DOI:
10.1039/D3RA02621C
(Paper)
RSC Adv., 2023,
13, 20709-20722
Association behavior and physico-chemical parameters of a cetylpyridinium bromide and levofloxacin hemihydrate mixture in aqueous and additive media
Received
20th April 2023
, Accepted 8th June 2023
First published on 11th July 2023
Abstract
The investigation of the micellization of a mixture of cetylpyridinium bromide (CPB) and levofloxacin hemihydrate (LFH) was carried out by a conductivity technique in aqueous and aq. additive mixtures, including NaCl, NaOAc, NaBenz, 4-ABA, and urea. The aggregation behavior of the CPB + LFH mixture was studied considering the variation in additive contents and the change in experimental temperature. The micelle formation of the CPB + LFH mixture was examined from the breakpoint observed in the specific conductivity versus surfactant concentration plots. Different micellar characteristics, such as the critical micelle concentration (CMC) and the extent of counter ion bound (β), were evaluated for the CPB + LFH mixture. The CMC and β were found to undergo a change with the types of solvents, composition of solvents, and working temperatures. The ΔG0m values of the CPB + LFH system in aqueous and aq. additive solutions were found to be negative, which denotes a spontaneous aggregation phenomenon of the CPB + LFH system. The changes in ΔH0m and ΔS0m for the CPB + LFH mixture were also detected with the alteration in the solvent nature and solution temperature. The ΔH0m and ΔS0m values obtained for the association of the CPB + LFH mixture reveal that the characteristic interaction forces may possibly be ion–dipole, dipole–dipole, and hydrophobic between CPB and LFH. The thermodynamics of transfer and ΔH0m–ΔS0m compensation variables were also determined. All the parameters computed in the present investigation are illustrated with proper logic.
1. Introduction
In the recent past, researchers have tirelessly engaged in investigating how medicines do interaction with surfactants, as surfactants are most frequently employed in the pharmaceutical industries and other applications.1–7 These species have long been utilized in making drugs soluble in body fluids and pharmaceutical production. It is essential to study the drug-surfactant interactions in order to create novel therapeutic formulations and pharmaceutical delivery methods.8–13 Emulsifiers can alter the interfacial tension, polarization, and viscosity of sparingly soluble compounds and their surrounding environments to improve their solubility. The extensive use of surfactant micelles for industrial, technological, and commercial purposes is largely owing to their capacity to improve the extent of solubility.14–17 Surfactants can form micelles in aquatic media due to their amphiphilic nature. The level of surfactants at which this micellization phenomenon occurs is referred to as the ‘critical micelle concentration’ (CMC).18–25 The structure of surfactant molecules is similar to those of biological membranes. When drugs undergo interaction with surfactant molecules, one may find it amazing to envisage how the mode of interaction takes place with that of biological membranes.26,27
In micelles, a special core–shell structure can develop, leading to the accumulation of drug molecules, thereby causing an improved solubility.28–30 According to Osica et al.31 and Satake et al.,32 the interaction between cationic surfactants and the nucleic acid is crucial in the development of the associate-micellar complex. Electrolytes and hydrotropes (HDTs) are anticipated to have an effect on the formation of surfactant micelles, notably the CMC, as they have been demonstrated to change the solubilizing intensity of watery arrangements.33 In fact, this kind of solute has the power to alter the interaction that helps or hinders the micelle formation.10 When electrolytes/HDTs are added to a watery solution, in most practical instances, substances lose some of their solvent properties. Rarely, a drop in solubility results in actual solid-phase precipitation, which is referred to as “salting-out” in the surfactant literature.34–36 This effect happens mostly due to a decrease in the extent of solvation of polar groups. However, when an electrolyte/HDT expands, the ionic strength of the area around the micelle increases, leading to some screen influence that decreases the electrostatic repulsion amongst the polar head groups, which is referred to as the “salting-in” effect.34–36 Furthermore, the spherical micelles may change into cigar- or rod-shaped micelles in the presence of electrolytes/HDTs.37,38 Rahim et al.39 explored the interaction of TTAB + CFH drugs through conductometric studies. They found that the measured CMC values of TTAB decreased in the presence of electrolytes and the detected CMC values of TTAB increased with a bump of temperature but decreased with the rise in CFH content in an aq. medium.
Levofloxacin hemihydrate (LFH) (Scheme 1), a broad-spectrum fluoroquinolone group antibiotic, is used to treat infections caused by bacteria.40 LFH has the ability to inhibit the growth of bacterial cell wall and hamper bacterial DNA reproduction.41 Cetylpyridinium is a quaternary ammonium-based salt that serves as a surface-active agent. The head group of cetylpyridinium bromide (CPB) (Scheme 2) has quaternary nitrogen in the aromatic ring and is attached to a sixteen-carbon aliphatic chain.42 CPB, a common cationic surfactant, is used extensively in germicides. Numerous intriguing applications of CPB have been discovered recently, including its usage in pharmaceuticals as a drug release vehicle, protein folding, polymerization, enzyme-based research, gene delivery, and transportation of genes.42 Amin et al.43,44 reported the effect of organic solvents/urea/salts on the interaction of tetradecyltrimethylammonium bromide (TTAB) with levofloxacin hemihydrate (LFH) drug mixture. They also computed the energetics of the aggregation process and suggested the possible interaction forces among the participating components. Hoque and co-workers reported the effect of electrolytes and temperature on the micellization of cetyltrimethylammonium bromide (CTAB)/tetradecyltrimethylammonium bromide (TTAB) in the manifestation of levofloxacin hemihydrate (LFH) by means of conductivity and molecular dynamics approaches.45,46 It is recognized that the inclusion of any additives such as organic molecules, medicines, polymers, salts, and hydrotropes can alter the surfactant's typical solution behavior. The formation of micelles in solutions is the primary factor contributing to surfactants' improved performance in various drug-surfactant systems. In the last few decades, the investigation of surfactant micelles has been performed to understand the behavior of micellar activity as drug carriers.47
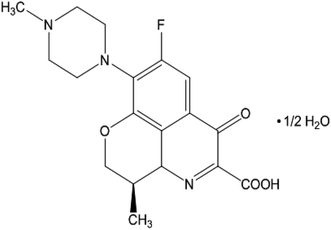 |
| Scheme 1 Levofloxacin hemihydrate (LFH). | |
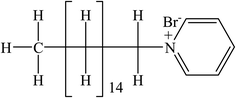 |
| Scheme 2 Cetylpyridinium bromide (CPB). | |
Among the numerous developed drug transporters (drug loading), surfactant micelles have been widely utilized as drug carriers, which possess special features, including a hydrodynamic size (diameter of the micelles) of <50 nm.48–50 The lower micellar size and large-scale production of micelles have stimulated their reception as a first-line drug formulation technology.51 However, the inadequate stability of surfactant micelles in the biological atmosphere restricts their efficiency as potential drug transporters. In many investigations, attempts to enhance the stability of surfactant micelles were made by researchers with the knowledge of reducing the CMC values of surfactants. In order to understand the principles that govern micelle formation, it is useful to obtain different thermodynamic parameters at several temperatures. Micellization thermodynamic parameters in aqueous and non-aqueous environments are more important since they determine the relative importance of hydrophobic interactions, surfactant-water contact, as well as head-group repulsions in the case of ionic surfactants. The calculation of thermodynamic parameters at the CMC can be done using the temperature dependence of the CMC. In water, micellization occurs via the association of the surfactants above the CMC, minimizing the free energy of the solution. Usually, phase separation and mass action models have been used to assess the various thermodynamic parameters for micellization.52 In a phase separation model, micelles and counterions are treated separately. In the mass action model, surfactant monomers and micelles are in dissociation–association equilibrium, allowing the equilibrium constant to be determined. Phase separation models assume that the total count of moles existing at the CMC equals the summation of the water and surfactant moles, while mass-action models assume that the total count of moles is the summation of water, surfactant ions, micelles, and free counterions. By considering the earlier studies and applications of the surfactant-drug mixed system, in the current work, we employed the conductivity approach to examine the relationship between CPB and LFH, in the presence of various additives (NaCl/NaOAc/NaBenz/4-ABA/urea) at various experimental temperatures (293.15–323.15 K). The several micellization parameters including CMC, fraction of counter ion binding (β), related thermodynamic parameters (ΔG0m, ΔH0m, and ΔS0m) of micellization, and thermodynamic parameters of transfer ((ΔG0m,t), (ΔH0m,t), and (ΔS0m,t)) as well as compensation parameters have been determined and reported in great detail.
2. Experimental
2.1. Chemicals
Since all of the used materials were of analytical grade, these substances were employed without any further treatment. Table 1 displays the comprehensive information on these materials. Distilled-deionized H2O with a specific conductivity below 2 × 10−6 S cm−1 was used to formulate the necessary solutions (293.15–323.15) K.
