DOI:
10.1039/D3RA02169F
(Review Article)
RSC Adv., 2023,
13, 15566-15574
Sources, distribution, and environmental effects of microplastics: a systematic review
Received
2nd April 2023
, Accepted 18th May 2023
First published on 23rd May 2023
Abstract
Microplastics (MPs) are receiving increasing attention from researchers. They are environmental pollutants that do not degrade easily, are retained for prolonged periods in environmental media such as water and sediments, and are known to accumulate in aquatic organisms. The aim of this review is to show and discuss the transport and effects of microplastics in the environment. We systematically and critically review 91 articles in the field of sources, distribution, and environmental behavior of microplastics. We conclude that the spread of plastic pollution is related to a myriad of processes and that both primary and secondary MPs are prevalent in the environment. Rivers have been indicated as major pathways for the transport of MPs from terrestrial areas into the ocean, and atmospheric circulation may be an important avenue for transporting MPs between environmental compartments. Additionally, the vector effect of MPs can change the original environmental behavior of other pollutants, leading to severe compound toxicity. Further in-depth studies on the distribution and chemical and biological interactions of MPs are highly suggested to improve our understanding of how MPs behave in the environment.
1 Introduction
Plastics are polymers composed of monomer molecules in long chains.1 Owing to their durability and low production costs, they are widely used in construction, transportation, agriculture, packaging, and production of personal and household goods.2 Although few countries have achieved high plastic recycling rates, numerous developing countries have no legal requirements for recycling plastic waste. A significant amount of the current immense volume of plastic ends up as non-recycled waste.3 The surge in the production and use of single-use plastic protective equipment during the Covid-19 pandemic has resulted in a large volume of additional plastic waste entering the environment.4 Millions to tens of millions of tons of plastic waste enter the ocean every year.5 Lakes and rivers, which are major pathways for plastic entering the ocean, have become potential reservoirs of plastic pollution.6 Given the ever-increasing use of plastic, we cannot afford to turn a blind eye to plastic waste in the environment.7
Plastic microbeads were first discovered in the 1970s, and the concept of “microplastics” was first proposed by Thompson et al.8 In subsequent decades, the threat posed by microplastics (MPs) because of their small size, large number, absorption of pollutants, and ingestion by organisms has become apparent to both the scientific community and the wider public.9,10 Several studies have defined MPs as plastic particles with a diameter of less than 5 mm.11 MPs do not always exist in the form of dispersed particles after being released to aquatic environments, as aggregation occurs under the action of water currents or organisms.12,13 Aggregation can be either homogeneous, between particles of the same type; or heterogeneous, between different particles.14 MPs are also particularly diverse in terms of size (nanometers to microns) and properties (shape as well as physical and chemical properties), and each polymer possesses its own unique grouping structure, such as amide bonds in PA, halogen bonds in PVC, nitrile groups in ABS, and benzene rings in PS. Because of the diversity and prevalence of plastic particles in natural systems, other pollutants interact with them in various ways.15 Although studies have indicated that plastic pollution is prevalent in the environment, few studies have systematically summarized the occurrence and behavior of MPs in the environment. We still lack the data and knowledge to link sources, transportation pathways, fates, and ecological impacts of plastic fragments in the environment, but these are needed if we are to assess the extent of plastic pollution in aquatic ecosystems and formulate effective prevention strategies. To gain a better understanding of the distribution and fates of MPs, we conducted a systematic review of recent research on the distribution and transportation of MPs in various environmental compartments and discussed the behavior of MPs in the environment as well as their potential effects on organisms.
2 Sources, classification and analysis of microplastics
Some MPs are produced directly in microscopic size in the process of artificial production. One example is microbeads, which are added to facial cleansers, body washes, hand sanitizer, toothpaste and sunscreen. Such particles are able to pass through the screens at wastewater treatment plants and are eventually released into the environment. These MPs are called primary MPs. In contrast, MPs formed by the decomposition of large-sized plastic products under the influence of light, weathering, waves and organisms, are called secondary MPs.16 A study across six continents has shown that most MPs in the marine environment are secondary MPs.17 The most common MPs in the environment are polypropylene, polyethylene, polystyrene, and polyvinyl chloride. Table 1 lists physical properties and sources of common MPs.18
Table 1 Types, properties and sources of common microplastic waste constituents
Plastic class |
|
Density (g cm−1) |
Products and typical origin |
High-density polyethylene |
HDPE |
0.96 |
Toys, solution containers, tubing |
Low-density polyethylene |
LDPE |
0.92 |
Bags, plastic wrap, containers |
Polypropylene |
PP |
0.90 |
Food packaging, pipes, microwavable lunch box |
Polyvinyl chloride |
PVC |
1.40 |
Floor panels, piping, cable enclosures |
Polystyrene |
PS |
1.02–1.05 |
Foamed foam, insulation material |
Polyethylene terephthalate |
PET |
1.55 |
Water bottles, fabric |
Polyamides |
PA |
1.02–1.14 |
Adhesive, fabric |
Polymethylmethacrylate |
PMMA |
1.18 |
Plates, plexiglas |
Polycarbonate |
PC |
1.36 |
Insulators, medical tubes, instrument casings |
Polyurethane |
PU |
1.01∼1.03 |
Artificial leather, foam, adhesive |
Primary MPs such as microbeads tend to be fairly uniform in appearance and have smooth surfaces. Secondary MPs tend to have a more random appearance, often with uneven surfaces and obvious traces of weathering or erosion. Laboratory studies have clearly shown that MPs may be toxic for organisms, and the size and shape of MPs are important factors influencing toxicity.19 Weathering and degradation breaks down and decomposes MPs, and the continuous reduction in particle size will increase their environmental concentration, which is conducive to their migration through the environment and enrichment in organisms. In addition, the distribution of MPs in the environment is influenced by their density. Generally speaking, the low density of PE and PP MPs allows them to float on the surface of the water. Because PVC, PS, PET and PA MPs have greater density than water, they readily settle and enter the sediment.19 However, some studies have also found a large number of MPs with a density lower than water in river surface sediments.20 The density of MPs is not an immutable characteristic, and weathering, degradation, surface wear and other effects may lead to substantial changes in density.21 Because most MPs have a high specific surface area (SSA), they easily accumulate biomass in natural environments or form heterogeneous aggregates with organic debris and fragments; this may also cause changes in their density and buoyancy, and thus lead to migration into different environments.14
The diversity of MPs and their ubiquity in different environmental media pose a challenge to their accurate identification. A variety of identification and analysis methods have been developed to address this problem. Optical microscopy is the most convenient and practical MPs identification technology and is often used for observation and initial identification before further analysis. However, there are often subjective factors in the process of visual identification, and issues such as possibly insufficient microscope resolution, background interference, and deformation of MPs may lead to unreliable identification results. Various forms of microscopy other than visible light can produce better results. The most important of these is the scanning electron microscope, which can accurately identify the size and morphology of MPs with little damage to the original sample and achieve image resolutions far higher than possible with optical microscopes, thus providing details of the microstructure of MPs.22 Fourier transform infrared (FTIR) spectroscopy is the most widely used technology in the further analysis and identification of MPs, being direct, reliable and non-destructive. FTIR can not only accurately identify the polymer type of MPs, but also provide information about physicochemical weathering by analyzing MP oxidation strength.23 Raman spectroscopy is considered a complement to FTIR. It features higher spatial resolution for very small samples, can provide structural and chemical characteristics of samples smaller than 1 μm, and is highly sensitive to non-polar functional groups.24 Pyrolytic-gas chromatography-mass spectrometry and nuclear magnetic resonance spectroscopy have also been used to analyze and identify MPs in some studies.25 However, because these methods are destructive to samples, time-consuming to operate, have a low detection ratio, and suffer from some other drawbacks, their utilization rate is far lower than that of the other methods noted.
