DOI:
10.1039/D3RA02267F
(Paper)
RSC Adv., 2023,
13, 15942-15946
Synthesis of 4-aryl-3,4-dihydrocoumarins and 4-aryl-4H-chromenes via Er(OTf)3-catalyzed cascade reactions of p-quinone methides with 1,3-dicarbonyl compounds†
Received
5th April 2023
, Accepted 23rd May 2023
First published on 26th May 2023
Abstract
The Er(OTf)3-catalyzed cascade cyclization reaction of para-quinone methides (p-QMs) with various 1,3-dicarbonyl compounds has been developed, which efficiently constructed a series of versatile 4-aryl-3,4-dihydrocoumarins and 4-aryl-4H-chromenes. Herein, we not only propose a novel cyclization strategy of p-QMs, but also provide an easy access to structurally diverse coumarins and chromenes.
Introduction
4-Aryl-3,4-dihydrocoumarin and 4-aryl-4H-chromene are important structural motifs that broadly occur in many natural products and biologically active compounds.1 Molecules containing the 4-aryl-3,4-dihydrocoumarin or 4-aryl-4H-chromene scaffold exhibit noteworthy pharmacological effects. For example, compound 1 isolated from Aloe vera displays remarkable antioxidative and immunomodulatory properties.2 Compound 2 is a synthetic analog of podophyllotoxin and exhibits interesting antimitotic activity.3 Compound 3, a specific inhibitor of insulin-regulated aminopeptidase, has potential ability in the enhancement of cognitive functions.4 Compound 4, also called crolibulin, is a tubulin polymerization inhibitor with potent apoptosis induction and cell growth inhibition, which has progressed to phase I/II clinical trial for anaplastic thyroid cancer.5 Due to their wide biological applications, significant efforts have been devoted to the synthesis of 4-aryl-3,4-dihydrocoumarin and 4-aryl-4H-chromene derivatives. The conventional synthetic methods for 4-aryl-3,4-dihydrocoumarins involve (i) catalytic hydrogenation of 4-aryl-coumarins,6 (ii) hydroarylation of cinnamic acids with phenols,7 (iii) annulation reaction of phenols with Meldrum's acid derivatives,8 (iv) lactonization of 3-aryl-3-(2-hydroxyphenyl)propanoates,9 (v) asymmetric formal [4 + 2] cyclization of o-quinone methides (o-QMs) with various two-carbon reaction partners,10 and (vi) multicomponent reaction between phenols, malonates and aryl aldehydes.11 Meanwhile, several facile methodologies have been developed for the synthesis of 4-aryl-4H-chromenes including the electrophilic substitution of aromatic compounds with 4-methylsulfanyl-4H-chromenes,12 dehydration of the 1,4-adducts between arylboronic acids and β-(2-hydroxyaryl)-enones,13 tandem benzylation–cyclization of 2-(hydroxymethyl)phenols with 1,3-dicarbonyl compounds,14 domino Michael addition–cyclization of 2-hydroxychalcone with indoles,15 and multicomponent reaction between phenols, aryl aldehydes and malononitrile.16 To our knowledge, however, all of these approaches are hardly realized the simultaneous synthesis of 4-aryl-3,4-dihydrocoumarins and 4-aryl-4H-chromenes under current conditions (Fig. 1).
