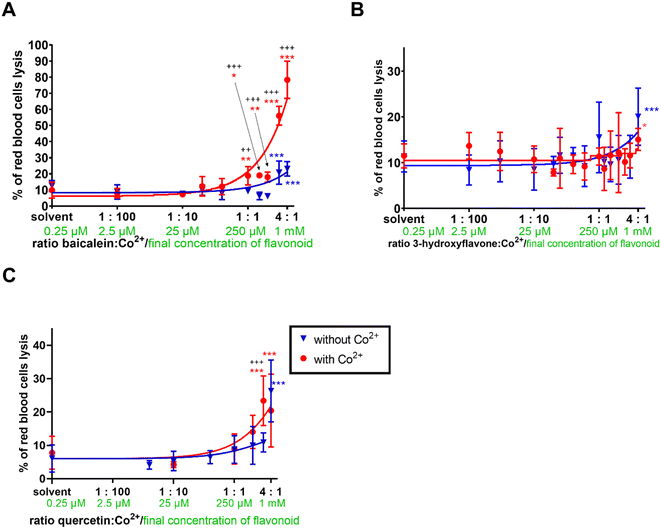 |
| Fig. 5 Effect of the selected flavonoids on rat erythrocytes lysis: (A) baicalein; (B) 3-hydroxyflavone; (C) quercetin: the graphs show the lysis of erythrocytes caused by the tested flavonoids alone and in the presence of cobalt ions. The final concentration of cobalt ions was 250 μM. *p < 0.05, **p < 0.01, ***p < 0.001: blue symbols indicate comparison vs. the blank without cobalt, red symbols indicate comparison vs. the blank with cobalt ions, and black color indicates the corresponding samples with and without cobalt ions. | |
In contrast to 3-hydroxyflavone, quercetin and baicalein when given together with cobalt, increased the red blood cell lysis. The effect of quercetin was relatively mildly enhanced and became significant only at a proportion of 3
:
1, while cobalt profoundly increased the toxicity of baicalein from 1
:
1 proportion (Fig. 5A–C).
4. Discussion
Literature affords some indications that flavonoids can directly interact with cobalt. These data come mostly from cellular experiments and few very recent animal studies. The available rat studies reported uniform protection of flavonoids against cobalt toxicity.34–38 These data are interesting but cannot offer additional explanation in this area, as all tested flavonoids (luteolin, quercetin, naringenin and taxifolin) have low bioavailability in the parent form40 and, hence, could have interacted with cobalt only at the gastrointestinal level or the observed effect was mediated by their metabolites.
Cellular studies are of greater interest in relation to our investigation. Quercetin, rutin, epigallocatechin gallate, genistein and naringin protected different cell lines against toxicity following incubation with excessive cobalt.29–32,48 Similarly, cobalt-induced intracellular oxidative stress was decreased or even fully abolished by co-incubation with isorhamnetin, fisetin, and epigallocatechin gallate.30,33,49 The protective effect against cobalt toxicity might be, however, mediated by a different mechanism(s) than cobalt chelation. For example, apigenin and genistein blocked both cobalt and hypoxia-induced HIF-1α expression.50,51 Moreover, this effect is not a class effect of flavonoids, as flavonols myricetin and kaempferol were active while flavanols epicatechin and catechin were not.51 Regardless, the protective effect of flavonoids seems to be associated with the lowering of ROS and is attenuated in the absence of HIF-1α.33
However, it should also be mentioned that Crispo et al. have not found protection against cobalt toxicity in rat pheochromocytoma-derived PC12 cells in a large series of flavonoids (epigallocatechin gallate, fisetin, kaempferol, morin, myricetin and quercetin).52 This finding is at the moment hardly to conciliate with current knowledge as epigallocatechin and fisetin protected the same cell line in other studies.30,33 The only obvious difference is the use of lower concentration of CoCl2 in the latter studies. Interestingly, there is also one study which reported that cobalt reduced the cellular toxicity of green and black tea polyphenols.53
In order to investigate the impact of flavonoids more deeply, we selected red blood cells, which is a model not burdened by the aforementioned transcriptional cascades. Very surprisingly, both cobalt chelating flavonoid baicalein and inactive cobalt chelator quercetin enhanced their toxicity in the presence of cobalt ions (Fig. 5). This was an unexpected finding at least for baicalein, which in our assay of the cobalt-triggered Fenton reaction behaved as an antioxidant. Contrarily, quercetin promoted both the Fenton reaction (Fig. 4C) and red blood cell lysis (Fig. 5C). As far as we know, these are the first data on the effect of flavonoids on the cobalt-driven Fenton reaction. There is only one report showing contrarily quercetin to block the cobalt-driven Fenton reaction. This effect was observed merely at a pH of 9.0, which is a non-physiological condition, and it is well known that chelation effects of flavonoids increase with the pH.54–56 This is probably the explanation for this phenomenon as strong chelation will block the participation of cobalt in the Fenton reaction.