Table 1 Chemical, source, purity, and CAS number of chemicals used in this work
Chemical |
Source |
Mass fraction purity |
CAS number |
MW (g mol−1) |
LFH |
Zhejiang Jinxin, China |
0.99 |
138199-71-0 |
370.38 |
CPB |
Sigma, Aldrich, UK |
0.97 |
140-72-7 |
384.44 |
NaCl |
Merck, Mumbai, India |
0.99 |
7647-14-5 |
58.44 |
NaOAc |
Worli, Mumbai, India |
0.99 |
127-09-3 |
136.08 |
NaBenz |
BDH Chemical Ltd, England |
0.99 |
532-32-1 |
144.10 |
4-ABA |
BDH Chemical Ltd, England |
0.99 |
150-13-0 |
137.14 |
Urea |
Darmstadt, Germany |
0.99 |
57-13-6 |
60.06 |
H2O |
Distilled-deionized |
|
|
18.02 |
2.2. Conductometric technique
The conductivity of the CPB + LFH system was measured using a conductivity meter (Mettler-Toledo AG, FiveEasy TMFE30, China) with a precision of ±0.5% along with a conductivity cell (cell constant of 0.97 cm−1). The required solvent (aq. LFH/LFH + aq. NaCl/NaOAc/NaBenz/4-ABA/urea, with the specified content of LFH) was used to prepare the stock solution of CPB (25 mmol kg−1). First, 20 mL of a chosen solvent (aq. LFH/LFH + aq. NaCl/NaOAc/NaBenz/4-ABA/urea with the specified LFH) was taken in a pyrex test tube that had previously been heated in a thermostatic water bath (Appl. Sci. Technol., BD). The CPB stock solution was then carefully taken and slowly added with constant stirring. The conductivity values were then measured and noted. A KCl solution with a concentration of 0.01 M was used to calibrate the conductivity meter. All of the experiments in the current investigation followed the methodology outlined in the literature.53,54 The CMC was calculated by plotting the collected specific conductivity against the surfactant concentration using microcal origin software. Microsoft excel was used to make all other calculations.
3. Results and discussion
3.1. Assessment of the micellar parameters (CMC, and β) of CPB + LFH systems
The aggregation of the CPB + LFH mixture was examined in the current investigation using the conductivity approach. The charged amphiphiles experience ionization in the solution phase and behave similarly to those of strong electrolytes. Initially, the values of κ demonstrated the attitude of rising with the increase in the concentration of CPB owing to the participation of free CP+ and Br− ions, which have been generated from CPB molecules. However, the expected growth of κ values deviated from the former trend after reaching a certain CPB concentration, which is recognized as the critical micelle concentration (CMC).55,56 Thus, clear breakpoints in the κ vs. [CPB] plots have been achieved in the current study (Fig. 1). Moreover, in the present study, the investigations were performed in aqueous and aq. additive media in the temperature range of 293.15 to 323.15 K with a 5 K interval. Considering the room temperature, as well as the body temperature, a temperature of 303.15 K from the study range was selected to investigate the effect of concentrations of the LFH drug and other additives on the micellization phenomenon. From the collected data for κ values at experimental temperatures, we randomly selected the experimental data of κ values at 303.15 K to represent the change in nature κ with a variation of [CPB], as shown in Fig. 1.
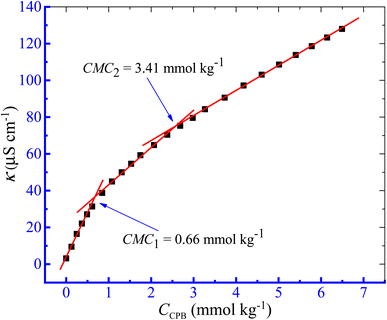 |
| Fig. 1 κ versus [CPB] plot of the CPB + LFH (1.982 mmol kg−1) mixed system in water at T = 303.15 K. | |
In the present study, two CMC values for the CPB + LFH mixture were achieved, which were designated as CMC1 and CMC2, respectively. The pre-micellar slopes were always found to be higher than that of the post-micellar slopes. Therefore, we have calculated the micelle ionization using the slope ratio between above and below the straight lines of the CMC. The α1 and α2 can be premeditated from the ratios of S2/S1 and S3/S2,57,58 respectively, if S1 and S2 are the corresponding slopes above and below CMC1, while S3 is the slope above CMC2. By deducting the value of α from one, we can calculate the fraction of counter ion binding, β1, and β2, i.e., β1 = 1 − α1 and β2 = 1 − α2. CMC1 and CMC2 signify the critical micelle concentration of CPB in the presence of LFH drug, respectively. Such results of the detection of two CMC values for the amphiphile + solute mixture have also been described in the literature.59,60
According to Dai et al.,61 the development of a double CMC may be caused via an ion–dipole interaction among the hydrophilic moieties of polymers and the amphiphile head groups. The influence of LFH, on the micellization of CPB, was examined in the current observation, and the results of the changes in CMC with the increase in LFH concentrations are disclosed in Fig. 2. The values of CMC1 of CPB micellization were increased as a function of LFH concentrations. The addition of LFH generates an unfavorable environment for the aggregation of CPB with the LFH drug. The CMC2 values first decreased up to a concentration of 5.995 mmol kg−1, reached a minimum value, and then tend to increase with the increase in [LFH], which indicates that at a lower LFH concentration, the micelle development of CPB is enhanced, while the process becomes unfavorable after attaining a LFH concentration of 5.995 mmol kg−1.
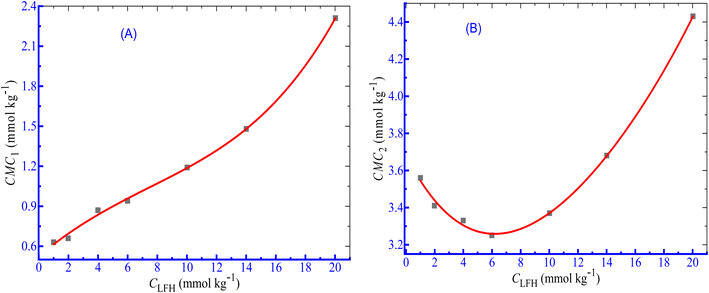 |
| Fig. 2 Change in CMC ((A) CMC1 and (B) CMC2) with [LFH] for the CPB + LFH mixture in a H2O environment at 303.15 K temperature. | |
3.2. Effect of the composition of additives on the micellar parameters (CMC, and β) of CPB + LFH systems
Here, the effects of several additives such as salts (NaCl and NaOAc), and HDTs (NaBenz, NaSal, and urea) on the solution behavior of the mixture of CPB + LFH were observed. The NaBenz was selected as the anionic HDT, and 4-ABA and urea were used as the nonionic HDTs in the present study. The changes in κ as a function of [CPB] for the association of CPB + LFH (1.98 mmol kg−1) mixture in a 4-ABA solution at 303.15 K in additive solutions are disclosed in Fig. 3. In all of the cases of micellization of the CPB + LFH mixture, two CMCs were achieved in the present study. The impacts of the concentrations of additives on the physico-chemical parameters (CMC1, CMC2, β1 and β2) of the CPB + LFH mixture are shown in Table 2.