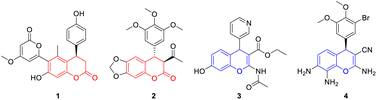 |
| Fig. 1 Selected bioactive 4-aryl-3,4-dihydrocoumarins and 4-aryl-4H-chromenes. | |
In recent years, the application of p-QMs in the construction of oxygen/nitrogen-containing heterocyclic frameworks has attracted much attention in the synthetic community. Especially, Fan and coworkers described an efficient synthesis of 4-aryl-3,4-dihydrocoumarins through an intramolecular vinylogous Rauhut–Currier reaction of ortho-acryloyloxyphenyl-substituted p-QMs (Scheme 1, eqn (1)).17 Shi18a and Hu18b reported a [4 + 2] cyclization of o-hydroxyphenyl-substituted p-QMs with alkynes, which provided an efficient method for the construction of 4-aryl-4H-chromene scaffold (Scheme 1, eqn (2)). In addition, two reports involving an organocatalytic domino reaction of o-hydroxyphenyl-substituted p-QMs with β-functionalized ketones/nitriles have been presented for the synthesis of chiral 4-aryl-4H-chromenes.18c,d Very recently, our group developed an efficient synthetic approach to 7-aminocoumarins via Er(OTf)3-catalyzed Pechmann condensation of 3-aminophenols with β-ketoesters.19 As we know, Er(OTf)3 as a very gentle Lewis acid catalyst can effectively activate carbonyl, epoxide and alkene compounds even in the presence of trace amount of water.20 1,3-Dicarbonyl compounds have been widely used as versatile and highly active building blocks in organic synthesis because of their intrinsic reactivities.21 Based on these discoveries, we envisioned that the simultaneous synthesis of 4-aryl-3,4-dihydrocoumarin and 4-aryl-4H-chromene could be realized through the union of p-QMs and different 1,3-dicarbonyl compounds via a Er(OTf)3-catalyzed cascade reaction (Scheme 1, eqn (3)).
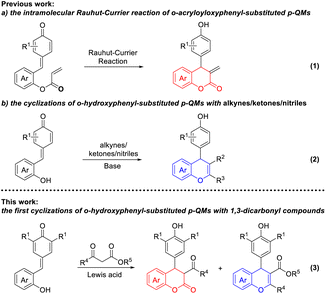 |
| Scheme 1 Cyclization strategies for the synthesis of 4-aryl-3,4-dihydrocoumarins and 4-aryl-4H-chromenes from p-QMs. | |
Results and discussion
To verify our hypothesis, o-hydroxyphenyl-substituted p-QM 5a and β-ketoester 6a were employed as model substrates for the optimization of the cascade reaction conditions; the results are presented in Table 1. The screening of solvent was carried out first because the use of a suitable solvent is crucial for Lewis acid-catalyzed reaction. Notably, when toluene was used at reflux temperature for 8 h, the expected 4-aryl-3,4-dihydrocoumarin 7a and 4-aryl-4H-chromene 8a could be obtained in 62% and 22% yields, respectively (entry 4). However, DCE, ACN and dioxane with low boiling point were inefficient for the generation of 8a, only affording the desired product 7a in low yields (10–38%) even with extended reaction times (entries 1–3). The polar solvent with high boiling point, like DMSO and DMF, directly prevented this transformation due to the coordination of these solvents with the Lewis acid catalysts (entries 5 and 6). Next, our attention turned to investigate the effect of reaction time and temperature on the formation of 7a and 8a (entries 7–11). After several attempts, we found that prolonging the reaction time was beneficial to improve the yield and 12 h was the appropriate reaction time. The reaction temperature was the key factor for the formation of 8a. When the reaction proceeded at 70 °C, the expected 7a was produced in 52% yield without the generation of 8a (entry 10). At 90 °C, we obtained 7a in 71% yield and 8a in 11% yield (entry 11). In addition, a set of lanthanide triflates were investigated for this reaction under the same reaction conditions. Compared with Er(OTf)3, other lanthanide salts such as Sc(OTf)3, Y(OTf)3, La(OTf)3, Ce(OTf)3 and Yb(OTf)3 provided lower total yields of 7a and 8a (entries 12–16). However, these explorations are difficult to achieve product specificity. Finally, the optimal reaction conditions for the simultaneous synthesis of 7a and 8a are selected as those shown in entry 7 (1.0 equiv. of p-QM 5a, 1.1 equiv. of β-ketoester 6a, 0.2 equiv. of Er(OTf)3 as catalyst in toluene at 110 °C for 12 h).