Direct proofs on cobalt chelation by flavonoids are very limited. The UV-Vis spectrum of flavonols quercetin and its glycoside rutin are changed when cobalt is added.27,29,57 Similarly, quercetin complexes were confirmed by electrospray ionization tandem mass spectrometry.28 Contrarily, isoflavonoid genistein containing solely one possible chelation site, 5-hydroxy-4-ketogroup, was not found to complex cobalt.29 Our data seem to agree in general with such finding as different flavones possessing only this chelation site were not able to substantially chelate cobalt either at pH 6.8 or at pH 7.5. We must admit that some mild chelation was observed with some flavanones as naringenin, but the extent of chelation was low. Flavanones in contrast to flavones do not contain 2,3-double bonds. Interestingly, one study reported that flavonoids chelated cobalt through the discussed 5-hydroxyl and the 4-carbonyl moiety.28 This is however very unlikely based on our current data and also previously published data with iron and copper, where this site was observed as less active after 3-hydroxy-4-keto site or adjacent hydroxyl groups in ring A.54,55 Indeed, the most active compound from this study is 3-hydroxyflavone, which has solely one chelation position composed of the 3-hydroxyl and 4-ketogroup, and baicalein, possessing the 5,6,7-trihydroxygroup in ring A. Moreover, the 7,8-dihydroxygroup was associated with some degree of chelation, but this was markedly less pronounced as in the previous case. The summary of the observed findings is shown in Fig. 6.
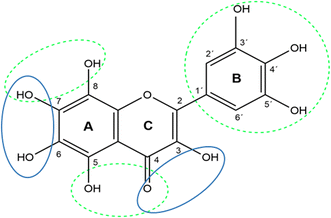 |
| Fig. 6 Possible cobalt chelating sites of flavonoids: major chelation sites are shown in blue ellipses and minor sites in green dashed ellipses and circle. | |
An interesting finding is the difference in cobalt chelation between stereoisomers, catechin and epicatechin. There is currently no explanation for this phenomenon, but the finding is not isolated as differences in metal reduction as well as zinc chelation have been previously observed between this couple.41,58
Very little is known about the stoichiometry of the cobalt–flavonoid complexes. In the case of quercetin, three stoichiometries (1
:
1, 1
:
2 and 2
:
3, metal
:
flavonoid) were suggested by electrospray ionization tandem mass spectrometry,28 whereas 1
:
1 by potentiometry/UV-Vis spectrometry.27 The latter was observed also for rutin.27 Our measurement did not bring very much light in this issue as most flavonoids were inactive or relatively low active cobalt chelators. The strongest activity enabling the assessment of stoichiometry of the forming complex was observed for 3-hydroxyflavone, but solely at pH 7.5. At a proportion of 1
:
1, it chelated cobalt ions from about 33% (Fig. 2B), and this might lead to a conclusion that a complex 1
:
3, Co
:
3-hydroxyflavone was formed. This is however burdened by the fact that these complexes were unstable.