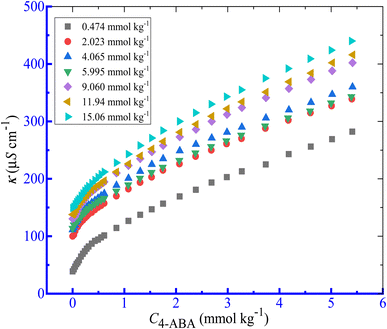 |
| Fig. 3 Conductivity (κ) vs. [CPB] plot for the CPB + LFH (1.982 mmol kg−1) mixed system in aq. solutions of 4-ABA at 303.15 K temperature. | |
Table 2 Values of critical micelle concentration (CMC1 and CMC2) and counter ion (β1 and β2) binding of CPB + LFH mixed systems in water and different additives
Media |
CLFH (mmol kg−1) |
Cadditives (mmol kg−1) |
CMC1 (mmol kg−1) |
CMC2 (mmol kg−1) |
β1 |
β2 |
H2O |
1.004 |
0 |
0.63 |
3.56 |
0.66 |
0.24 |
1.982 |
0 |
0.66 |
3.41 |
0.51 |
0.34 |
3.996 |
0 |
0.87 |
3.33 |
0.49 |
0.37 |
5.994 |
0 |
0.94 |
3.25 |
0.46 |
0.38 |
10.02 |
0 |
1.19 |
3.37 |
0.40 |
0.39 |
14.01 |
0 |
1.48 |
3.68 |
0.33 |
0.40 |
20.03 |
0 |
2.31 |
4.43 |
0.29 |
0.46 |
H2O + NaCl |
1.982 |
0.556 |
0.56 |
2.10 |
0.50 |
0.38 |
2.310 |
0.62 |
2.22 |
0.47 |
0.31 |
4.192 |
0.89 |
2.83 |
0.41 |
0.25 |
6.000 |
0.91 |
3.09 |
0.23 |
0.20 |
8.941 |
1.16 |
3.26 |
0.28 |
0.18 |
15.23 |
1.31 |
3.88 |
0.38 |
0.15 |
H2O + NaOAc |
1.982 |
0.478 |
0.33 |
1.90 |
0.63 |
0.39 |
2.076 |
0.46 |
1.98 |
0.49 |
0.35 |
3.968 |
0.55 |
2.12 |
0.47 |
0.31 |
5.989 |
0.60 |
2.22 |
0.45 |
0.28 |
9.039 |
0.84 |
2.43 |
0.43 |
0.25 |
12.07 |
1.04 |
2.67 |
0.41 |
0.21 |
14.88 |
1.23 |
2.97 |
0.34 |
0.14 |
H2O + NaBenz |
1.982 |
0.486 |
0.61 |
2.23 |
0.50 |
0.39 |
1.960 |
0.84 |
2.54 |
0.19 |
0.35 |
3.990 |
0.92 |
2.65 |
0.18 |
0.25 |
6.002 |
0.99 |
2.88 |
0.17 |
0.22 |
9.004 |
1.15 |
3.25 |
0.20 |
0.19 |
11.99 |
0.98 |
3.01 |
0.24 |
0.14 |
14.99 |
0.68 |
2.27 |
0.41 |
0.12 |
H2O + 4-ABA |
1.982 |
0.474 |
0.33 |
2.31 |
0.37 |
0.63 |
2.023 |
0.35 |
2.33 |
0.59 |
0.40 |
4.065 |
0.37 |
2.35 |
0.62 |
0.39 |
5.995 |
0.41 |
2.40 |
0.58 |
0.32 |
9.06 |
0.46 |
2.48 |
0.55 |
0.30 |
11.94 |
0.53 |
2.56 |
0.48 |
0.29 |
15.06 |
0.61 |
2.68 |
0.47 |
0.28 |
H2O + urea |
1.982 |
100.2 |
0.55 |
2.14 |
0.59 |
0.38 |
200.0 |
0.54 |
2.12 |
0.60 |
0.36 |
399.9 |
0.53 |
2.10 |
0.64 |
0.35 |
599.9 |
0.52 |
2.07 |
0.59 |
0.37 |
1000 |
0.49 |
2.03 |
0.57 |
0.40 |
1500 |
0.46 |
1.96 |
0.55 |
0.42 |
2000 |
0.43 |
1.90 |
0.53 |
0.43 |
In an aq. system of NaCl and NaOAc, the CMC1 and CMC2 values of the CPB + LFH mixture have been detected to be augmented with the concentration of salts. However, in the study ranges of salt concentration, the CMC1 and CMC2 values of the CPB + LFH mixture were lower in magnitudes compared to those values detected in a water medium for the same system. The CMC1 values of the CPB + LFH mixture in an aq. NaBenz medium first increased, attained a maximum point, and then experienced a drop with the upsurge of the contents of NaBenz. Thus, the aggregation phenomenon was achieved to be disfavored in the attendance of NaBenz. The CMC2 values of the CPB + LFH mixture in an aq. NaBenz medium are always lesser in magnitude than the CMC2 value obtained in an aq. medium. The CMC2 values of the CPB + LFH mixture in this case first increased, achieved a maximum point, and then endured a decrease with the upsurge of [NaBenz]. Thus, the micelle formation phenomenon of the CPB + LFH mixture has been achieved to be favored in the attendance of NaBenz.
In an aq. solution of 4-ABA, the CMC1 and CMC2 values of the CPB + LFH mixture were achieved to be much lower in magnitude than that the CMC values found in water under an identical condition. However, in the present study, in the ranges of 4-ABA concentration, the CMC1 and CMC2 values of the CPB + LFH mixture were achieved to be enhanced as a function of the concentration of 4-ABA. The CMC1 and CMC2 values of the CPB + LFH mixture experience a depression with the augmentation of urea concentration. The findings showed that the appearance of urea generates a favorable condition for the formation of micellar structures in the mixture of CPB + LFH. Rather and co-workers62 studied the effect of alcohols and temperatures on the micellization of CPB in polyvinyl alcohol (PVA) media and found out the micellization parameters and the nature of interaction forces present in the employed media, which are comparable with what we have achieved in our present study.
3.3. Effect of temperature on CPB + LFH mixture in salt/HDT media
The effect of temperature in the range of 293.15 to 323.15 K on the micellization of the CPB + LFH mixture has been performed in the present investigation. The conductivities of the CPB + LFH mixture were boosted through the enhancement of temperature. As for example, plots of κ versus [CPB] for the association of the CPB + LFH mixture in aq. additive media are shown in Fig. 4. The CMC1 value of the CPB + LFH mixture in an aqueous medium first increased, achieved the highest value, and then decreased via an increase in study temperature, while the CMC2 values of the same system demonstrated the opposite trend in comparison to the CMC1 values with a similar bump of study temperature.
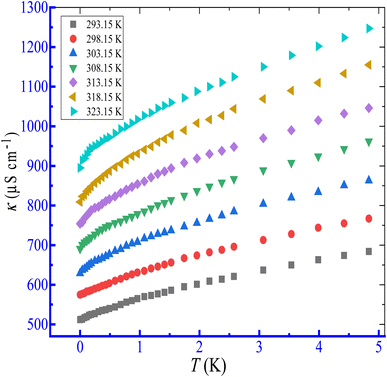 |
| Fig. 4 Plots of κ versus T for the association of the CPB + LFH (1.983 mmol kg−1) mixed system in an aq. NaOAc (5.989 mmol kg−1) solution at the ambient experimental temperatures. | |
The CMC1 values of the CPB + LFH mixture in an aq. NaCl medium diminished through the increase in experimental temperature, which exposes that the aggregation was favored with the growth of temperature. Moreover, the CMC2 values of the CPB + LFH mixture in an aq. NaCl medium increased, reached the maximum value, and then decreased with the upsurge of experimental temperature. The CMC1 and CMC2 values of the CPB + LFH mixture in an aq. NaOAc medium were found to experience a decline with the enhancement of experimental temperature, which exposes that the micelle formation has been favored through the rise in temperature. The CMC1 and CMC2 values of the CPB + LFH mixture in an aq. NaBenz medium decreased through the increase in experimental temperature, while the values in aq. 4-ABA exhibited an increasing nature through the rise in temperature. In an aq. urea solution, however, the CMC1 and CMC2 values of the CPB + LFH mixture showed the tendency to undergo an enhancement as a function of temperature, which also follow an analogous behavior to those values found in the aq. 4-ABA solution (Fig. 5).
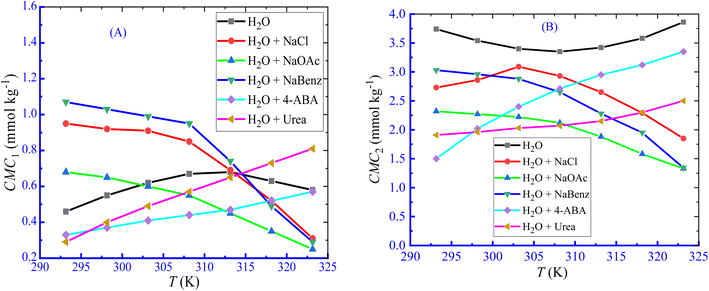 |
| Fig. 5 Plots of CMC ((A) CMC1 and (B) CMC2) against T for the assembly of the CPB + LFH (1.983 mmol kg−1) mixture in different additive media at different study temperatures. | |
The variation in CMC values with an elevated temperature can be justified by the impact of temperature on the possible modes of hydrations surrounding the polar/nonpolar moieties of the surfactants. Only two types of hydrations, namely, hydrophobic hydration (H2O structure surrounding the nonpolar tail) and hydrophilic hydration (H2O arrangement nearby the hydrophilic head of surfactant), are possible for surfactant molecules before aggregation takes place, but after the development of micelles, only the hydrophilic hydration has the possibility to happen. With the increase in temperature, the magnitude of both categories of hydrations suffers a reduction. The reduction in the degree of hydrophobic hydration causes a decrease in the hydrophobic interaction between the nonpolar parts of amphiphiles, which hampers the micelle development progression (i.e., an upsurge of CMC is achieved).63–65 In contrast, a lessening in the amount of hydrophilic hydration causes improvement in the hydrophobic nature, which augments the micellization (i.e., a decline of CMC has happened).63–65 In an aqueous medium, however, at lower temperatures, the second factor dominates, while the first factor becomes influencing at higher temperatures. In contrast, the reverse effect has been found in the case of aq. NaCl in comparison to a water medium. In aq. NaOAc and NaBenz media, the second factor exerts the dominating effect, and the first factor dominates over the second one in aq. 4-ABA and urea solutions over the temperature ranges studied (Table 3). The values of XCMC1 , XCMC1, β1, and β1 of studied system at different temperatures are exposed in Table 3.