3 Microplastics in different environmental media
3.1 Microplastics in the marine environment
The density of most plastics is close to that of water, which is why plastic particles float or are suspended in water and move with the current. One study estimated that 60–80% of marine litter is plastic.26 High concentrations of plastic particles have been found in coastal areas, semi-enclosed seas, and gyres.27 The study by Carson et al.28 showed that the concentration of MPs in the North Pacific Gyre had reached 85
184 items per km2. Lusher et al.29 collected and identified 2315 plastic particles from surface waters in the northeast Atlantic, of which 89% were smaller than 5 mm, indicating that MPs dominated in quantity. In China's Yellow Sea in particular, the average abundance of MPs in the surface seawater reached 545 ± 282 items per m3, and sea cucumbers (a food widely eaten along China's coasts) in the Yellow Sea have been found to ingest more MPs than other organisms.30 Meteorological phenomena such as rainfall and storms can also change the abundance and fate of MPs in the ocean to some extent. Hitchcock31 analyzed the variation of the abundance of MPs in surface water during a storm event and found that the maximum abundance of MPs reached 17
833 m−3 during the peak of the storm. The results highlight the importance of meteorological factors with respect to MPs pollution in the ocean. Terrestrial activities are the main sources of plastic fragments in the marine environment. Concentrations of MPs had a significant positive correlation with population density and urban/suburban development,32 and MPs that wash up along coastlines tend to accumulate in close proximity to coastal urban-industrialized centers.33 Additionally, plastics enter the marine environment directly through wastewater discharge, sewer overflows, and shipping activities.34 MP pollution derived from fishery is often underestimated, even though ropes and nets produce sizable volumes of fragments under the effects of tides and other processes.35 Notably, one study estimated that plastic waste found on the sea surface is only a small fraction of the plastic waste that enters the ocean, and that most plastic ends up on the sea bottom.23 In reality, after MPs are released into aquatic environments, they do not just exist as dispersed particles. Floating or suspended plastic particles interact with other substances or organisms in several ways, including via aggregation, adsorption, and phagocytosis, causing vertical movement of plastic particles in water.36 Moreover, MP sedimentation is reversible; even MPs in deep parts of water bodies or in the sediment can float back to the surface as a consequence of biological processes or water flows.13
3.2 Microplastics in the soil
While past studies on the distribution of MPs have tended to focus on the marine environment, recent studies have confirmed the significance of MPs in terrestrial ecosystems.37 MPs are found in soils around the world, and the annual input of MPs into soil ecosystems could be much greater than that into the global oceans, with an accumulated burden 4–23 times higher.38 Agriculture is one of the largest sources of soil MPs, releasing hundreds of thousands of tons of MPs into farmland each year.39 MPs can enter agricultural soil through the use of plastic mulch and coated fertilizers, among other sources, which can result in the accumulation of tens or hundreds of MPs per kilogram of soil.40,41 Improper disposal of municipal waste is another major source of MPs in the soil. It has been estimated that approximately a billion tons of municipal solid waste is generated globally each year, the majority of which is plastic waste; however, less than 20% of this can be converted into energy, while the rest is landfilled.42 A great amount of plastic thus ends up in landfills, where it is subject to severe environmental conditions, resulting in fragmentation to smaller and even nanoscale plastic particles.43 The fragmentation of plastics is a long-term process, and the amount of MPs will continue to increase as their particle size decreases. Plastic fragments with smaller particle sizes are more likely to enter the surrounding soil or water under the influence of rainfall, runoff, and wind.
3.3 Microplastics in freshwater
As noted above, a large proportion of marine plastic derives from terrestrial sources, and rivers are likely the main transportation pathway for plastic of various sizes.44 MPs in freshwater ecosystems come from a variety of sources, but spatial correlations between the types of MPs at a given location and human activities in the surrounding area have been reported, with major sources including urban waterways, industrial discharge, and effluent from wastewater treatment plants.38 Both point and non-point sources of pollution contribute significant volumes of MPs to rivers.45,46 Researchers have estimated that the annual discharge of MPs into surrounding rivers from a single industrial production site could be as high as 95.5 t.47 As the freshwater environment is not subject to the same mechanical effects that exacerbate plastic decomposition in the marine environment, such as strong tidal forces, it is generally believed that secondary MPs are mainly found in the latter. Nevertheless, analysis of the morphology and particle size of MPs in the freshwater environment has shown that these are not all primary; many are secondary MPs produced by the decomposition of larger plastics.48 Identification of a clear source is difficult, as secondary MPs can enter the freshwater environment through many pathways, and they can be generated before or after entering freshwater.49 A prime example of secondary MPs released into the environment are synthetic fibers detached from clothes by washing. It is estimated that 140
000–730
000 microfibers are released by an average 6 kg wash load of acrylic fabric.50 Those microfibers become secondary MPs before entering the environment. Most secondary MPs, however, are generated after entering the environment through mechanical abrasion, photodegradation, or biological processes, and they are then transported to the freshwater environment by wind, surface runoff, and other processes.51
3.4 Microplastics in the atmosphere
The diversity of polymer types found in atmospheric samples reported to date does not indicate an obvious distinction between polymer types of lesser or greater density, and polymers with greater densities have been reported in atmospheric deposition/air mass sampling.52 In terms of size and shape of MPs observed in the atmosphere, most are at the micron scale and are microfibers, with smaller proportions of foam, fragment, and film.53,54 MPs in urban atmospheres are often associated with intensive human activities, with major sources including synthetic fabric wear, waste incineration, industrial cutting, vehicle tire wear, and urban dust.55,56 The atmosphere plays a vital role in the transportation of MPs. Furthermore, atmospheric circulation and wet and dry deposition may be important pathways by which MPs from terrestrial sources enter other environmental compartments, which can influence the source-sink dynamics of plastic pollution in various ecosystems.52,57 Additionally, rainfall and heat cycles affect the distribution of atmospheric MPs.58 This suggests that the storage and transportation of plastics in the atmosphere differ based on the prevailing meteorological conditions at different temporal or spatial scales. Although the above studies confirmed that MPs are ubiquitous in the atmosphere and that terrestrial MPs can be transported to the marine environment, it is still unclear to what extent atmospheric transportation contributes to aquatic and terrestrial pollution. Thus, further research is required regarding these transportation processes and their relationship to meteorological conditions.