The major outcome of this study in relation to our hypothesis was rather disappointing, as flavonoids were found to be weak or even ineffective cobalt chelators with two exceptions of baicalein and 3-hydroxyflavone. The former compound was not found to be a suitable cobalt chelator as it potentiated red blood cell lysis. Only 3-hydroxyflavone appears to be a safe compound, but its chelation activity and its consequences measured by the inhibition of the Fenton reaction were limited, so its use as a cobalt chelator is improbable. Regardless, it could not be excluded that its chemical derivation cannot improve its chelation properties. Based on our data, hydroxylation of ring B is not advantageous in this case, as it resulted in a marked drop or even loss of activity (vs. kaempferol, morin and quercetin (Fig. 1)). Moreover, this modification can promote toxicity as observed with quercetin.
Quercetin together with baicalein behaved as pro-oxidants toward red blood cells. This effect is considered negative but might open a reflection if such effect can be targeted toward cancer cell lines. As cobalt is stabilizing HIF-1α with subsequent effects on gene transcription and cellular apoptosis and quercetin has been recently shown to increase the transfer of cobalt into Caco-2 cells,29 this idea might gain some scientific interest in cancer.
5. Conclusion
Only two out of 23 flavonoids demonstrated moderate cobalt chelating activity. These compounds possess either the 3-hydroxy-4-keto chelation site (3-hydroxyflavone) or the 5,6,7-trihydroxygroup (baicalein). The former compound was a stronger chelator; however, its chelation potency dropped with pH. Contrarily, the latter had a more stable chelation effect under (patho)physiologically relevant pH conditions ranging from 7.5 to 5.5. Both compounds only mildly decreased the hydroxyl radical formation via the cobalt-triggered Fenton chemistry. Moreover, the toxicity of baicalein toward red blood cells was even potentiated by cobalt. The same but less expressed behaviour was also observed for non-chelating quercetin. The use of natural flavonoids as cobalt chelators is improbable, but 3-hydroxyflavone can serve as a prototype for the development of more potent cobalt chelators.
List of abbreviations
2,3-DHBA | 2,3-Dihydroxybenzoic acid |
2,5-DHBA | 2,5-Dihydroxybenzoic acid |
DMSO | Dimethyl sulfoxide |
EDTA | Ethylenediaminetetraacetic acid or its disodium salt |
HEPES | 4-(2-Hydroxyethyl)-1-piperazineethanesulfonic acid |
LDH | Lactate dehydrogenase |
NAD+ | Nicotinamide adenine dinucleotide |
NNDSA | 1-Nitroso-2-naphthol-3,6-disulfonic acid or its disodium salt |
˙OH | Hydroxyl radical |
ROS | Reactive oxygen species |
Author contributions
M. M.: investigation; data curation; writing—original draft; writing—review and editing; Z. L.: investigation; data curation; writing—original draft; writing—review and editing; R. K.: methodology; supervision; writing—review and editing; P. M.: conceptualization; data curation; funding acquisition; methodology; supervision; writing—original draft; writing—review and editing.
Conflicts of interest
None of the authors have a conflict of interest to disclose.
Acknowledgements
The authors also acknowledge the support of EFSA-CDN project (CZ.02.1.01/0.0/0.0/16_019/0000841) co-funded by the ERDF. M. M. thanks also to the Charles University (SVV 260 549).
References
- D. J. Paustenbach, B. E. Tvermoes, K. M. Unice, B. L. Finley and B. D. Kerger, Crit. Rev. Toxicol., 2013, 43, 316–362 CrossRef CAS PubMed.
- L. Leyssens, B. Vinck, C. Van Der Straeten, F. Wuyts and L. Maes, Toxicology, 2017, 387, 43–56 CrossRef CAS PubMed.
- K. A. Fox, T. M. Phillips, J. H. Yanta and M. G. Abesamis, Clin. Toxicol. (Phila), 2016, 54, 874–877 CrossRef CAS PubMed.