Table 3 Micellar parameters of the aggregation of the mixture of CPB and (1.982 mmol kg−1) LFH in aq. solutions of NaCl, NaOAc, NaBenz, 4-ABA, and urea media at different study temperatures
Medium |
Cadditives (mmol kg−1) |
T (K) |
XCMC1 (×105) |
XCMC2 (×105) |
β1 |
β2 |
H2O |
|
293.15 |
0.8289 |
6.739 |
0.71 |
0.19 |
298.15 |
0.9911 |
6.378 |
0.64 |
0.30 |
303.15 |
1.117 |
6.126 |
0.59 |
0.34 |
308.15 |
1.207 |
6.036 |
0.53 |
0.39 |
313.15 |
1.225 |
6.162 |
0.48 |
0.40 |
318.15 |
1.135 |
6.451 |
0.41 |
0.45 |
323.15 |
1.045 |
6.955 |
0.35 |
0.48 |
H2O + NaCl |
6.000 |
293.15 |
1.712 |
4.919 |
0.11 |
0.11 |
298.15 |
1.658 |
5.153 |
0.13 |
0.12 |
303.15 |
1.640 |
5.567 |
0.24 |
0.20 |
308.15 |
1.531 |
5.279 |
0.48 |
0.22 |
313.15 |
1.243 |
4.774 |
0.52 |
0.23 |
318.15 |
0.9369 |
4.126 |
0.64 |
0.31 |
323.15 |
0.5585 |
3.333 |
0.68 |
0.37 |
H2O + NaOAc |
5.989 |
293.15 |
1.117 |
4.180 |
0.31 |
0.20 |
298.15 |
1.171 |
4.090 |
0.37 |
0.22 |
303.15 |
1.081 |
4.000 |
0.45 |
0.28 |
308.15 |
0.9909 |
3.820 |
0.46 |
0.31 |
313.15 |
0.8108 |
3.387 |
0.47 |
0.39 |
318.15 |
0.6306 |
2.847 |
0.49 |
0.42 |
323.15 |
0.4504 |
2.396 |
0.57 |
0.44 |
H2O + NaBenz |
6.002 |
293.15 |
1.928 |
5.459 |
0.09 |
0.49 |
298.15 |
1.856 |
5.333 |
0.10 |
0.30 |
303.15 |
1.784 |
5.189 |
0.18 |
0.22 |
308.15 |
1.712 |
4.774 |
0.22 |
0.20 |
313.15 |
1.333 |
4.108 |
0.26 |
0.17 |
318.15 |
0.8828 |
3.513 |
0.35 |
0.15 |
323.15 |
0.5225 |
2.414 |
0.40 |
0.11 |
H2O + 4-ABA |
5.995 |
293.15 |
0.5945 |
2.703 |
0.54 |
0.41 |
298.15 |
0.6667 |
3.639 |
0.57 |
0.40 |
303.15 |
0.7387 |
4.324 |
0.58 |
0.32 |
308.15 |
0.7747 |
4.882 |
0.66 |
0.28 |
313.15 |
0.8828 |
5.315 |
0.68 |
0.27 |
318.15 |
1.099 |
5.621 |
0.69 |
0.22 |
323.15 |
1.261 |
6.035 |
0.69 |
0.19 |
H2O + urea |
1000.12 |
293.15 |
0.513 |
3.381 |
0.63 |
0.45 |
298.15 |
0.708 |
3.469 |
0.6 |
0.42 |
303.15 |
0.867 |
3.593 |
0.57 |
0.40 |
308.15 |
1.009 |
3.664 |
0.56 |
0.35 |
313.15 |
1.151 |
3.805 |
0.54 |
0.33 |
318.15 |
1.292 |
4.071 |
0.53 |
0.32 |
323.15 |
1.434 |
4.425 |
0.52 |
0.30 |
3.4. Equivalent conductivity at infinite dilution and aggregation number of the micellization of CPB in the presence of LFH drug
The equivalent conductivity at infinite dilution (Λ0) was determined by extrapolating the plot of equivalent conductivity (Λ) vs.
of the CPB + LFH mixture in an aqueous medium. For this purpose, the conductivity values of the CPB + LFH mixture for the low concentration ranges of the cationic surfactant were utilized. The acquired Λ0 values of the CPB + LFH mixture in an aqueous medium are given in Table 4. The Λ0 values experienced an increase with the increase in temperature, with an exception at 298.15 K.
Table 4 Values of equivalent conductivity at infinite dilution (Λ0) and aggregation number (Nagg) of CPB + LFH (1.982 mmol kg−1) drug mixture in an aqueous medium
T (K) |
Λ0 (cm2 ohm−1 mol−1) |
Nagg |
293.15 |
99 |
125 |
298.15 |
96 |
113 |
303.15 |
104 |
71 |
308.15 |
122 |
70 |
313.15 |
198 |
105 |
318.15 |
212 |
130 |
323.15 |
280 |
148 |
The micelle aggregation numbers (Nagg) have been calculated utilizing the following equation (eqn (1)):65
|
 | (1) |
where
Λ0 is the equivalent conductivity at infinite dilution for the CPB in the presence of LFH drug,
ΛCMC is the equivalent conductivity of CPB just at the
CCMC,
Λ is the equivalent conductivity of CPB at different concentrations and
N represents the values of aggregation number (
Nagg). The values of aggregation number (
Nagg) were calculated from the slope of
vs. 
plot. The
Nagg values for the CPB + LFH mixture in an aqueous medium are given in
Table 4. The
Nagg values for the CPB + LFH mixture initially tend to decrease, attained the minimum value, and after that, the values again have increased with the upsurge of temperature. This result also supports the changes in CMC with an enhanced temperature. Mata and co-workers reported the aggregation number (
Nagg) for the assembly of CPB in an aqueous medium using eqn
(1). They achieved the values of
Nagg of 111, 106, 99, and 90 at 303.15, 313.15, 323.15, and 333.15 K, respectively, for the micelle formation of CPB in an aqueous medium.
65 In the molecular simulation scheme, 122 CPB molecules were also required to construct a spherical CPB micelle in an aqueous medium.
42,66 Consequently, the present investigation exhibits a good agreement with the literature values.
42,66
3.5. Thermodynamic parameters of micellization in CPB + LFH systems in salts and HDTs media
The thermodynamic parameters of micellization (free energy change (ΔG0m), change of enthalpy (ΔH0m), and change of entropy (ΔS0m) for ionic amphiphiles of the 1
:
1 electrolyte nature were calculated using the following equations (eqn (2)–(6)):67–69 |
ΔG0m = (1 + β)RTln XCMC
| (2) |
|
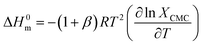 | (3) |
|
ln XCMC = A + BT + CT2
| (4) |
|
ΔH0m = −(1 + β)RT2(B + 2CT)
| (5) |
|
ΔS0m = (ΔH0m − ΔG0m)/T
| (6) |
In these equations, the term R stands for gas constant, XCMC is the mole fraction of CPB at CMC, and β is the counter ion binding adhered at various temperatures. The values of XCMC were determined by dividing the total moles of amphiphile at CMC and the moles of all components there in the solution. The regression coefficients (A, B, and C shown in eqn (4)) were obtained by fitting the second-order polynomial (Fig. 6), and their values are displayed in Table 5.
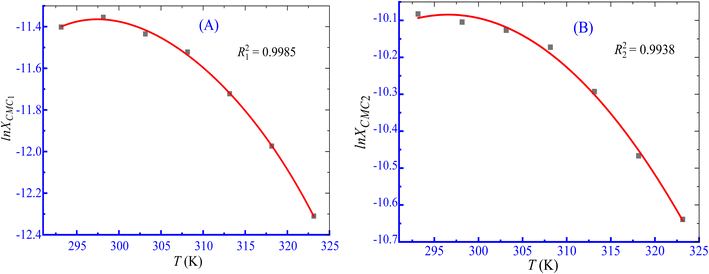 |
| Fig. 6 ln XCMC vs. T second-order fitting plots for the association of the CPB + LFH (1.983 mmol kg−1) mixture in an aq. NaOAc (5.989 mmol kg−1) solution ((A) CMC1 and (B) CMC2). | |
Table 5 Variation in enthalpies for the assembly of CPB + LFH (1.982 mmol kg−1) in aq. salt/HDT media within 293.15 to 323.15 K, as determined by the values of the fitting parameters
Medium |
Csalts/HDTs (mmol kg−1) |
A1 |
B1 |
C1 |
R12 |
A2 |
B2 |
C2 |
R22 |
H2O |
0 |
−122.78 |
0.7158 |
−0.0011 |
0.9953 |
42.135 |
−0.3374 |
0.0005 |
0.9961 |
H2O + NaCl |
6.0000 |
−223.02 |
1.410 |
−0.0023 |
0.9909 |
−119.49 |
0.7242 |
−0.0012 |
0.9922 |
H2O + NaOAc |
5.9890 |
−166.45 |
0.7132 |
−0.0012 |
0.9990 |
−79.536 |
0.4684 |
−0.0008 |
0.9958 |
H2O + NaBenz |
6.0020 |
−221.57 |
1.342 |
−0.0022 |
0.9888 |
−116.96 |
0.7197 |
−0.0012 |
0.9874 |
H2O + 4-ABA |
5.9950 |
1.8228 |
−0.120 |
0.0002 |
0.9953 |
−98.420 |
0.5495 |
−0.0009 |
0.9904 |
H2O + urea |
1000.12 |
−92.181 |
0.4911 |
−0.0007 |
0.9951 |
9.0570 |
−0.1339 |
0.0002 |
0.9867 |
The values of ΔG0m, ΔH0m, and ΔS0m of the CPB + LFH system in water and aq. salts/HDTs solutions are disclosed in Table 6. In all of the solvents employed in the current investigation, the acquired ΔG0m values were negative, which displays that the micelle development is a spontaneous phenomenon. In H2O, aq. NaCl, aq. NaOAc, aq. NaBenz, and aq. 4-ABA solutions, the −ΔG0m values were found to be enhanced/reduced with an upsurge in temperature, which discloses that the extent of spontaneity has been increased/decreased with the increase in temperature. In aq. urea solutions, the −ΔG0m values are found to be decreased with the increase in temperature, which discloses that the extent of spontaneity has been lessened with the increase in temperature.