4 Environmental behavior of microplastics
4.1 Adsorption of pollutants on microplastics
Because of the large SSA and strong hydrophobicity of MPs, they can adsorb a variety of environmental pollutants, particularly organic chemicals that are themselves characterized by high hydrophobicity.59 Studies have confirmed that MPs have a strong adsorption affinity toward persistent organic pollutants, including dichlorodiphenyltrichloroethane (DDT), polycyclic aromatic hydrocarbons (PAHs), polychlorinated biphenyls (PCBs), polybrominated diphenyl ethers (PBDEs), and dioxins.60–63 The adsorption of pollutants on MPs is extremely complicated under heterogeneous environmental conditions because of the variety of factors involved, and exploring the methods and mechanisms of this adsorption is key to understanding the environmental behavior of MPs.
Two of the main factors that affect adsorption capacity are particle size and SSA. Generally, as particle size decreases, SSA increases and the number of adsorption sites increases, thereby increasing adsorption capacity.64 The sorption capacity of polypropylene (PP) MPs for 3,6-dibromocarbazole and 1,3,6,8-tetrabromocarbazole increases with the decrease in PP particle size.65 Wang et al.66 studied the sorption of the PAH pyrene on MPs with different SSAs (high-density polyethylene (PE), polystyrene (PS), and polyvinyl chloride (PVC)) and demonstrated the importance of SSA in the adsorption of organic pollutants by MPs. In the environment, MPs are susceptible to degradation and decomposition under the influence of light, weathering, or biological processes, which increases the SSA and porosity of the material, thereby providing pollutants with more adsorption sites.67 More importantly, a reduction in the size of MPs increases the toxicity of pollutants that cannot be absorbed into the MP matrix, thus posing a greater threat to organisms.
Furthermore, MP crystallinity may also play an important role in adsorption. Based on the alignment of their molecular chains, polymers can be classed as crystalline, amorphous, or semi-crystalline (containing both crystalline and amorphous regions). The crystalline regions of polymer molecular chains are regular, compact, and orderly, whereas their amorphous regions are arranged randomly with substantial distances between chains.68 The lower the crystallinity of a polymer, the greater the mass or volume ratio occupied by its amorphous regions, and environmental pollutants have an affinity to these regions.69 Liu et al.70 found that crystallinity is significantly negatively correlated with the adsorption capacity of MPs. Guo et al.71 examined the sorption of hydrophobic organic contaminants (HOCs) by three types of polymers (PE, PS, and polyphenylene oxide [PPO]) and found that their sorption coefficients increased with the decrease in crystallinity. Nevertheless, the sorption of pollutants onto pristine MPs decreased as crystallinity increased, but the opposite trend was observed with aged MPs.72 This may be related to changes in SSA, crystallinity, or surface functional groups brought about by aging.
Additionally, the functional groups and polarity of MPs are important because of their impact on the adsorption capacity of MPs. Aromatic rings enhance the hydrophobicity of MPs and facilitate π–π interactions with aromatic compounds.73 Oxygen atoms in certain oxygen-containing functional groups (e.g., carboxylic acids and esters) can form hydrogen bonds with hydrogen in water molecules, which decreases the sorption capacity of substances.72 Hydrophilic groups can reduce hydrophobicity, thus affecting the adsorption of hydrophobic substances.15 Moreover, the presence of polar functional groups, such as hydroxyl, carboxyl, and ether groups, enhances the polarity of substances, thus affecting the adsorption of polar chemicals.74 However, functional groups and polarity alone cannot fully explain differences in adsorption capacity. Researchers have found that polar polyamide (PA) MPs demonstrated a higher adsorption capacity for four antibiotics (ciprofloxacin, trimethoprim, amoxicillin, and tetracycline), while polar PVC MPs had less affinity for the same antibiotics.75
In addition to the effects of MP properties on adsorption, as outlined above, environmental factors such as salinity, pH, and temperature have an even greater impact on the adsorption of pollutants by MPs. Temperature changes alter the surface tension and water solubility of organic pollutants, which affects their adsorption on MPs.76 Because of the existence of an optimum temperature for the adsorption of organic pollutants onto MPs, an ambient temperature that is too high or too low will reduce the adsorption capacity of MPs.77 An early study found that the adsorption capacity of MPs in river water was greater than that in seawater because of the effect of salt ions.78 Subsequent studies have shown that salt ions reduce the partition coefficients and adsorption of some organic pollutants onto MPs.79 Moreover, increased water salinity may reduce homogeneous or heterogeneous aggregation of MPs, thus affecting their adsorption behavior.12
In water systems, pH is a key parameter that affects chemical reactions as well as the balance of the reaction system. Some properties of MPs and other pollutants are directly affected by pH, which can affect the adsorption or desorption between pollutants and MPs.80 Acidic or alkaline conditions can influence MP protonation, making them inclined to adsorb cations or anions.81 Li et al.75 found that pH can affect the form (molecule or ion) of organic pollutants in water, which in turn affects the electrostatic attraction between them and the surface of MPs. Wan et al.82 used a spectroscopic technique to measure the average hydrodynamic diameter of PS microbeads and found that it decreased as pH increased. This indicates that pH may affect the adsorption sites on the surface of MPs by changing the aggregation characteristics of MPs in a solution, thus affecting the adsorption capacity.
4.2 Interactions between microplastics and pollutants
As noted above, the adsorption of environmental pollutants to MPs depends on the affinity between the polymer and the pollutant, which is affected by a variety of factors. The forces between pollutants and MPs are diverse and complex; they include electrostatic forces, van der Waals forces, hydrogen bonds, and other intermolecular forces (Fig. 1).