- M. Kovochich, A. Monnot, D. G. Kougias, S. L. More, J. T. Wilsey, Q. Q. Qiu, L. E. L. Perkins, P. Hasgall, M. Taneja, E. E. Reverdy, J. Sague, S. Marcello, K. Connor, J. Scutti, W. V. Christian, P. Coplan, L. B. Katz, M. Vreeke, M. Calistri-Yeh, B. Faiola, K. Unice and G. Eichenbaum, Regul. Toxicol. Pharmacol., 2021, 122, 104910 CrossRef CAS PubMed.
- M. Packer, Circ.: Heart Failure, 2016, 9, e003604 Search PubMed.
- D. Pelclova, M. Sklensky, P. Janicek and K. Lach, Clin. Toxicol. (Phila), 2012, 50, 262–265 CrossRef CAS PubMed.
- J. J. Devlin, A. C. Pomerleau, J. Brent, B. W. Morgan, S. Deitchman and M. Schwartz, J. Med. Toxicol., 2013, 9, 405–415 CrossRef CAS PubMed.
- A. D. Monnot, M. Kovochich, S. B. Bandara, J. T. Wilsey, W. V. Christian, G. Eichenbaum, L. E. Perkins, P. Hasgall, M. Taneja and K. Connor, Regul. Toxicol. Pharmacol., 2021, 123, 104932 CrossRef CAS PubMed.
- B. Sánchez-Dalmau, F. Spencer, L. Sánchez-Vela, A. Camós-Carreras, S. N. Xarau and J. Fernández-Valencia, Arch. Soc. Esp. Oftalmol. (Engl. Ed.), 2021, 96, 316–320 CrossRef.
- M. I. Sanz Pérez, A. M. Rico Villoras, A. Moreno Velasco, S. Bartolomé García and J. Campo Loarte, Hip Int., 2019, 29, NP1–NP5 CrossRef PubMed.
- M. Umar, N. Jahangir, M. F. Khan, Z. Saeed, F. Sultan and A. Sultan, J. Orthop. Surg. (Hong Kong), 2020, 28 Search PubMed.
- F. Zheng, Y. Li, F. Zhang, Y. Sun, C. Zheng, Z. Luo, Y.-L. Wang, M. Aschner, H. Zheng and L. Lin, J. Hazard. Mater., 2021, 419 CrossRef CAS PubMed.
- S. E. Green, M. W. Luczak, J. L. Morse, Z. DeLoughery and A. Zhitkovich, Toxicol. Sci., 2013, 136, 467–477 CrossRef CAS PubMed.
- K. S. Kasprzak, Free Radical Biol. Med., 2002, 32, 958–967 CrossRef CAS PubMed.
- A. Biglia, V. Morandi, S. Monti, P. Delvino, L. Cavagna and C. Montecucco, Jt., Bone, Spine, 2020, 87, 652–654 CrossRef CAS PubMed.
- X. Du, J. Liu, Y. Wang, M. Jin and Q. Ye, Toxicol. Ind. Health, 2021, 37, 280–288 CrossRef CAS PubMed.
- B. E. Tvermoes, B. L. Finley, K. M. Unice, J. M. Otani, D. J. Paustenbach and D. A. Galbraith, Food Chem. Toxicol., 2013, 53, 432–439 CrossRef CAS PubMed.
- H. I. Choi, J. A. Hong, M. S. Kim, S. E. Lee, S. H. Jung, P. W. Yoon, J. S. Song and J. J. Kim, Cardiovasc. Toxicol., 2019, 19, 82–89 CrossRef CAS PubMed.
- U. E. Pazzaglia, P. Apostoli, T. Congiu, S. Catalani, M. Marchese and G. Zarattini, Arch. Orthop. Trauma Surg., 2011, 131, 1299–1308 CrossRef CAS PubMed.
- M. M. Kasprzak, A. Erxleben and J. Ochocki, RSC Adv., 2015, 5, 45853–45877 RSC.
- J. A. Ross and C. M. Kasum, Annu. Rev. Nutr., 2002, 22, 19–34 CrossRef CAS PubMed.