Table 6 Values of thermodynamics parameter of micellization for CPB and CPB + LFH (1.982 mmol kg−1) in aq. salt/HDT media
Medium |
Cadditive (mmol kg−1) |
T (K) |
ΔG0m,1 (kJ mol−1) |
ΔG0m,2 (kJ mol−1) |
ΔH0m,1 (kJ mol−1) |
ΔH0m,2 (kJ mol−1) |
ΔS0m,1 (J mol−1 K−1) |
ΔS0m,2 (J mol−1 K−1) |
H2O |
0 |
293.15 |
−48.76 |
−27.86 |
−86.59 |
37.62 |
−129.0 |
223.4 |
298.15 |
−46.84 |
−31.13 |
−72.57 |
37.71 |
−86.29 |
230.9 |
303.15 |
−45.69 |
−32.76 |
−59.37 |
35.07 |
−45.12 |
223.7 |
308.15 |
−44.39 |
−34.60 |
−45.74 |
32.10 |
−4.39 |
216.4 |
313.15 |
−43.58 |
−35.34 |
−32.42 |
27.68 |
35.63 |
201.2 |
318.15 |
−42.47 |
−37.01 |
−18.83 |
23.49 |
74.29 |
190.2 |
323.15 |
−41.60 |
−38.07 |
−5.708 |
18.31 |
111.1 |
174.5 |
H2O + NaCl |
6.00 |
293.15 |
−29.69 |
−26.84 |
−48.78 |
−16.37 |
−65.12 |
35.71 |
298.15 |
−30.83 |
−27.41 |
−32.16 |
−7.152 |
−4.455 |
35.71 |
303.15 |
−34.44 |
−29.63 |
−14.69 |
3.081 |
65.12 |
107.9 |
308.15 |
−42.04 |
−30.78 |
8.751 |
14.79 |
164.8 |
147.9 |
313.15 |
−44.70 |
−31.86 |
37.78 |
27.44 |
263.4 |
189.4 |
318.15 |
−50.23 |
−34.98 |
73.82 |
43.39 |
389.9 |
246.3 |
323.15 |
−54.59 |
−37.94 |
111.6 |
61.09 |
514.2 |
306.5 |
H2O + NaOAc |
5.989 |
293.15 |
−36.11 |
−29.49 |
−9.020 |
0.549 |
92.40 |
102.5 |
298.15 |
−38.56 |
−30.56 |
−16.08 |
7.790 |
75.41 |
128.6 |
303.15 |
−41.79 |
−32.67 |
−2.083 |
16.27 |
131.0 |
161.4 |
308.15 |
−43.10 |
−34.14 |
13.97 |
25.48 |
185.2 |
193.5 |
313.15 |
−44.86 |
−37.25 |
31.30 |
36.99 |
243.2 |
237.1 |
318.15 |
−47.19 |
−39.31 |
50.31 |
48.56 |
306.5 |
276.2 |
323.15 |
−51.93 |
−41.16 |
73.77 |
60.81 |
389.0 |
315.6 |
H2O + NaBenz |
6.002 |
293.15 |
−28.84 |
−35.65 |
−40.84 |
−17.18 |
−40.93 |
62.98 |
298.15 |
−29.71 |
−32.68 |
−24.75 |
−4.100 |
16.60 |
95.86 |
303.15 |
−32.52 |
−30.34 |
−7.609 |
7.327 |
82.20 |
124.2 |
308.15 |
−34.30 |
−30.59 |
13.06 |
18.81 |
153.7 |
160.3 |
313.15 |
−36.82 |
−30.77 |
36.53 |
30.39 |
234.2 |
195.3 |
318.15 |
−41.56 |
−31.20 |
65.39 |
42.45 |
336.2 |
231.5 |
323.15 |
−45.75 |
−31.71 |
96.70 |
53.83 |
440.8 |
264.7 |
H2O + 4-ABA |
5.995 |
293.15 |
−45.78 |
−36.15 |
3.125 |
−21.99 |
166.8 |
48.29 |
298.15 |
−47.07 |
−35.47 |
0.975 |
−13.27 |
161.1 |
74.45 |
303.15 |
−47.68 |
−33.43 |
−1.400 |
−3.863 |
152.7 |
97.54 |
308.15 |
−50.05 |
−32.55 |
−4.141 |
5.224 |
149.0 |
122.6 |
313.15 |
−50.90 |
−32.54 |
−7.068 |
14.67 |
140.0 |
150.8 |
318.15 |
−51.04 |
−31.58 |
−10.18 |
23.79 |
128.4 |
174.0 |
323.15 |
−51.22 |
−31.06 |
−13.44 |
33.24 |
116.9 |
199.0 |
H2O + urea |
1000.12 |
293.15 |
−48.39 |
−36.38 |
−93.97 |
17.24 |
−155.5 |
182.9 |
298.15 |
−47.03 |
−36.15 |
−87.14 |
15.36 |
−134.5 |
172.8 |
303.15 |
−46.12 |
−36.11 |
−80.00 |
13.52 |
−111.8 |
163.7 |
308.15 |
−45.98 |
−35.33 |
−73.51 |
11.34 |
−89.35 |
151.4 |
313.15 |
−45.60 |
−35.24 |
−66.16 |
9.369 |
−65.65 |
142.4 |
318.15 |
−45.56 |
−35.21 |
−58.83 |
7.376 |
−41.72 |
133.8 |
323.15 |
−45.54 |
−35.02 |
−51.06 |
5.237 |
−17.06 |
124.6 |
The ΔH0m,1 and ΔH0m,2 values for the CPB + LFH system in water/urea are negative and positive, respectively, while the −ΔH0m,1 and +ΔH0m,2 values tend to decrease with the rise in temperature. Consequently, the micellization is endothermic in nature in an aq. environment. The ΔS0m,1 values for the CPB + LFH system in water/urea are negative (except few cases), the values tend to decrease and turn to be positive with the increase in temperature, while the ΔS0m,2 values are positive within the temperatures studied, the +ΔS0m,2 values tend to decrease with of the rise in temperature. In aq. NaCl and NaBenz solutions, the ΔH0m,1 and ΔH0m,2 values for the CPB + LFH system are negative at lower study temperatures (293.15–303.15 K), while the values are positive at higher investigational temperatures (308.15–323.15 K). Subsequently, the association of the analyzed system in aq. NaCl is exothermic in nature, while the process occurs endothermic in character with the increase in temperature. The ΔS0m,1 and ΔS0m,2 values for the CPB + LFH system are almost positive (except some few cases) within the study temperatures, except ΔS0m,1 at 293.15 and 298.15 K (−ΔS0m,1). Again, the ΔH0m,1 and ΔH0m,2 values for the CPB + LFH system in aq. NaOAc solutions are almost positive within the study temperatures, except ΔH0m,1 at 293.15, 298.15, and 303.15 K (−ΔH0m,1). Furthermore, the ΔS0m,1 and ΔS0m,2 values for the CPB + LFH system are positive within the study temperatures, while the positive entropy values have the tendency to increase with the increase in temperature.70–72 In aq. 4-ABA solutions, the ΔH0m,1 values for the CPB + LFH system are positive and negative in nature at lower and higher study temperatures, respectively, while the ΔH0m,2 values demonstrate the opposite nature. In addition, the ΔS0m,1 and ΔS0m,2 values for the CPB + LFH system display the positive trend within the range of study temperatures. Consequently, the micellization is both enthalpy and entropy driven, while the contribution of entropy is significant over that of enthalpy.
The acquired positive enthalpies of ΔH0m were claimed to be related to the rupturing of water structure (H-bonding) on the exterior portions of hydrophobic regions.70–72 According to Nusselder & Engberts,73 the negative ΔH0m values imply the existence of London-dispersion forces, which cause the surfactant monomers to aggregate in the solution phase. The values of ΔH0m and ΔS0m disclose that the suggested interaction forces between CPB and LFH are exothermic (ion–dipole and dipole–dipole types), as well as hydrophobic interactions, and the micellization is caused by the contribution of both enthalpy and entropy.