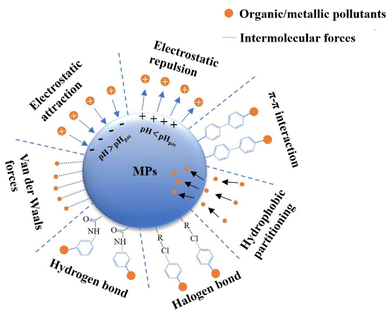 |
| Fig. 1 Main interactions between microplastics and environmental pollutants. | |
Electrostatic interactions play a vital role in the adsorption of environmental pollutants to MPs. These interactions are determined by the surface charge of the MP itself, point of zero charge (pHpzc) of the MP, electric charge of the pollutant, dissociation constant (pKa) of the pollutant, and pH of the solution.83 The pH of the solution and the pKa of the pollutant have a direct influence on the form and electrical charge of chemical compounds. When the pH of the solution exceeds the pHpzc of the MP, the surface of the MP will be negatively charged, causing it to adsorb positively charged organic pollutants by electrostatic attraction. When the pH value of the solution exceeds the pKa of the organic pollutant, the organic pollutant will deprotonate and exist as an anion in the solution, causing electrostatic repulsion and inhibiting the adsorption of the pollutant by the MP.74 For example, in fresh water (pH 6.7–7.1), electrostatic attraction occurs between positively charged ciprofloxacin and negatively charged MPs, which increases adsorption.75 However, at the same pH value, sulfamethoxazole is negatively charged, resulting in electrostatic repulsion with MPs, which reduces adsorption.84
The term “van der Waals forces” refers to intermolecular interactions. These forces guide the physical adsorption between environmental pollutants and MPs, and they can result in multilayer adsorption.85 They also affect the adsorption of environmental pollutants by aliphatic polymers, such as PE and PVC.74 Hüffer et al.86 found that seven aliphatic and aromatic organic compounds (namely n-hexane, cyclohexane, benzene, toluene, chlorobenzene, ethyl benzoate, and naphthalene) can only be adsorbed on aliphatic PE MPs by van der Waals forces. Xu et al.87 found that the adsorption of sulfamethoxazole (SMX) on PE can only be explained by van der Waals forces. Nevertheless, van der Waals forces are weak interactions that do not create intermolecular chemical bonds. Guo et al.73 compared the adsorption of the aromatic polymer PS and the aliphatic polymer PE on SMX and found that the benzene ring substituent in PS increased its aromaticity, allowing it to generate a π–π bond with SMX. This force is much greater than van der Waals forces and causes greater adsorption of SMX by PS than by PE.
π–π interactions and hydrogen bonds can also play a major or minor role (depending on the dominant forces) in the adsorption of environmental pollutants by MPs.88 These interactions are intermolecular interactions of certain compounds with conjugated structures, which can greatly increase the affinity between aromatic polymers and aromatic compounds.80 The π–π interaction between PS and SMX mentioned above increases the adsorption of SMX by PS. Hydrogen bonding was found to occur between the amide group (proton donor group) of PA and the carbonyl groups of hydrophilic organic contaminants (proton acceptor).89 Oxygen-containing functional groups (namely carboxyl or hydroxyl groups) formed during the aging of MPs may also increase hydrogen bonding in the course of adsorption.74 Liu et al.72 used Fourier Transform Infrared Spectroscopy (FTIR) to analyze the interactions between MPs and CIP before and after adsorption and found that the hydrogen bonding peak was at 3500 cm−1, which demonstrates that hydrogen bonding is a possible adsorption mechanism.
Hydrogen and halogen bonds can also enhance molecular interactions to some extent. For example, the amide bond in the PA-MP molecular chain can function as a hydrogen bond acceptor, while the hydroxyl group contained in bisphenol A can act as a hydrogen bond donor, resulting in a hydrogen bond between BPA and PA MPs. Although the weak hydrophobicity of PA MPs renders the hydrophobic interaction between PA MPs and bisphenol A a weak interaction, the strong bond energy of the hydrogen bond contributes binding sites of greater stability, thus offsetting the influence of the weak hydrophobic interaction.90 Wu et al.91 studied the adsorption of bisphenols on PVC MPs and found that the halogen atoms in PVC can act as electron acceptors to bond with the π electron on the benzene ring.
These results indicate that the interaction forces between pollutants and MPs are diverse and complex, and that they are influenced not only by the characteristics of MPs and organic compounds, but also by environmental factors.
5 Ecological effects of microplastics
Their prevalence in aquatic ecosystems has exposed many freshwater, marine, and benthic organisms to MPs and increased the probability of aquatic organisms taking in these particles through feeding, inhalation, and dermal contact. Starting with accumulation in smaller aquatic organisms, MPs can be transferred and accumulate across trophic levels.92 Researchers have confirmed the presence of MPs in organisms using different methods including dissection, tissue digestion, microscopy, FTIR, and Raman spectroscopy (Table 2). The most common MPs found in aquatic organisms are PET, PE, PES, PP, and nylon fibers. The average density of MPs found in organisms in various studies varied significantly, with a minimum of 0.2 plastic particles (pp) per ind and a maximum of 81.4 pp·per ind.65 Some laboratory studies have shown that higher trophic-level organisms tend to ingest more MPs.93 This suggests that the whole food chain is at risk of MP contamination. Furthermore, it should be noted that because of the difficulty in identifying and analyzing very small MPs, the MP size detection threshold in organisms in the field is usually >100 μm. As a result, the presence of MPs in living organisms may be significantly underestimated.