- F. Fusi, A. Trezza, M. Tramaglino, G. Sgaragli, S. Saponara and O. Spiga, Pharmacol. Res., 2020, 152, 104625 CrossRef CAS PubMed.
- P. Knekt, J. Kumpulainen, R. Järvinen, H. Rissanen, M. Heliövaara, A. Reunanen, T. Hakulinen and A. Aromaa, Am. J. Clin. Nutr., 2002, 76, 560–568 CrossRef CAS PubMed.
- M. Olszowy, Plant Biochem. J., 2019, 144, 135–143 CrossRef CAS PubMed.
- D. Procházková, I. Boušová and N. Wilhelmová, Fitoterapia, 2011, 82, 513–523 CrossRef PubMed.
- L. Wen, Y. Zhao, Y. Jiang, L. Yu, X. Zeng, J. Yang, M. Tian, H. Liu and B. Yang, Free Radical Biol. Med., 2017, 110, 92–101 Search PubMed.
- G. M. Escandar and L. F. Sala, Can. J. Chem., 1991, 69, 1994–2001 CrossRef CAS.
- Y. Liu and M. Guo, Molecules, 2015, 20, 8583–8594 CrossRef CAS PubMed.
- V. B. Vergara and J. F. Kalinich, Nutrients, 2021, 13, 2545 CrossRef CAS PubMed.
- J. Y. Jung, H. C. Mo, K. H. Yang, Y. J. Jeong, H. G. Yoo, N. K. Choi, W. M. Oh, H. K. Oh, S. H. Kim, J. H. Lee, H. J. Kim and W. J. Kim, Life Sci., 2007, 80, 1355–1363 CrossRef CAS PubMed.
- S. Li, J. Jiang, J. Fang, X. Li, C. Huang, W. Liang and K. Wu, Int. J. Mol. Med., 2021, 47, 1–11 Search PubMed.
- Y. N. Shi, X. Q. Zhang, Z. Y. Hu, C. J. Zhang, D. F. Liao, H. L. Huang and L. Qin, Pharmacology, 2019, 103, 282–290 CrossRef CAS PubMed.
- P. Y. Chen, Y. R. Ho, M. J. Wu, S. P. Huang, P. K. Chen, M. H. Tai, C. T. Ho and J. H. Yen, Food Funct., 2015, 6, 287–296 Search PubMed.
- T. O. Ajibade, A. A. Oyagbemi, T. O. Omobowale, E. R. Asenuga and K. O. Adigun, Biol. Trace Elem. Res., 2017, 175, 347–359 CrossRef CAS PubMed.
- A. S. Akinrinde and O. E. Adebiyi, Neurotoxicology, 2019, 74, 252–263 CrossRef CAS PubMed.
- A. A. Oyagbemi, A. S. Akinrinde, O. E. Adebiyi, T. A. Jarikre, T. O. Omobowale, O. E. Ola-Davies, A. B. Saba, B. O. Emikpe and A. A. Adedapo, Environ. Toxicol. Pharmacol., 2020, 80, 103488 CrossRef CAS PubMed.
- A. T. Salami, A. A. Oyagbemi, M. V. Alabi and S. B. Olaleye, Biomarkers, 2022, 1–13, DOI:10.1080/1354750x.2022.2137235.
- C. Tanoğlu, A. Ersoy, T. A. Çoban, G. N. Yazıcı, R. Mammadov and B. Süleyman, Acta Neurobiol. Exp. (Wars), 2022, 82, 254–262 Search PubMed.
- M. Kozsup, X. Zhou, E. Farkas, A. C. Bényei, S. Bonnet, T. Patonay, K. Kónya and P. Buglyó, J. Inorg. Biochem., 2021, 217, 111382 CrossRef CAS PubMed.
- A. Crozier, D. Del Rio and M. N. Clifford, Mol. Aspects Med., 2010, 31, 446–467 CrossRef CAS PubMed.