Table 1 Investigation of conditions for lanthanide triflate-catalyzed synthesis of 4-aryl-3,4-dihydrocoumarin 7a and 4-aryl-4H-chromene 8aa
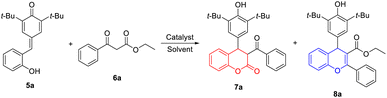
|
Entry |
Catalyst |
Solvent |
Temp. (°C) |
T (h) |
Yield of 7ab (%) |
Yield of 8ab (%) |
Reaction conditions: 5a (1.0 mmol), 6a (1.1 mmol), salt (20 mol%), solvent (3 mL). Isolated yield. |
1 |
Er(OTf)3 |
DCE |
84 |
24 |
38 |
0 |
2 |
Er(OTf)3 |
ACN |
82 |
24 |
33 |
0 |
3 |
Er(OTf)3 |
Dioxane |
100 |
24 |
10 |
0 |
4 |
Er(OTf)3 |
Toluene |
110 |
8 |
62 |
22 |
5 |
Er(OTf)3 |
DMF |
120 |
12 |
0 |
0 |
6 |
Er(OTf)3 |
DMSO |
140 |
12 |
0 |
0 |
7 |
Er(OTf)3 |
Toluene |
110 |
12 |
68 |
22 |
8 |
Er(OTf)3 |
Toluene |
110 |
24 |
64 |
23 |
9 |
Er(OTf)3 |
Toluene |
110 |
48 |
61 |
24 |
10 |
Er(OTf)3 |
Toluene |
70 |
24 |
52 |
0 |
11 |
Er(OTf)3 |
Toluene |
90 |
24 |
71 |
11 |
12 |
Sc(OTf)3 |
Toluene |
110 |
12 |
28 |
37 |
13 |
Y(OTf)3 |
Toluene |
110 |
12 |
21 |
34 |
14 |
La(OTf)3 |
Toluene |
110 |
12 |
25 |
44 |
15 |
Ce(OTf)3 |
Toluene |
110 |
12 |
35 |
10 |
16 |
Yb(OTf)3 |
Toluene |
110 |
12 |
24 |
23 |
With the optimal reaction conditions in hand, the generality of Er(OTf)3-catalyzed cyclization between p-QMs 5 and β-ketoesters 6 was investigated. As shown in Table 2, a wide range of β-ketoesters 6 could readily react with p-QM 5a (R4 = H), which simultaneously afforded the corresponding 4-aryl-3,4-dihydrocoumarins 7b–7m and 4-aryl-4H-chromenes 8b–8m in good overall yields. In detail, β-ketoesters 6 bearing alkane groups (R4 = Me, Et, i-Pr, cyclopropyl, cyclobutyl and cyclopentyl) mainly provided 8b–8g in moderate to good yields (41–75%), with 7b–7g in low yields (14–37%). By contrast, when R4 was cyclohexyl group, the corresponding 4-aryl-3,4-dihydrocoumarin 7h was obtained in good yield (63%), together with 4-aryl-4H-chromenes 8h in low yield (25%). Encouraged by the above results, we further explored the effects of (heter)aryl groups on the product yields. Interestingly, β-ketoester 6 with a strong electron-donating group on the benzene ring gave the corresponding product 8j (R4 = 4-methoxyphenyl) in good yield (60%); however, 8a (R4 = phenyl), 8i (R4 = 4-methylphenyl) and 8k (R4 = 4-chlorophenyl) were produced in low yields (19–29%) under the same reaction conditions. When R4 were heteraryl groups, the corresponding 4-aryl-3,4-dihydrocoumarins 7l (R4 = 2-furanyl) and 7m (R4 = 2-thiophenyl) were the main products in 59–62% yields. However, the substituents on the benzene ring of p-QMs 5 could affect the proportion of products, and the corresponding products 7n–7o and 8n–8o were obtained in 52–55% and 26–39% yields, compared to 7j and 8j. Moreover, NMR data displayed 7b, 7c, 7e, 7f with enol form, and 7d, 7g–7o with keto form. The structure of 8h was determined by single-crystal X-ray diffraction analyses.22