Table 2 Studies on microplastics in organisms in natural environments
Location |
Organism |
Detection method |
Microplastic contenta |
Main type of plastic |
Ref. |
pp: plastic particles; ind: individual; dw: dry weight; ww: wet weight. |
Pearl river, China |
Freshwater fish |
Digestion, filtration, separation and weighing, microscopy, mFTIR |
0.2–81.4 pp·per ind |
PET, PE, PP |
65 |
Lake Tai, China |
Corbicula fluminea |
Digestion, filtration, microscopy, SEM/EDS |
0.2–12.5 pp·per g ww |
Fibers |
105 |
Culture ponds, Shanghai, China |
Crayfish |
Digestive tract isolation, digestion, filtration, separation, mFTIR |
2.5 ± 0.6 pp·per ind |
PE and PP fibers |
106 |
Rivers in SW Wales, UK |
Ephemeroptera and Trichoptera |
Homogenization, digestion, microscopy |
0.14 pp·per mg dw |
— |
92 |
Brazos river, USA |
Centrarchidae |
Homogenization, filtration, microscopy |
0.34–1.33 pp·per ind |
— |
107 |
Western English Channel |
Saltwater fish larvae |
Dissection, microscopy, mFTIR |
— |
Nylon |
108 |
Fish farms, California, USA |
Oysters |
Digestion, microscopy |
1.8 pp·per ind |
Fibers |
109 |
Three Gorges reservoir, China |
Freshwater fish |
Digestion, filtration, microscopy, Raman spectroscopy |
0.33 ± 0.58–1.5 ± 1.38 pp·per ind |
PE and nylon fibers fragments |
110 |
Lake Geneva, Switzerland |
Birds |
Digestive tract isolation, microscopy |
4.3 ± 2.6 pp·per ind |
Fragments, foam, films, microbeads, fibers |
111 |
Bloukrans river, South Africa |
Chironomus spp. |
Digestion, filtration, microscopy |
0.37 ± 0.44–1.12 ± 1.19 pp·per mg |
— |
112 |
Thames river, UK |
Rutilus rutilus |
Digestive tract isolation, Raman spectroscopy |
0.69 pp·per ind |
PE, PP, PES |
6 |
English Channel |
Crangon crangon |
Dissection, digestion, microscopy |
0.75 pp·per g ww |
Fragments (200–1000 μm) |
11 |
Tributaries of Lake Michigan, USA |
Freshwater fish |
Dissection, digestion, filtration, microscopy |
10 ± 2.3–13 ± 1.6 pp·per ind |
Fibers |
113 |
River Irwell, Manchester, UK |
Tubifex tubifex |
Digestion, filtration, microscopy, FTIR |
129 ± 65.4 pp·per g dw |
Fibers (87%), fragments (13%) |
93 |
To date, few studies have evaluated the exposure of aquatic organisms to MPs, and even fewer have investigated the adverse effects of MPs on organisms. Several recent studies have observed a number of biological behavioral changes and dysfunctions, as well as growth retardation and reproduction inhibition caused by MPs.94,95 After being ingested by organisms, MPs can also cause physical damage to the gastrointestinal system, such as entanglement and digestive tract obstruction.96 Zhang et al.97 examined the interaction between the primary producer Skeletonema costatum and MPs. They discovered that PVC MPs (average diameter 1 μm) had an obvious inhibitory effect on the growth of S. costatum, and that high concentrations of PVC MPs had negative effects on its photosynthetic efficiency. Using exposure tests, Kokalj et al.98 confirmed the ingestion of four different environmentally relevant MPs (from two facial cleansers, a type of plastic bag, and polyethylene textile fleece) by zooplankton crustaceans. Khan et al.99 investigated the potential acute and long-term effects of micronized car tire wear particles on the amphipod Hyallela azteca and showed that the median lethal concentration (LC50) was 3426 ± 172 pp·per mL and that higher exposure concentrations (>500 pp·per mL) significantly increased mortality, reproductive output, and net growth after 21 days. Karami et al.100 investigated the biomarker responses of African catfish exposed to low-density polyethylene (LDPE) fragments (50 μg L−1) and found that exposure led to severe hyperplasia, along with an increase in the degree of tissue change and an increase in plasma albumin. Moreover, studies have shown that smaller MPs can enter the circulatory system of organisms and phagocytes, and that their long-term retention may cause serious damage to organisms.101 It is worth pointing out that MPs tend to accumulate pollutants in the environment, and that the bioavailability of these pollutants may increase following MP ingestion by organisms. Chen et al.102 confirmed the co-exposure of MPs and bisphenol A led to increased neurotoxic effects in zebrafish by inducing significant effects on various biomarkers in the central nervous system and the dopaminergic systems. Hu et al.103 found that PAHs and their derivatives carried by MPs are highly bioavailable in the digestive system and may induce human cancer risks. MPs surfaces also pose a problem as they are a niche ready for colonisation by diverse biofilm assemblages, composed of specific bacterial communities and putative pathogens prone to acquiring antibiotic resistance genes and resistance in the biofilm.104 These results suggest that MPs can cause both direct (physical) and indirect (chemical) damage to organisms.
6 Conclusion
This study reviewed the sources, distribution, and fates of MPs in the environment. Although data on these topics are limited, the existing literature clearly shows that MPs are prevalent in the environment and that they circulate between environmental compartments via rivers and the atmosphere, thereby affecting locations far from the sources of MP pollution. The lack of standardization of sampling, classification, and identification methods used to date has resulted in a lack of comparable high-quality data on the abundance and characteristics of MPs. Furthermore, no consensus has yet been reached on the main sources and pollution pathways of MPs in the environment. Given the widespread use of plastic products in human production activities and everyday life, the pathways through which MP pollution reaches the environment are complex. There is therefore an urgent requirement for timely analyses of the sources of MPs, improvements in the regulations on the control of MP pollution sources, and the implementation of controls of the use and emission of MPs at national levels. Finally, research is lacking on the interactions of MPs with other polluting substances in the environment, especially new pollutants. As the release of MP additives and surface-adsorbent pollutants in organisms causes far more harm to organisms than the impact of MPs alone, increased attention must be paid to the range of toxic effects that MPs as pollutant-carriers can cause when ingested by organisms, including humans.
Author contributions
Wang Li: conceptualization, writing – original draft. Bo Zu: conceptualization, supervision. Qingwei Yang: review & editing. Juncheng Guo and Jiawen Li: editing.
Conflicts of interest
There are no conflicts to declare.
Acknowledgements
This study was financially supported by the Natural Science Foundation of Chongqing, China (cstc2020jcyj-msxmX0763).
References
- B. Li, H. Wan, Y. Cai, J. Peng, B. Li, Q. Jia, X. Yuan, Y. Wang, P. Zhang and B. Hong, Sci. Total Environ., 2022, 820, 187–206 Search PubMed.
- H. Yu, W. Qi, X. Cao, J. Hu, Y. Li, J. Peng, C. Hu and J. Qu, Environ. Int., 2021, 156, 12–21 Search PubMed.
- R. Z. Habib, T. Thiemann and R. A. Kendi, J. Water Resour. Prot., 2020, 12, 1–35 CrossRef CAS.
- L. Li, X. Zhao, Z. Li and K. Song, J. Hazard. Mater., 2020, 411, 124955 CrossRef PubMed.
- J. R. Jambeck, R. Geyer, C. Wilcox, T. R. Siegler and K. L. Law, Science, 2015, 347, 768–771 CrossRef CAS PubMed.
- A. A. Horton, M. D. Jürgens, E. Lahive, P. M. van Bodegom and M. G. Vijver, Environ. Pollut., 2018, 236, 188–194 CrossRef CAS PubMed.
- J. Wang, S. Coffin, D. Schlenk and J. Gan, Environ. Sci. Technol., 2020, 54, 11220–11229 CrossRef CAS PubMed.