3.6. Thermodynamic properties of transfer
The transfer properties such as free energy change (ΔG0m,t), enthalpy (ΔH0m,t) and entropy (ΔS0m,t) of transfer were calculated for the shift of CPB + LFH from water to aq. NaCl/NaOAc/NaBenz/4-ABA/urea solutions using the following equations:74,75 |
ΔG0m,t = ΔG0m(aq. additive) − ΔG0m(aq.)
| (7) |
|
ΔH0m.t = ΔH0m(aq. additive) − ΔH0m(aq.)
| (8) |
|
ΔS0m,t = ΔS0m(aq. additive) − ΔS0m(aq.)
| (9) |
The thermodynamics of transfer for the micellization of CPB + LFH, in several aq. additive media, was determined at different temperatures, and the results are given in Table 7. The negative values of ΔG0m,t indicate that the micellization of CPB + LFH is more spontaneous in aq. additive media, while the positive values of ΔG0m,t of the CPB + LFH mixture in aq. additive media indicate that the micellization is less spontaneous in aq. additive media, thereby used in the present study. The values of ΔH0m,1,t of the CPB + LFH mixture are positive in aq. additives (NaCl, NaOAc, NaBenz, and 4-ABA) media, while the values of ΔH0m,1,t for the same system in an aq. urea solution are negative in magnitudes. The ΔH0m,2,t values of the CPB + LFH mixture are negative and positive at lower and higher temperatures, respectively, in aq. additive (NaCl, NaOAc, NaBenz, and 4-ABA) media, while the ΔH0m,2,t values of the same system in an aq. urea solution are negative in magnitudes. The negative, along with the positive, values of ΔH0m,t disclose, respectively, the more exothermic and endothermic natures of micellization of the system. In addition, the values of ΔS0m,1,t for the CPB + LFH mixture are positive in aq. additive (NaCl, NaOAc, NaBenz, and 4-ABA) media, while the values of ΔS0m,1,t for the same system in an aq. urea solution are negative in magnitudes. Furthermore, the values of ΔS0m,2,t for the CPB + LFH mixture are negative and positive at lower and higher temperatures, respectively, in aq. additive (NaCl, NaOAc, NaBenz, and 4-ABA) media, while the values of ΔH0m,2,t for the same system in an aq. urea solution are negative in magnitudes. Again, the negative, along with the positive, values of ΔS0m,t reveal the more ordered and more disordered states of the micellized system, respectively.
Table 7 Thermodynamic properties of transfer (free energy (ΔG0m,t), enthalpy (ΔH0m,t) and entropy (ΔS0m,t)) for the CPB + LFH (1.982 mmol kg−1) system in salt/HDT media
Medium |
Csalt/HDT (mmol kg−1) |
T (K) |
ΔG0m,1,t (kJ mol−1) |
ΔH0m,1,t (kJ mol−1) |
ΔS0m,1,t (J mol−1 K−1) |
ΔG0m,2,t (kJ mol−1) |
ΔH0m,2,t (kJ mol−1) |
ΔS0m,2,t (J mol−1 K−1) |
H2O + NaCl |
6.00 |
293.15 |
19.07 |
37.80 |
63.90 |
1.021 |
−53.99 |
−187.7 |
298.15 |
16.01 |
40.40 |
81.83 |
3.718 |
−44.86 |
−162.9 |
303.15 |
11.53 |
44.79 |
109.7 |
3.133 |
−31.99 |
−115.8 |
308.15 |
2.352 |
54.49 |
169.2 |
3.812 |
−17.30 |
−68.53 |
313.15 |
−1.120 |
70.21 |
227.8 |
3.474 |
−0.242 |
−11.87 |
318.15 |
−7.760 |
92.65 |
315.6 |
2.025 |
19.90 |
56.19 |
323.15 |
−13.00 |
117.3 |
403.1 |
0.1221 |
42.78 |
132.0 |
H2O + NaOAc |
5.989 |
293.15 |
12.65 |
77.56 |
221.4 |
−1.631 |
−37.07 |
−120.9 |
298.15 |
8.278 |
56.49 |
161.7 |
0.572 |
−29.92 |
−102.3 |
303.15 |
3.903 |
57.29 |
176.1 |
0.091 |
−18.79 |
−62.29 |
308.15 |
1.292 |
59.71 |
189.6 |
0.455 |
−6.615 |
−22.94 |
313.15 |
−1.286 |
63.73 |
207.6 |
−1.913 |
9.310 |
35.84 |
318.15 |
−4.726 |
69.14 |
232.2 |
−2.307 |
25.07 |
86.06 |
323.15 |
−10.33 |
79.48 |
277.9 |
−3.094 |
42.50 |
141.1 |
H2O + NaBenz |
6.002 |
293.15 |
19.92 |
45.75 |
88.09 |
−7.788 |
−54.80 |
−160.4 |
298.15 |
17.13 |
47.82 |
102.9 |
−1.553 |
−41.81 |
−135.0 |
303.15 |
13.17 |
51.76 |
127.3 |
2.423 |
−27.74 |
−99.50 |
308.15 |
10.09 |
58.80 |
158.1 |
4.008 |
−13.28 |
−56.11 |
313.15 |
6.755 |
68.95 |
198.6 |
4.570 |
2.712 |
−5.933 |
318.15 |
0.909 |
84.22 |
261.9 |
5.808 |
18.96 |
41.33 |
323.15 |
−4.148 |
102.4 |
329.8 |
6.361 |
35.52 |
90.24 |
H2O + 4-ABA |
5.995 |
293.15 |
2.984 |
89.71 |
295.8 |
−8.290 |
−59.61 |
−175.1 |
298.15 |
−0.232 |
73.54 |
247.4 |
−4.342 |
−50.99 |
−156.4 |
303.15 |
−1.990 |
57.97 |
197.8 |
−0.670 |
−38.93 |
−126.2 |
308.15 |
−5.658 |
41.60 |
153.4 |
2.042 |
−26.87 |
−93.84 |
313.15 |
−7.323 |
25.355 |
104.4 |
2.792 |
−13.01 |
−50.45 |
318.15 |
−8.578 |
8.648 |
54.14 |
5.426 |
0.299 |
−16.12 |
323.15 |
−9.623 |
−7.732 |
5.852 |
7.006 |
14.93 |
24.51 |
H2O + urea |
1000.12 |
293.15 |
0.3780 |
−7.386 |
−26.48 |
−8.362 |
−9.330 |
−3.302 |
298.15 |
−0.191 |
−14.57 |
−48.23 |
−4.911 |
−12.20 |
−24.44 |
303.15 |
−0.427 |
−20.63 |
−66.64 |
−3.350 |
−12.27 |
−29.43 |
308.15 |
−1.588 |
−27.77 |
−84.97 |
−0.731 |
−12.58 |
−38.46 |
313.15 |
−2.019 |
−33.73 |
−101.3 |
0.098 |
−11.08 |
−35.69 |
318.15 |
−3.090 |
−40.00 |
−116.0 |
1.801 |
−9.82 |
−36.51 |
323.15 |
−3.947 |
−45.35 |
−128.1 |
3.050 |
−7.802 |
−33.58 |
3.7. Enthalpy–entropy compensation for the CPB + LFH system in aq. salt/HDT media
The linear correlation between ΔH0m and ΔS0m (Fig. 7) can be employed to calculate the enthalpy–entropy compensation parameter and eqn (10) was employed to compute the parameters for the current investigation:76–83 |
 | (10) |
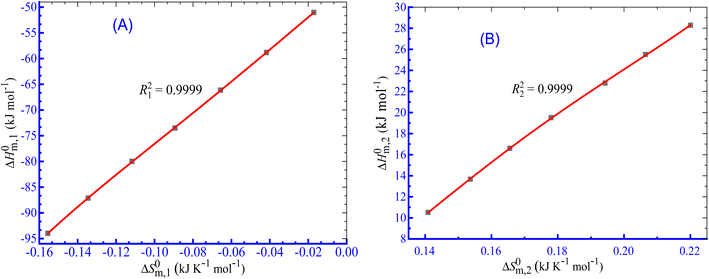 |
| Fig. 7 Enthalpy–entropy compensation plots for the CPB + LFH (1.98 mmol kg−1) system in aq. urea (1000.12 mmol kg−1) solutions ((A) for results from CMC1 and (B) results from CMC2). | |
The slope, Tc, in the above-mentioned equation refers to the compensation temperature and the intercept,
stands for the intrinsic enthalpy gain. The slope and intercept of the ΔH0m–ΔS0m plots, can be used to determine the values of Tc and
respectively.
To investigate the compensatory phenomenon between the enthalpy and entropy of micellization, the plots of ΔH0m–ΔS0m have been drawn. In the present case, the linear relationship between ΔH0m and ΔS0m was acquired in all solvent media employed; such a relationship is called the enthalpy-entropy compensation. The values of compensation temperature (Tc), intrinsic enthalpy gain
and R2 for the assembly of the CPB + LFH mixture in aq. and aq. additive media are presented in Table 8. The negative values of
indicate the formation of stable surfactant aggregates.80–82 An increase in the negative values of
indicates that the structure of micelles is becoming increasingly stable. The values of
represent the nature and extent of solute–solute interaction.