Table 2 Er(OTf)3-catalyzed simultaneous synthesis of 4-aryl-3,4-dihydrocoumarin 7 and 4-aryl-4H-chromene 8a,b
Reaction conditions: 5 (1.0 mmol), 6 (1.1 mmol) and Er(OTf)3 (20 mol%) in toluene (3 mL) at 110 °C for 12 h. Isolated yield. |
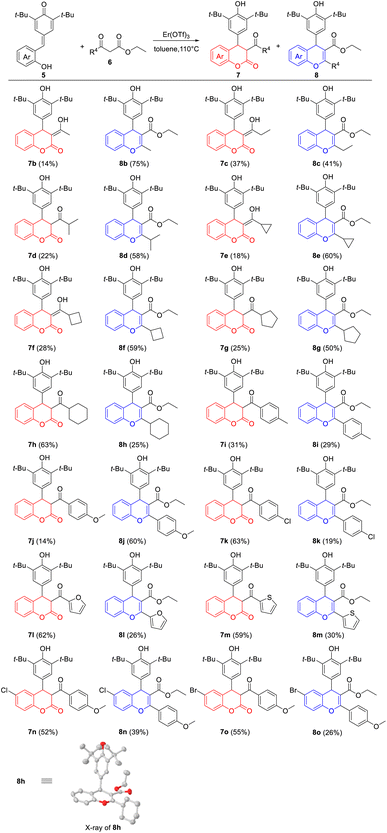 |
Subsequently, to realize the exclusive synthesis of 4-aryl-3,4-dihydrocoumarins, we extended this protocol to various malonates 9 under the standard conditions (Table 3). To our delight, a series of malonates could be employed to the reaction with p-QMs 5, which delivered functionalized 4-aryl-3,4-dihydrocoumarins 10a–10j in generally acceptable to good yields (up to 90%). It was found that the stability of malonate esters could affect the reaction efficiency in this intramolecular transesterification–cyclization process, and the corresponding products 10c (R5 = i-Pr) and 10d (R5 = benzyl) were formed in 42–54% yield. The electronic properties of substituents on the benzene ring of p-QMs 5 almost had no influence on the efficiency of this reaction; 10e–10i were obtained in 82–88% yield. Interestingly, however, the presence of strong electron-withdrawing nitro group promoted the hydrolysis and decarboxylation at the 3-position under the standard conditions, giving 10j in 90% yield. Moreover, the structure of 10c, which was a pair of enantiomers, was further assigned by X-ray crystallographic analysis.22
Table 3 Er(OTf)3-catalyzed exclusive synthesis of 4-aryl-3,4-dihydrocoumarin 10a,b
Reaction conditions: 5 (1.0 mmol), 9 (1.1 mmol) and Er(OTf)3 (20 mol%) in toluene (3 mL) at 110 °C for 12 h. Isolated yield. |
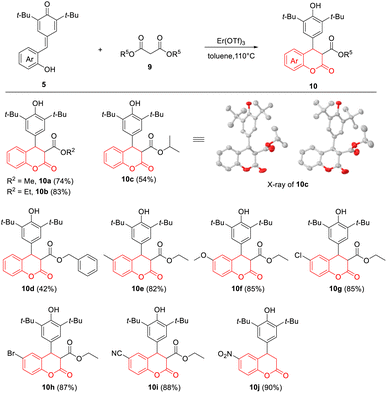 |
Furthermore, to verify whether it is suitable for the exclusive synthesis of 4-aryl-4H-chromenes, this protocol was extended to various β-diketones 11 under similar conditions (Table 4). We were pleased to find that the reaction of p-QM 5a with 2,4-pentanedione occurred readily to afford the desired 4-aryl-4H-chromene 12a in 39% yield. Significantly, a variety of 1-(hetero)aryl-1,3-butanediones reacted with p-QMs 5 smoothly to give the corresponding 2-(hetero)aryl-3-acetyl-4-aryl-4H-chromenes 12b–12j in 38–60% yields, without the generation of 2-acetyl-3-(hetero)aryl-4-aryl-4H-chromenes. This result was attributed to intramolecular π-conjugated effects and steric hindrance effects. To further check the substrate scope of this reaction, cyclic 1,3-diketones were taken under consideration, which provided products 12k and 12l in good yields (77–82%).