- R. C. Thompson, Y. Olsen, R. P. Mitchell, A. Davis, S. J. Rowland, A. W. G. John, D. McGonigle and A. E. Russell, Science, 2004, 304, 838 CrossRef CAS PubMed.
- M. Ahechti, M. Benomar, M. El Alami and C. Mendiguchía, Int. J. Environ. Anal. Chem., 2020, 102, 1118–1125 CrossRef.
- B. Li, W. Liang, Q.-X. Liu, S. Fu, C. Ma, Q. Chen, L. Su, N. J. Craig and H. Shi, Environ. Sci. Technol., 2021, 55, 71–79 Search PubMed.
- D. Eerkes-Medrano, R. C. Thompson and D. C. Aldridge, Water Res., 2015, 75, 63–82 CrossRef CAS PubMed.
- S. Li, H. Liu, R. Gao, A. Abdurahman, J. Dai and F. Zeng, Environ. Pollut., 2018, 237, 126–132 CrossRef CAS PubMed.
- X. Wang, N. Bolan, D. C. W. Tsang, B. Sarkar, L. Bradney and Y. Li, J. Hazard. Mater., 2021, 402, 123496 CrossRef CAS PubMed.
- O. S. Alimi, J. F. Budarz, L. M. Hernandez and N. Tufenkji, Environ. Sci. Technol., 2018, 52, 1704–1724 CrossRef CAS PubMed.
- M. Munoz, D. Ortiz, J. Nieto-Sandoval, Z. M. de Pedro and J. A. Casas, Chemosphere, 2021, 283, 131085 CrossRef CAS PubMed.
- A. Tursi, M. Baratta, T. Easton, E. Chatzisymeon, F. Chidichimo, M. De Biase and G. De Filpo, RSC Adv., 2022, 12, 28318–28340 RSC.
- F. De Falco, M. P. Gullo, G. Gentile, E. Di Pace, M. Cocca, L. Gelabert, M. Brouta-Agnesa, A. Rovira, R. Escudero and R. Villalba, Environ. Pollut., 2017, 236, 916–925 CrossRef PubMed.
- A. L. Andrady, Mar. Pollut. Bull., 2011, 62, 1596–1605 CrossRef CAS PubMed.
- S. Lambert, C. Scherer and M. Wagner, Integr. Environ. Assess. Manage., 2017, 13, 470–475 CrossRef CAS PubMed.
- A. A. Horton, A. Walton, D. J. Spurgeon, E. Lahive and C. Svendsen, Sci. Total Environ., 2017, 586, 127–141 CrossRef CAS PubMed.
- X. Guo and J. Wang, Mar. Pollut. Bull., 2019, 142, 1–14 CrossRef CAS PubMed.
- Z.-M. Wang, J. Wagner, S. Ghosal, G. Bedi and S. Wall, Sci. Total Environ., 2017, 603, 616–626 CrossRef PubMed.
- M. Kooi, S. Primpke, S. M. Mintenig, C. Lorenz, G. Gerdts and A. A. Koelmans, Water Res., 2021, 202, 117429 CrossRef CAS PubMed.
- L. Cabernard, L. Roscher, C. Lorenz, G. Gerdts and S. Primpke, Environ. Sci. Technol., 2018, 52, 13279–13288 CrossRef CAS PubMed.
- C. A. Peters, E. Hendrickson, E. C. Minor, K. Schreiner, J. Halbur and S. P. Bratton, Mar. Pollut. Bull., 2018, 137, 91–95 CrossRef CAS PubMed.
- S. Bejgarn, M. Macleod, C. Bogdal and M. Breitholtz, Chemosphere, 2015, 132, 114–119 CrossRef CAS PubMed.
- M. Cai, H. He, M. Liu, S. Li, G. Tang, W. Wang, P. Huang, G. Wei, Y. Lin and B. Chen, Sci. Total Environ., 2018, 633, 1206–1216 CrossRef CAS PubMed.
- H. S. Carson, M. R. Lamson, D. Nakashima, D. Toloumu, J. Hafner, N. Maximenko and K. J. McDermid, Mar. Environ. Res., 2013, 84, 76–83 CrossRef CAS PubMed.
- A. L. Lusher, V. Tirelli, I. O'Connor and R. Officer, Sci. Rep., 2015, 5, 1–9 Search PubMed.
- Q. Li and X. Sun, Anthropocene Coasts, 2020, 3, 43–52 CrossRef.
- J. N. Hitchcock, Sci. Total Environ., 2020, 734, 139436 CrossRef CAS PubMed.
- L. T. Yonkos, E. A. Friedel, A. C. Perez-Reyes, S. Ghosal and C. D. Arthur, Environ. Sci. Technol., 2014, 48, 14195–14202 CrossRef CAS PubMed.
- C. Collins and J. Hermes, Mar. Pollut. Bull., 2019, 139, 46–58 CrossRef CAS PubMed.
- G. Schernewski, H. Radtke, E. Robbe, M. Haseler, R. Hauk, L. Meyer, S. Piehl, J. Riedel and M. Labrenz, Environ. Manage., 2021, 68, 860–881 CrossRef PubMed.
- B. Xue, L. Zhang, R. Li, Y. Wang, J. Guo, K. Yu and S. Wang, Environ. Sci. Technol., 2020, 54, 2210–2217 CrossRef CAS PubMed.
- Y. Li, H. Zhang and C. Tang, Anthropocene Coasts, 2020, 3, 6–13 CrossRef.
- Y. Sun, C. Duan, N. Cao, X. Li, X. Li, Y. Chen, Y. Huang and J. Wang, Sci. Total Environ., 2022, 806, 150516 CrossRef CAS PubMed.
- L. Nizzetto, M. Futter and S. Langaas, Environ. Sci. Technol., 2016, 50, 10777–10779 CrossRef CAS PubMed.
- J. Cao, X. Zhao, X. Gao, L. Zhang, Q. Hu and K. H. M. Siddique, J. Environ. Manag., 2021, 294, 112997 CrossRef CAS PubMed.
- N. Katsumi, T. Kusube, S. Nagao and H. Okochi, Chemosphere, 2021, 267, 129185 CrossRef CAS PubMed.
- B. Zhou, J. Wang, H. Zhang, H. Shi, Y. Fei, S. Huang, Y. Tong, D. Wen, Y. Luo and D. Barceló, J. Hazard. Mater., 2020, 388, 121814 CrossRef CAS PubMed.
- H. Golwala, X. Zhang, S. M. Iskander and A. L. Smith, Sci. Total Environ., 2021, 769, 144581 CrossRef CAS PubMed.