- Z. Lomozová, M. Hrubša, P. F. Conte, E. Papastefanaki, M. Moravcova, M. C. Catapano, I. P. Silvestri, J. Karlíčková, R. Kučera and K. Macáková, Food Chem., 2022, 394, 133461 CrossRef PubMed.
- K. Macáková, P. Mladěnka, T. Filipský, M. Říha, L. Jahodář, F. Trejtnar, P. Bovicelli, I. Proietti Silvestri, R. Hrdina and L. Saso, Food Chem., 2012, 135, 2584–2592 CrossRef PubMed.
- I. Parejo, C. Codina, C. Petrakis and P. Kefalas, J. Pharmacol. Toxicol. Methods, 2000, 44, 507–512 CrossRef CAS PubMed.
- M. Moravcová, M. Hrubša, Z. Lomozová, M. C. Catapano, R. Argento, E. Jirkovský, R. Kučera, L. Mercolini and P. Mladěnka, Med. Chem., 2023, 19, 495–507 CrossRef.
- H. Li, Z. Lu, G. Cheng, K. Rong, F. Chen and R. Chen, RSC Adv., 2015, 5, 5059–5067 RSC.
- Z. Lomozová, M. C. Catapano, M. Hrubša, J. Karlíčková, K. Macáková, R. Kučera and P. Mladěnka, J. Agric. Food Chem., 2021, 69, 5926–5937 CrossRef PubMed.
- P. Mladenka, J. Karlickova, M. Hrubsa, E. Veljovic, S. Muratovic, A. Carazo, A. S. Mali, S. Spirtovic-Halilovic, L. Saso, M. Pour and K. Duric, Appl. Sci., 2020, 10, 4846 CrossRef CAS.
- R. L. Sundaram, V. K. Sali and H. R. Vasanthi, Phytomedicine, 2018, 51, 196–204 CrossRef PubMed.
- S. Seo, K. Seo, S. H. Ki and S. M. Shin, Biol. Pharm. Bull., 2016, 39, 1830–1838 CrossRef CAS PubMed.
- B. Wang, Y. Zou, Z. L. Yuan and J. G. Xiao, J. Ocul. Pharmacol. Ther., 2003, 19, 457–464 CrossRef CAS PubMed.
- M. Osada, S. Imaoka and Y. Funae, FEBS Lett., 2004, 575, 59–63 CrossRef CAS PubMed.
- J. A. Crispo, D. R. Ansell, M. Piche, J. K. Eibl, N. Khaper, G. M. Ross and T. C. Tai, Can. J. Physiol. Pharmacol., 2010, 88, 429–438 CrossRef CAS PubMed.
- H. Babich, T. Gold and R. Gold, Toxicol. Lett., 2005, 155, 195–205 CrossRef CAS PubMed.
- P. Mladěnka, K. Macáková, T. Filipský, L. Zatloukalová, L. Jahodář, P. Bovicelli, I. P. Silvestri, R. Hrdina and L. Saso, J. Inorg. Biochem., 2011, 105, 693–701 CrossRef PubMed.
- M. Říha, J. Karlíčková, T. Filipský, K. Macáková, L. Rocha, P. Bovicelli, I. P. Silvestri, L. Saso, L. Jahodář and R. Hrdina, RSC Adv., 2014, 4, 32628–32638 RSC.
- L. Chirug, E. Eran Nagar, Z. Okun and A. Shpigelman, Food Hydrocolloids, 2021, 116, 106654 CrossRef CAS.
- W. M. B. da Silva, S. de Oliveira Pinheiro, D. R. Alves, J. E. S. A. de Menezes, F. E. A. Magalhães, F. C. O. Silva, J. Silva, E. S. Marinho and S. M. de Morais, Neurotoxic. Res., 2020, 37, 893–903 CrossRef CAS PubMed.
- M. Hrubša, V. Tvrdý, M. Kutý, K. Valentová, V. Křen and P. Mladěnka, J. Agric. Food Chem., 2022, 70, 6134–6144 CrossRef PubMed.
|
This journal is © The Royal Society of Chemistry 2023 |