Table 4 Er(OTf)3-catalyzed exclusive synthesis of 4-aryl-4H-chromene 12a,b
Reaction conditions: 5 (1.0 mmol), 11 (1.1 mmol) and Er(OTf)3 (20 mol%) in toluene (3 mL) at 110 °C for 12 h. Isolated yield. |
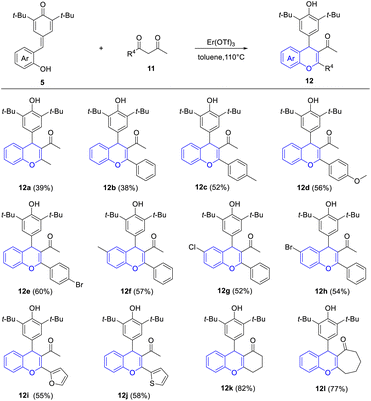 |
On the basis of the experimental results, a plausible mechanism was suggested (Scheme 2). As exemplified by the formation of products 7a and 8a, in the presence of Er(OTf)3, the whole cascade reaction was initiated by the 1,6-addition reaction of p-QM 5a and 1,3-dicarbonyl compound 6a, which produced the key intermediate 1,6-adduct I possessing two reactive sites. Then, intermediate I underwent an intramolecular transesterification between phenolic hydroxyl group and carboxylate group to accomplish the cyclization, which afforded 4-aryl-3,4-dihydrocoumarin 7a (Scheme 2, path a). Meanwhile, the intramolecular nucleophilic attack of phenolic hydroxyl group at the keto carbonyl group completed the cyclization of intermediate I to form 4-aryl-4H-chromene 8a with elimination of a water molecule (Scheme 2, path b).
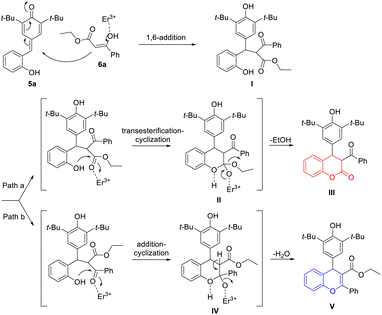 |
| Scheme 2 Plausible reaction path for the formation of 4-aryl-3,4-dihydrocoumarin and 4-aryl-4H-chromene. | |
Conclusions
In summary, we have established the first cyclization of para-quinone methide derivatives with 1,3-dicarbonyl compounds by utilizing Er(OTf)3-catalyzed cascade reaction, which efficiently constructed the scaffolds of 4-aryl-3,4-dihydrocoumarin and 4-aryl-4H-chromene. This protocol could not only fulfill the task of developing new cyclization strategies of p-QMs, but also provide an easy access to structurally diverse coumarins and chromenes. The further applications of Er(OTf)3-catalyzed cascade transformations of p-QMs are ongoing in our laboratory.
Author contributions
DC, XB, and WY conceived the idea and designed the research. XB and WY performed the research. LW, XD, and GW analyzed the data. DC, XB, and WY wrote the original manuscript. WC and FL reviewed the manuscript and suggested improvements. All authors have read and agreed to the published version of the manuscript.
Conflicts of interest
There are no conflicts to declare.