- P. He, L. Chen, L. Shao, H. Zhang and F. Lu, Water Res., 2019, 159, 38–45 CrossRef CAS PubMed.
- J. S. Chauhan, D. Semwal, M. Nainwal, N. Badola and P. Thapliyal, Environment Development and Sustainability, 2021, 23, 16819–16833 CrossRef.
- A. Kabir, M. Sekine, T. Imai, K. Yamamoto and T. Higuchi, Sci. Total Environ., 2021, 144655, DOI:10.1016/j.scitotenv.2020.144655.
- Z. Wang, Y. Zhang, S. Kang, L. Yang, H. Shi, L. Tripathee and T. Gao, Sci. Total Environ., 2021, 795, 148888 CrossRef CAS PubMed.
- A. Lechner and D. Ramler, Environ Pollut., 2015, 200, 159–160 CrossRef CAS PubMed.
- Y. Fan, K. Zheng, Z. Zhu, G. Chen and X. Peng, Environ. Pollut., 2019, 251, 862–870 CrossRef CAS PubMed.
- S. Lasee, J. Mauricio, W. A. Thompson, A. Karnjanapiboonwong, J. Kasumba, S. Subbiah, A. N. Morse and T. A. Anderson, Integr. Environ. Assess. Manage., 2017, 13, 528–532 CrossRef CAS PubMed.
- I. E. Napper and R. C. Thompson, Mar. Pollut. Bull., 2016, 112, 39–45 CrossRef CAS PubMed.
- J. P. Da Costa, A. R. Nunes, P. S. Santos, A. V. Girao, A. C. Duarte and T. Rocha-Santos, J. Environ. Sci. Health, Part A: Toxic/Hazard. Subst. Environ. Eng., 2018, 53, 866–875 CrossRef CAS PubMed.
- Y. Zhang, S. Kang, S. Allen, D. Allen and M. Sillanp, Earth-Sci. Rev., 2020, 203, 103118 CrossRef CAS.
- G. Chen, Z. Fu, H. Yang and J. Wang, TrAC, Trends Anal. Chem., 2020, 130, 115981 CrossRef CAS.
- K. Liu, X. Wang, T. Fang, P. Xu, L. Zhu and D. Li, Sci. Total Environ., 2019, 675, 462–471 CrossRef CAS PubMed.
- N. Evangeliou, H. Grythe, Z. Klimont, C. Heyes, S. Eckhardt, S. Lopez-Aparicio and A. Stohl, Nat. Commun., 2020, 11, 1–11 CrossRef PubMed.
- F. Liu, K. B. Olesen, A. R. Borregaard and J. Vollertsen, Sci. Total Environ., 2019, 671, 992–1000 CrossRef CAS.
- K. Liu, T. Wu, X. Wang, Z. Song, C. Zong, N. Wei and D. Li, Environ. Sci. Technol., 2019, 53, 10612–10619 CrossRef CAS PubMed.
- A. I. S. Purwiyanto, T. Prartono, E. Riani, Y. Naulita, M. R. Cordova and A. F. Koropitan, Mar. Pollut. Bull., 2022, 174, 113195 CrossRef CAS PubMed.
- N. Weithmann, J. N. Möller, M. G. Löder, S. Piehl, C. Laforsch and R. Freitag, Sci. Adv., 2018, 4, 8060 CrossRef PubMed.
- C.-F. Chen, Y.-R. Ju, Y. C. Lim, N.-H. Hsu, K.-T. Lu, S.-L. Hsieh, C.-D. Dong and C.-W. Chen, Chemosphere, 2020, 254, 126818 CrossRef CAS PubMed.
- O. H. Fred-Ahmadu, I. T. Tenebe, O. O. Ayejuyo and N. U. Benson, Chemosphere, 2022, 298, 134193 CrossRef CAS PubMed.
- H.-S. Lo, C.-Y. Wong, N. F.-Y. Tam and S.-G. Cheung, Chemosphere, 2019, 219, 418–426 CrossRef CAS PubMed.
- B. G. Yeo, H. Takada, R. Yamashita, Y. Okazaki, K. Uchida, T. Tokai, K. Tanaka and N. Trenholm, Mar. Pollut. Bull., 2020, 151, 110806 CrossRef CAS PubMed.
- N. Naqash, S. Prakash, D. Kapoor and R. Singh, Environ. Chem. Lett., 2020, 18, 1–12 CrossRef.
- K. Zheng, Y. Fan, Z. Zhu, G. Chen, C. Tang and X. Peng, Environ. Toxicol. Chem., 2019, 38, 1504–1513 CrossRef CAS PubMed.
- W. Wang and J. Wang, Chemosphere, 2018, 193, 567–573 CrossRef CAS PubMed.
- R. Frankowski, J. Platkiewicz, E. Stanisz, T. Grzeskowiak and A. Zgola-Grzeskowiak, Environ. Pollut., 2021, 289, 117947 CrossRef CAS PubMed.
- O. H. Fred-Ahmadu, G. Bhagwat, I. Oluyoye, N. U. Benson, O. O. Ayejuyo and T. Palanisami, Sci. Total Environ., 2020, 706, 135978 CrossRef CAS PubMed.
- Y. Zhou, Y. Yang, G. Liu, G. He and W. Liu, Water Res., 2020, 184, 116209 CrossRef CAS PubMed.
- X. Liu, H. Shi, B. Xie, D. D. Dionysiou and Y. Zhao, Environ. Sci. Technol., 2019, 53, 10188–10196 CrossRef CAS PubMed.
- X. Guo, X. Wang, X. Zhou, X. Kong, S. Tao and B. Xing, Environ. Sci. Technol., 2012, 46, 7252–7259 CrossRef CAS PubMed.
- G. Liu, Z. Zhu, Y. Yang, Y. Sun, F. Yu and J. Ma, Environ. Pollut., 2019, 246, 26–33 CrossRef CAS PubMed.
- X. Guo and J. Wang, Mar. Pollut. Bull., 2019, 149, 110511 CrossRef CAS PubMed.
- L. Fu, J. Li, G. Wang, Y. Luan and W. Dai, Ecotoxicol. Environ. Saf., 2021, 217, 112207 CrossRef CAS PubMed.
- J. Li, K. Zhang and H. Zhang, Environ. Pollut., 2018, 237, 460–467 CrossRef CAS PubMed.
- S. Chen, Z. Tan, Y. Qi and C. Ouyang, Mar. Pollut. Bull., 2019, 149, 110490 CrossRef CAS PubMed.
- X. Zhang, M. Zheng, L. Wang, Y. Lou, L. Shi and S. Jiang, Mar. Pollut. Bull., 2018, 126, 606–609 CrossRef CAS PubMed.