Table 8 Different variables acquired for the enthalpy–entropy compensation study
Media |
Cadditives (mmol kg−1) |
ΔH0m,1 (kJ mol−1) |
Tc,1 (K) |
R12 |
ΔH0m,2 (kJ mol−1) |
Tc,2 (K) |
R22 |
H2O |
0 |
−43.79 |
335.9 |
0.9997 |
−44.02 |
356.2 |
0.9819 |
H2O + NaCl |
6.000 |
−32.67 |
274.9 |
0.9983 |
−32.58 |
274.6 |
0.9997 |
H2O + NaOAc |
5.989 |
−37.09 |
287.0 |
0.9990 |
−28.64 |
280.6 |
0.9992 |
H2O + NaBenz |
6.002 |
−30.06 |
285.3 |
0.9997 |
−36.94 |
344.7 |
0.9981 |
H2O + 4-ABA |
5.995 |
−53.28 |
335.8 |
0.9894 |
−39.99 |
366.6 |
0.9995 |
H2O + urea |
1000.12 |
−45.88 |
307.9 |
0.9999 |
−19.94 |
204.4 |
0.9976 |
The higher negative values of
disclose that the most stable aggregate was formed in a H2O medium, and the least stable aggregate was formed in an aq. urea medium, while intermediate stable aggregates were developed in aq. salt/NaBenz and 4-ABA media. For studying the participation of water in the cases of formulation of proteins, numerical values of Tc have been used. For proteins and solutions of several small solutes, the Tc values in the range of 270–350 K have been reported.77 In the current investigation, the Tc values of the CPB + LFH system were determined to be in the range of 204.4–366.6 K in aq. salt/HDT solutions. The Tc values of the CPB + LFH system in the solvents utilized follow the order: Tc (aq. 4-ABA) > Tc (H2O) > Tc (aq. NaBenz) > Tc (aq. NaOAc) > Tc (aq. NaCl) > Tc (aq. urea). The Tc values of CPB + LFH in the employed medium, with a few exceptions, nearly fall identical to those values found in biological fluids. The Tc values in the present study are also comparable to those values computed earlier for the micellization of ionic surfactants in an environment carried out in the presence of aq. additives.83
4. Conclusions
In this work, the micellization of a mixture of CPB and LFH in aq. salt/HDT solutions at various contents and temperatures have been studied by means of the conductivity technique. The introduction of LFH generates a favorable environment for the micelle formation up to 6 mmol kg−1 of LFH drug, and the micelle development of the CPB + LFH mixture happens at greater CPB concentrations when the concentration of LFH attains > 6 mmol kg−1 in the employed solution. The micellization of the CPB + LFH mixture experiences an augmentation in aq. NaCl, NaOAc, NaBenz, 4-ABA, and urea media in comparison to aqueous solutions, which has been detected by the reduction of CMC values. The CMC values were also altered with the compositions of the solvents used. The conductivities and micellar parameters (CMC, and β) have been varied with the change in experimental temperature and additive contents. For the assembly of the CPB + LFH system, the ΔG0m values were obtained to be all negative. The values of ΔH0m and ΔS0m reveal that the proposed interaction forces between CPB and LFH are ion–dipole and dipole–dipole types, as well as hydrophobic in nature. The micellization phenomenon was mainly entropy contributed, which has been proved by the positive entropy changes of micellization. The Tc values of the CPB + LFH system were acquired to be in the range of 204.4–366.6 K in aqueous and aq. salt/HDT solutions. The Tc values of CPB + LFH in the employed solvents, with a few exceptions, demonstrated a nearly identical behavior with those values found in biological fluids. The
values in the range of −19.94 to −44.02 kJ mol−1 exhibited stable aggregate creation in the case of the CPB + LFH mixture. In order to reduce the degradation of medication, to avoid the negative side effects, to increase the drug bioavailability, and also to make it easier to control drug absorption, surfactants are widely utilized in drug distribution and drug targeting strategies. Surfactant micelles make the feebly water-soluble medicines to enter into solutions, thereby enhancing their bioavailability, as a result of which they can stay in the blood for a long enough time to allow steady accumulation in the target area. Surfactant micelles, used as drug carriers in this situation, greatly enhance the therapeutic efficacy of medications. In addition, the present study will offer a unique promise and understanding to carry out a more rigorous study regarding how medications cross the cell membranes. The acquired knowledge from this study may be useful for investigating the research on interactions between a potential drug and a surfactant, developing better industrial formulations, drug delivery, and new drug development purposes in wider ranges of aq. salt/HDT media.
Conflicts of interest
There are no conflicts of interest to declare.
Acknowledgements
Authors are grateful to the Researchers Supporting Project number (RSP2023R360), King Saud University, Riyadh, Saudi Arabia.
References
- R. Sharma and R. K. Mahajan, RSC Adv., 2012, 2, 9571 RSC.
- X. Ji, C. Shi, L. Qi, Y. Guo, N. Li, Z. Li and Y. Luan, RSC Adv., 2014, 4, 62698 RSC.
- A. Z. Naqvi, S. Noori and Kabir-ud-Din, RSC Adv., 2016, 6, 20324 RSC.
- P. Khadka, J. Ro, H. Kim, I. Kima, J. Kima, H. Kima, J. Choa, G. Yunb and J. Leea, Asian J. Pharm. Sci., 2014, 9, 304 CrossRef.
- T. Hasan, S. Mahbub, D. Kumar, M. K. Gatasheh, M. T. R. Joy, M. A. Goni, S. Rana and M. A. Hoque, Mol. Phys., 2022, 120, e2121776 CrossRef.
- M. N. Islam, M. A. Rub, M. M. Alotaibi, M. T. R. Joy, I. Jahan, S. Mahbub, S. Rana, D. Kumar, M. Alfakeer, A. M. Asiri, M. A. Hoque and S. E. Kabir, Chem. Pap., 2023 DOI:10.1007/s11696-023-02856-7.
- M. N. Islam, M. A. Rub, M. K. Islam, M. A. Goni, S. Rana, D. Kumar, A. M. Asiri, Y. G. Alghamdi, M. A. Hoque and S. E. Kabir, J. Mol. Liq., 2023, 379, 121601 CrossRef.
- M. A. Rub, N. Azum and A. M. Asiri, J. Chem. Eng. Data, 2017, 62, 3216 CrossRef.
- D. Kumar and M. A. Rub, J. Mol. Liq., 2017, 238, 389 CrossRef CAS.
- M. A. Rub, T. Hasan, R. Akter, D. Kumar, Kabir-ud-Din, A. M. Asiri and M. A. Hoque, J. Mol. Liq., 2023, 371, 121070 CrossRef.
- V. Bhardwaj, T. Bhardwaj, K. Sharma, A. Gupta, S. Chauhan, S. S. Cameotra, S. Sharma, R. Guptad and P. Sharma, RSC Adv., 2014, 4, 24935 RSC.
- J. Bhattacharjee, G. Verma, V. K. Aswal, V. Patravale and P. A. Hassan, RSC Adv., 2013, 3, 23080 RSC.
- B. Yang, C. Wu, B. Ji, X. Ai, X. Kuang, M. Wu, M. Sun, C. Luo, Z. He and J. Sun, RSC Adv., 2015, 5, 104846 RSC.
- N. Gull, M. Ishtikhar, M. S. Alam, S. N. S. Andrabia and R. H. Khan, RSC Adv., 2017, 7, 8452 Search PubMed.
- R. Akter, K. M. Anis-Ul-Haque, M. A. Mottalib, D. Kumar, M. T. R. Joy, S. Rana, M. A. Hoque, T. M. Almutairi, A. A. A. Mohammedf and A. Iqbal, Mol. Phys., 2023, 121, e2148584 CrossRef.
- C. L. Liu, Y. J. Nikas and D. Blankschtein, Biotechnol. Bioeng., 1996, 52, 185 CrossRef CAS PubMed.
- D. Attwood and A. T. Florence, Surfactant Systems; their Chemistry, Pharmacy and Biology, Chapman & Hall, New York, 1983 Search PubMed.
- T. P. Niraula, S. N. Sah, A. Bhattarai, H. Dominguez, A. B. Salazar-Arriaga and D. Kumar, J. Mol. Liq., 2022, 367, 120339 CrossRef.
- S. Mahbub, M. A. Rub, M. A. Hoque, M. A. Khan and D. Kumar, J. Phys. Org. Chem., 2019, 32, e3967 CrossRef.
- A. Bhattarai, M. A. Rub, M. Posa, B. Saha, A. M. Asiri and D. Kumar, Colloids Surf., A, 2022, 655, 130334 CrossRef CAS.
- M. A. Hoque, M. I. Ali, M. A. Rub, M. Rahman, S. Rana, M. M. Rahman, D. Kumar, N. Azum, A. M. Asiri and M. A. Khan, Int. J. Biol. Macromol., 2023, 228, 445 CrossRef CAS PubMed.
- S. Das, S. Mondal and S. Ghosh, RSC Adv., 2016, 6, 30795 RSC.
- M. Akram, S. Anwar, F. Ansari, I. A. Bhat and Kabir-ud-Din, RSC Adv., 2016, 6, 21697 RSC.
- M. Akram, I. A. Bhat and Kabir-ud-Din, RSC Adv., 2015, 5, 102780 RSC.
- A. Bagheri and P. Khalili, RSC Adv., 2017, 7, 18151 RSC.
- M. Fresta, S. Guccione, A. R. Beccari, P. M. Furneri and G. Puglisi, Med. Chem., 2002, 10, 3871 CAS.
- J. N. Israelachvili, Intermolecular and Surface Forces, Academic Press, New York, 1995 Search PubMed.
- B. Lindman and H. Wennerström, Micelles: Topics in Current Chemistry, Springer, 1st edn, 1980 Search PubMed.
- C. J. Drummond and C. Fong, Curr. Opin. Colloid Interface Sci., 1999, 4, 449 CrossRef CAS.
- P. A. Bhat, G. M. Rather and A. A. Dar, J. Phys. Chem. B, 2009, 113, 997 CrossRef CAS PubMed.
- T. Chakraborty, I. Chakraborty and S. Ghosh, Langmuir, 2006, 22, 9905 CrossRef CAS PubMed.
- R. Liu, J. Yang, C. Sun, X. Wu, L. Li and B. Su, Colloids Surf., B, 2004, 34, 59 CrossRef CAS.