Acknowledgements
This work was supported by the Advanced Training Program for Teachers as Professional Leaders in Higher Vocational Colleges in Jiangsu Province (No. 2021GRFX031) and the National Natural Science Foundation of China (No. 82171224, 82103981).
Notes and references
- V. Raj and J. Lee, Front. Chem., 2020, 8, 623 CrossRef CAS PubMed.
- X. F. Zhang, H. M. Wang, Y. L. Song, L. H. Nie, L. F. Wang, B. Liu, P. P. Shen and Y. Liu, Bioorg. Med. Chem. Lett., 2006, 16, 949 CrossRef CAS PubMed.
- J. K. Batra, G. J. Kang, L. Jurd and E. Hamel, Biochem. Pharmacol., 1988, 37, 2595 CrossRef CAS PubMed.
- A. L. Albiston, S. Diwakarla, R. N. Fernando, S. J. Mountford, H. R. Yeatman, B. Morgan, V. Pham, J. K. Holien, M. W. Parker, P. E. Thompson and S. Y. Chai, Br. J. Pharmacol., 2011, 164, 37 CrossRef CAS PubMed.
- A. M. A. Lorza, H. Ravi, R. C. Philip, J. P. Galons, T. P. Trouard, N. A. Parra, D. D. Von Hoff, W. L. Read, R. Tibes, R. L. Korn and N. Raghunand, Sci. Rep., 2020, 10, 14449 CrossRef CAS PubMed.
- M. A. McGuire, S. C. Shilcrat and E. Sorenson, Tetrahedron Lett., 1999, 40, 3293 CrossRef CAS.
- K. Li, L. N. Foresee and J. A. Tunge, J. Org. Chem., 2005, 70, 2881 CrossRef CAS PubMed.
- E. Fillion, A. M. Dumas, B. A. Kuropatwa, N. R. Malhotra and T. C. Sitler, J. Org. Chem., 2006, 71, 409 CrossRef CAS PubMed.
-
(a) J. Barluenga, F. Andina and F. Aznar, Org. Lett., 2006, 8, 2703 CrossRef CAS PubMed;
(b) C. Chen, R. Zhang, L. Lin, G. F. Yang and Q. Y. Wu, Tetrahedron, 2016, 72, 3917 CrossRef CAS.
-
(a) L. Cui, D. Lv, Y. Wang, Z. Fan, Z. Li and Z. Zhou, J. Org. Chem., 2018, 83, 4221 CrossRef CAS PubMed;
(b) T. Zhang, C. Ma, J. Zhou, G. J. Mei and F. Shi, Adv. Synth. Catal., 2018, 360, 1128 CrossRef CAS;
(c) C. Gharui, C. Parida and S. C. Pan, J. Org. Chem., 2021, 86, 13071 CrossRef CAS PubMed.
- W. H. Dos Santos and L. C. Da Silva-Filho, Synthesis, 2012, 44, 3361 CrossRef CAS.
-
(a) A. Parthiban, M. Kumaravel, J. Muthukumaran, R. Rukkumani, R. Krishna and H. S. P. Rao, Med. Chem. Res., 2016, 25, 1308 CrossRef CAS;
(b) H. S. Rao and M. Kamalraj, Nat. Prod. Commun., 2014, 9, 1333 CrossRef CAS PubMed.
- T. Nishikata, Y. Yamamoto and N. Miyaura, Adv. Synth. Catal., 2007, 349, 1759 CrossRef CAS.
-
(a) J. Fan and Z. Wang, Chem. Commun., 2008, 5381 RSC;
(b) T. Aoyama, T. Yamamoto, S. Miyota, M. Hayakawa, T. Takido and M. Kodomari, Synlett, 2014, 25, 1571 CrossRef;
(c) S. Yaragorla, P. L. Saini, P. V. Babu, A. I. Almansour and N. Arumugam, Tetrahedron Lett., 2016, 57, 2351 CrossRef CAS.