- L. A. Holmes, A. Turner and R. C. Thompson, Mar. Chem., 2014, 167, 25–32 CrossRef CAS.
- T. Wang, L. Wang, Q. Chen, N. Kalogerakis, R. Ji and Y. Ma, Sci. Total Environ., 2020, 142427, DOI:10.1016/j.scitotenv.2020.142427.
- X. Guo, J. Pang, S. Chen and H. Jia, Chemosphere, 2018, 209, 240–245 CrossRef CAS PubMed.
- F. Wang, K. M. Shih and X. Y. Li, Chemosphere, 2015, 119, 841–847 CrossRef CAS PubMed.
- T. Wan, S. Lu, W. Cheng, J. Ren, M. Wang, B. Hu, Z. Jia, Y. Li and Y. Sun, Environ. Pollut., 2019, 249, 398–405 CrossRef CAS PubMed.
- L. M. R. Mendoza and M. Balcer, TrAC, Trends Anal. Chem., 2019, 113, 402–408 CrossRef.
- R. M. Razanajatovo, J. N. Ding, S. S. Zhang, H. Jiang and H. Zou, Mar. Pollut. Bull., 2018, 136, 516–523 CrossRef CAS PubMed.
- F. Wang, M. Zhang, W. Sha, Y. Wang, H. Hao, Y. Dou and Y. Li, Molecules, 2020, 25, 1827 CrossRef CAS PubMed.
- T. Hüffer and T. Hofmann, Environ. Pollut., 2016, 214, 194–201 CrossRef PubMed.
- B. L. Xu, F. Liu, P. C. Brookes and J. M. Xu, Mar. Pollut. Bull., 2018, 131, 191–196 CrossRef CAS PubMed.
- A. Ghaffar, S. Ghosh, F. Li, X. Dong, D. Zhang, M. Wu, H. Li and B. Pan, Environ. Pollut., 2015, 206, 502–509 CrossRef CAS PubMed.
- W. Mei, G. Chen, J. Bao, M. Song, Y. Li and C. Luo, Sci. Total Environ., 2020, 736, 139472 CrossRef CAS PubMed.
- A. W. Verla, C. E. Enyoh, E. N. Verla and K. O. Nwarnorh, SN Appl. Sci., 2019, 1, 1–30 CAS.
- P. Wu, Z. Cai, H. Jin and Y. Tang, Sci. Total Environ., 2019, 650, 671–678 CrossRef CAS PubMed.
- F. M. Windsor, I. Durance, A. A. Horton, R. C. Thompson, C. R. Tyler and S. J. Ormerod, Global Change Biol., 2019, 25, 1207–1221 CrossRef PubMed.
- R. R. Hurley, J. C. Woodward and J. J. Rothwell, Environ. Sci. Technol., 2017, 51, 12844–12851 CrossRef CAS PubMed.
- T. Q. Chagas, A. P. da Costa Araújo and G. Malafaia, Sci. Total Environ., 2021, 761, 143231 CrossRef CAS PubMed.
- X. Zhang, M. Xia, J. Zhao, Z. Cao, W. Zou and Q. Zhou, Environ. Int., 2022, 158, 106922 CrossRef CAS PubMed.
- C. C. Chen, Y. Shi, Y. Zhu, J. Zeng, W. Qian, S. Zhou, J. Ma, K. Pan, Y. Jiang and Y. Tao, Water Res., 2022, 219, 118536 CrossRef CAS PubMed.
- C. Zhang, X. Chen, J. Wang and L. Tan, Environ. Pollut., 2017, 220, 1282–1288 CrossRef CAS PubMed.
- A. J. Kokalj, U. Kunej and T. Skalar, Chemosphere, 2018, 208, 522–529 CrossRef CAS PubMed.
- F. R. Khan, L. L. Halle and A. Palmqvist, Aquat. Toxicol., 2019, 213, 105216 CrossRef CAS PubMed.
- A. Karami, N. Romano, T. Galloway and H. Hamzah, Environ. Res., 2016, 151, 58–70 CrossRef CAS PubMed.
- J. C. Prata, J. P. da Costa, I. Lopes, A. C. Duarte and T. Rocha-Santos, Sci. Total Environ., 2020, 702, 134455 CrossRef CAS PubMed.
- Q. Chen, D. Yin, Y. Jia, S. Schiwy, J. Legradi, S. Yang and H. Hollert, Sci. Total Environ., 2017, 609, 1312–1321 CrossRef CAS PubMed.
- X. Hu, Q. Yu, M. G. Waigi, W. Ling, C. Qin, J. Wang and Y. Gao, Environ. Int., 2022, 168, 107459 CrossRef CAS PubMed.
- K. Stenger, O. Wikmark, C. Bezuidenhout and L. Molale-Tom, Environ. Pollut., 2021, 291, 118130 CrossRef CAS PubMed.
- L. Su, Y. Xue, L. Li, D. Yang, P. Kolandhasamy, D. Li and H. Shi, Environ. Pollut., 2016, 216, 711–719 CrossRef CAS PubMed.
- W. Lv, W. Zhou, S. Lu, W. Huang, Q. Yuan, M. Tian, W. Lv and D. He, Sci. Total Environ., 2019, 652, 1209–1218 CrossRef PubMed.
- C. A. Peters and S. P. Bratton, Environ. Pollut., 2016, 210, 380–387 CrossRef CAS PubMed.
- M. Steer, M. Cole, R. C. Thompson and P. K. Lindeque, Environ. Pollut., 2017, 226, 250–259 CrossRef CAS PubMed.
- C. M. Rochman, A. Tahir, S. L. Williams, D. V. Baxa, R. Lam, J. T. Miller, F.-C. Teh, S. Werorilangi and S. J. Teh, Sci. Rep., 2015, 5, 1–10 Search PubMed.
- K. Zhang, X. Xiong, H. Hu, C. Wu, Y. Bi, Y. Wu, B. Zhou, P. K. S. Lam and J. Liu, Environ. Sci. Technol., 2017, 51, 3794–3801 CrossRef CAS PubMed.
- F. Faure, C. Demars, O. Wieser, M. Kunz and L. F. De Alencastro, Environ. Chem., 2015, 12, 582–591 CrossRef CAS.
- H. A. Nel, T. Dalu and R. J. Wasserman, Sci. Total Environ., 2018, 612, 950–956 CrossRef CAS PubMed.
- R. E. McNeish, L. Kim, H. Barrett, S. Mason, J. Kelly and T. Hoellein, Sci. Rep., 2018, 8, 1–12 CAS.
|
This journal is © The Royal Society of Chemistry 2023 |
Click here to see how this site uses Cookies. View our privacy policy here.