- K. Shinoda, J. Phys. Chem., 1954, 58, 1136 CrossRef CAS.
- V. K. Jadhav, B. A. Dixit and N. S. Tavare, J. Chem. Eng. Data, 1995, 40, 669 CrossRef CAS.
- R. M. Khalil, Pharmazie, 1997, 52, 866 CAS.
- H. O. Ammar and R. M. Khalil, Pharmazie, 1993, 48, 842 CAS.
- J. C. Eriksson and G. Gillberg, Acta Chem. Scand., 1966, 20, 2019 CrossRef CAS.
- P. M. Lindemuth and G. L. Bertrand, J. Phys. Chem., 1993, 97, 7769 CrossRef CAS.
- M. A. Rahim, S. Mahbub, S. M. A. Ahsan, M. Alam, M. Saha, I. Shahriar, S. Rana, M. A. Halim, M. A. Hoque, D. Kumar and J. M. Khan, J. Mol. Liq., 2021, 322, 114683 CrossRef CAS.
- M. Hossain, S. Mahbub, M. A. Rub, S. Rana, M. A. Hoque, D. Kumar, Y. G. Alghamdi and M. A. Khan, J. Mol. Liq., 2023, 376, 121431 CrossRef CAS.
- M. R. Hossain, M. A. Rub, S. Mahbub, S. Rana, A. M. Asiri, M. A. Hoque and M. Kabir, J. Mol. Liq., 2022, 367, 120372 CrossRef.
- R. Verma, A. Mishra and K. R. Mitchell-Koch, J. Chem. Theory Comput., 2015, 11, 5415–5425 CrossRef CAS PubMed.
- M. R. Amin, S. Mahbub, S. Hidayathulla, M. M. Alam, M. A. Hoque and M. A. Rub, J. Mol. Liq., 2018, 269, 417–425 CrossRef CAS.
- R. Amin, M. R. Molla, S. Rana, M. A. Hoque, M. A. Rub, M. Kabir and A. M. Asiri, Phys. Chem. Liq., 2019, 57, 703–719 CrossRef CAS.
- M. A. Hoque, M. M. Alam, M. R. Molla, S. Rana, M. A. Rub, M. A. Halim, M. A. Khan and A. Ahmed, J. Mol. Liq., 2017, 244, 512–520 CrossRef CAS.
- M. A. Hoque, M. R. Molla, M. R. Amin, M. M. Alam, M. F. Hossain and M. A. Rub, J. Sol. Chem., 2019, 48, 105–124 CrossRef CAS.
- Y. Lu, E. Zhang, J. Yang and Z. Cao, Nano Res., 2018, 11, 4985–4998 CrossRef PubMed.
- J. W. Yoo, D. J. Irvine, D. E. Discher and S. Mitragotri, Nat. Rev. Drug Discovery, 2011, 10, 521–535 CrossRef CAS PubMed.
- G. Verma and P. Hassan, Phys. Chem. Chem. Phys., 2013, 15, 17016–17028 RSC.
- V. S. Kulkarni and C. Shaw, Surfactants, lipids, and surface chemistry, in, Essential Chemistry for Formulators of Semisolid and Liquid Dosages, ed. V. S. Kulkarni and C. Shaw, Academic Press, Boston, 2016, ch. 2, pp. 5–19 Search PubMed.
- S. Kim, Y. Z. Shi, J. Y. Kim, K. Park and J.-X. Cheng, Expert Opin. Drug Delivery, 2010, 7, 49–62 CrossRef CAS PubMed.
- S. Paula, W. Siis, J. Tuchtenhagen and A. Blume, J. Phys. Chem., 1995, 99, 11742–11751 CrossRef CAS.
- Y. G. Alghamdi, M. A. Rub, D. Kumar and A. M. Asiri, R. Soc. Open Sci., 2021, 8, 211527 CrossRef CAS PubMed.
- M. A. Hoque, M. A. Rub, M. M. Rahman, M. A. Khan, D. Kumar and A. M. Asiri, J. Mol. Liq., 2021, 344, 117770 CrossRef.
- A. Dutta, M. T. R. Joy, S. M. A. Ahsan, M. K. Gatasheh, D. Kumar, M. A. Rub, M. A. Hoque, M. M. Rahman, N. Hoda and D. M. S. Islam, Chin. J. Chem. Eng., 2023, 57, 280–289 CrossRef.
- Y. G. Alghamdi, M. A. Rub and D. Kumar, R. Soc. Open Sci., 2023, 10, 221249 CrossRef CAS PubMed.
- F. Ahmed, M. R. Molla, M. Saha, I. Shahriar, M. S. Rahman, M. A. Halim, M. A. Rub, M. A. Hoque and A. M. Asiri, RSC Adv., 2019, 9, 6556 RSC.
- J. M. Goronja, A. M. J. Lezaic, B. M. Dimitrijevic, A. M. Malenovic, D. R. Stanisavljev and N. D. Pejic, Hem. Ind., 2016, 70, 485 CrossRef.
- A. Gonzalez-Perez, J. Czapkiewicz, G. Prieto and J. R. Rodrıguez, Colloid Polym. Sci., 2003, 281, 1191 CrossRef CAS.
- M. Perez-Rodrıguez, G. Prieto, C. Rega, L. M. Varela, F. Sarmiento and V. Mosquera, Langmuir, 1998, 14, 4422 CrossRef.
- S. Dai and K. C. Tam, J. Phys. Chem. B, 2001, 105, 10759 CrossRef CAS.
- S. Rather, S. Islam, H. S. Bamufleh, H. Alhumade, A. A. Taimoor, U. Saeed, A. A. Sulaimon, M. A. Hoque, W. M. Alalayah and A. M. Shariff, J. Mol. Liq., 2023, 380, 121722 CrossRef CAS.
- M. A. Hoque, M. M. Rahman, M. M. Alam, S. Mahbub, M. A. Khan, D. Kumar, M. D. Albaqami and S. M. Wabaidur, J. Mol. Liq., 2021, 326, 115337 CrossRef CAS.
- M. J. Rosen, Surfactants and Interfacial Phenomena, Wiley, New York, 3rd edn, 2004 Search PubMed.
- J. Mata, D. Varade and P. Bahadur, Thermochim. Acta, 2005, 428, 147 CrossRef CAS.
- L. Martínez, R. Andrade, E. G. Birgin and J. M. Martínez, J. Comput. Chem., 2009, 30, 2157–2164 CrossRef PubMed.
- S. Mahbub, M. R. Molla, M. Saha, I. Shahriar, M. A. Hoque, M. A. Halim, M. A. Rub and M. A. Khan, J. Mol. Liq., 2019, 283, 263 CrossRef CAS.
- M. S. Alam, V. Nareshkumar, N. Vijayakumar, K. Madhavan and A. B. Mandal, J. Mol. Liq., 2014, 194, 206 CrossRef CAS.
- D. Bhatt, K. C. Maheria and J. Parikh, J. Surfactants Deterg., 2013, 16, 547 CrossRef CAS.
- H. Kumar, A. Katal and N. K. Rawat, Z. Phys. Chem., 2020, 234, 1603 CrossRef CAS.
- M. A. Hamdiyyah, J. Phys. Chem., 1965, 69, 2720 CrossRef.
- C. K. Bahal and H. B. Kostenbauder, J. Pharm. Sci., 1964, 53, 1027 CrossRef CAS PubMed.
- J. J. H. Nusselder and J. B. Engberts, J. Colloid Interface Sci., 1992, 148, 353 CrossRef CAS.
- A. K. Sood and S. Sharma, Phys. Chem. Liq., 2016, 54, 574 CrossRef CAS.
- S. Das, B. Naskar and S. Ghosh, Soft Matter, 2014, 10, 2863 RSC.
- T. Hasan, M. A. Rub, M. T. R. Joy, S. Rana, F. Khan, M. A. Hoque and M. Kabir, J. Mol. Liq., 2022, 354, 118853 CrossRef CAS.
- R. Lumry and S. Rajender, Biopolym, 1970, 9, 1125 CrossRef CAS PubMed.
- C. Jolicoeur and P. R. Philip, Can. J. Chem., 1974, 52, 1834 CrossRef CAS.
- M. R. Amin, M. A. Rub, A. H. Shah, D. Kumar, M. M. Rahman, M. A. Hoque, M. Kabir, A. M. Asiri and S. E. Kabir, J. Mol. Liq., 2022, 339, 119325 CrossRef.
- M. A. Rub, M. A. Hoque, N. Azum and S. Mahbub, J. Mol. Liq., 2021, 346, 117109 Search PubMed.
- S. Kumar and K. Parikh, J. Appl. Solution Chem. Model., 2012, 1, 65 CAS.
- S. M. A. Ahsan, S. Mahbub, M. R. Amin, J. M. Khan, M. A. Hoque, A. Malik, A. Ahmed, M. Z. Ahmed and M. K. Anwer, J. Mol. Liq., 2021, 342, 116953 CrossRef.
- M. Rahman, S. J. Anwar, M. R. Molla, S. Rana, M. A. Hoque, M. A. Rub, M. A. Khan and D. Kumar, J. Mol. Liq., 2019, 292, 111322 CrossRef CAS.
|
This journal is © The Royal Society of Chemistry 2023 |