- G. Yin, L. Fan, T. Ren, C. Zheng, Q. Tao, A. Wu and N. She, Org. Biomol. Chem., 2012, 10, 8877 RSC.
- W. Kemnitzer, J. Drewe, S. Jiang, H. Zhang, J. Zhao, C. Crogan-Grundy, L. Xu, S. Lamothe, H. Gourdeau, R. Denis, B. Tseng, S. Kasibhatla and S. X. Cai, J. Med. Chem., 2007, 50, 2858 CrossRef CAS PubMed.
- X. Z. Zhang, K. J. Gan, X. X. Liu, Y. H. Deng, F. X. Wang, K. Y. Yu, J. Zhang and C. A. Fan, Org. Lett., 2017, 9, 3207 CrossRef PubMed.
-
(a) G. J. Mei, S. L. Xu, W. Q. Zheng, C. Y. Bian and F. Shi, J. Org. Chem., 2018, 83, 1414 CrossRef CAS PubMed;
(b) J. Wang, Q. Rong, L. Zhao, X. Pan, L. Zhao, K. Zhao and L. Hu, J. Org. Chem., 2020, 85, 11240 CrossRef CAS PubMed;
(c) L. Zhang, X. Zhou, P. Li, Z. Liu, Y. Liu, Y. Sun and W. Li, RSC Adv., 2017, 7, 39216 RSC;
(d) C. Duan, L. Ye, W. Xu, X. Li, F. Chen, Z. Zhao and X. Li, Chin. Chem. Lett., 2018, 29, 1273 CrossRef CAS.
- X. Bao, G. Wang, C. Tian, X. Dong, G. Xu, F. Li and D. Chen, Tetrahedron, 2022, 123, 132994 CrossRef CAS.
- For selected recent examples, see:
(a) L. Maiuolo, B. Russo, V. Algieri, M. Nardi, M. L. Di Gioia, M. A. Tallarida and A. De Nino, Tetrahedron Lett., 2019, 60, 672 CrossRef CAS;
(b) X. Liu, L. Longwitz, B. Spiegelberg, J. Tonjes, T. Beweries and T. Werner, ACS Catal., 2020, 10, 13659 CrossRef CAS;
(c) Y. W. Liu, R. J. Ma, Q. E. Wang, C. M. Si and B. G. Wei, Tetrahedron, 2020, 76, 131649 CrossRef CAS;
(d) S. S. Hosseini, A. Abdi, A. Nikbakht, H. R. Bijanzadeh, F. Rominger, D. B. Werz and S. Balalaie, Synlett, 2022, 33, 468 CrossRef CAS.
- For selected recent examples, see:
(a) X. Xu, Y. Shi, D. Wang, Y. Ding, S. Chen and X. Zhang, J. Org. Chem., 2022, 87, 14352 CrossRef CAS PubMed;
(b) S. Sunagawa, F. Morisaki, T. Baba, A. Tsubouchi, A. Yoshimura, K. Miyamoto, M. Uchiyama and A. Saito, Org. Lett., 2022, 24, 5230 CrossRef CAS PubMed;
(c) T. Cai, Z. Zhang, P. Li, T. Sun, X. Chen, Y. Ni, J. Chen, H. Xu, Y. Xu, C. Wu, R. Shen and Y. Gao, Adv. Synth. Catal., 2021, 363, 3750 CrossRef CAS;
(d) M. C. Sau and M. Bhattacharjee, RSC Adv., 2020, 10, 36014 RSC.
- CCDC 2248199 (8h) and 2246898 (10c) contain the supplementary crystallographic data (ESI†).
Footnotes |
† Electronic supplementary information (ESI) available. CCDC 2246898 and 2248199. For ESI and crystallographic data in CIF or other electronic format see DOI: https://doi.org/10.1039/d3ra02267f |
‡ These authors contributed equally to this work. |
|
This journal is © The Royal Society of Chemistry 2023 |
Click here to see how this site uses Cookies. View our privacy policy here.