DOI:
10.1039/D3RA02164E
(Paper)
RSC Adv., 2023,
13, 21754-21768
Synthesis of AgBr/Ti3C2@TiO2 ternary composite for photocatalytic dehydrogenation of 1,4-dihydropyridine and photocatalytic degradation of tetracycline hydrochloride
Received
2nd April 2023
, Accepted 10th July 2023
First published on 19th July 2023
Abstract
In this work, AgBr/Ti3C2@TiO2 ternary composite photocatalyst was prepared by a solvothermal and precipitation method with the aims of introducing Ti3C2 as a cocatalyst and TiO2 as a compositing semiconductor. The crystal structure, morphology, elemental state, functional groups and photoelectrochemical properties were studied by XRD, SEM, TEM, XPS, FI-IR and EIS. The photocatalytic performances of the composites were investigated by the photodehydrogenation of diethyl 1,4-dihydro-2,6-dimethyl-3,5-pyridinedicarboxylate (1,4-DHP) and the photodegradation of tetracycline hydrochloride (TCH) under visible light irradiation (λ > 400 nm). The AgBr/Ti3C2@TiO2 composite photocatalyst showed enhanced photocatalytic performance in both photocatalytic reactions. The photocatalytic activity of the composite photocatalyst is dependent on the proportional content of Ti3C2@TiO2. With optimized Ti3C2@TiO2 proportion, the photocatalytic ability of the AgBr/Ti3C2@TiO2 composite was 24.5 times as high as that of Ti3C2@TiO2 for photodehydrogenation of 1,4-DHP and 1.9 times as high as that of pure AgBr for photodegradation of TCH. The enhanced photocatalytic performance of the AgBr/Ti3C2@TiO2 composite should be due to the formation of a p–n heterojunction structure between AgBr and Ti3C2@TiO2 and the excellent electronic properties of Ti3C2, which enhanced the visible light absorption capacity, lowered the internal resistance, speeded up the charge transfer and reduced the recombination efficiency of photo-generated carriers. Mechanism studies showed that superoxide free radical (˙O2−) was the main active species. In addition, the composite photocatalyst also displayed good stability, indicating its reutilization in practical application.
1. Introduction
With the rapid development of industrialization and urbanization, environmental pollution and the energy crisis have received extensive attention in the past decades.1–3 In order to solve these problems, inexhaustible solar-driven photocatalysis is considered to be a potential green method to produce photoinduced carriers with redox ability by using semiconductors as photocatalysts.4,5 So far, many photocatalysts have been reported in this field, such as TiO2,6,7 ZnO,8 CdS9,10 and g-C3N4.11,12 Among these photocatalysts, TiO2 has gained a lot of attention due to its high photocatalytic ability and good chemical stability. What's more, it is also low in price and easy to obtain, safe and harmless.13 However, the practical application of TiO2 as a photocatalyst is limited because it can only be excited by UV light that accounts for only a small fraction of the natural sunlight (∼5%).14,15 The method to overcome this shortcoming is to modify TiO2 with narrow band gap semiconductors. Under light irradiation, the photogenerated charge carriers can move from one semiconductor to another, thereby enhancing the photocatalytic activity.16–18
AgBr is an important photosensitive semiconductor for traditional use. It is an n-type semiconductor with a narrow band gap of ∼2.64 eV and is very active under visible light excitation.17 Unfortunately, the pure AgBr is quite unstable, and the photoinduced electrons will combine with Ag+ to form Ag0 clusters, resulting in the undesirable and uncontrolled photolysis of AgBr, which leads to the low reusability of AgBr in photocatalytic reaction.19–21 Therefore, the composite of AgBr and TiO2 can enhance the photocatalytic performance and improve the photocatalytic stability by reducing the band gap and prolonging the life of photoinduced electron–hole pairs. Abou Asi et al. prepared AgBr/TiO2 nanocomposite by a deposition–precipitation method with the addition of cetyltrimethylammonium bromide, and evaluated the photocatalytic activity by the reduction of CO2.22 Cui et al. successfully prepared AgBr nanoparticles modified TiO2 nanotube arrays (AgBr/TiO2 NTAs) photoelectrode by anodic oxidation and ultrasonic assisted precipitation. The enhance photocatalytic activity of the AgBr/TiO2 NTAs materials was evaluated by the degradation of 4-chlorophenol (4-CP) under visible light irradiation, and 92.6% of 4-CP degradation was obtained within 140 min of Xenon illumination.23
MXene, a new family of 2D transition metal carbides and/or nitrides, has attracted widespread interest since its first appearance in 2011.24 Up to now, MXenes has been widely studied and applied in energy storage and conversion,25 catalysis,26 sensor,27 heavy metal ion28 and dye adsorption29 and other fields. Because of the excellent conductivity and well visible light absorption capacity of Ti3C2 MXene, it has been clearly proved to be an efficient cocatalyst for improving photocatalytic activity, which can effectively promote the separation and transfer of photogenerated electrons. For example, Low et al. used the calcination method to grow TiO2 nanoparticles in situ on Ti3C2 MXene with good electrical conductivity. The photocatalytic reduction of CO2 to CH4 (0.22 μmol h−1) by the optimized TiO2/Ti3C2 composite is 3.7 times higher than that of commercial TiO2 (P25).30 Moreover, Li et al. modified carbon nitride (CN) with Ti3C2 and found that compared with the original CN, the modified CN showed significantly improved photocatalytic activity for U(VI) reduction.2
To the best of our knowledge, there is no report about the enhancement of the photocatalytic performance of AgBr by compositing with TiO2 and simultaneously introducing Ti3C2 as a cocatalyst. In this study, Ti3C2@TiO2 was prepared by in situ growth of TiO2 on Ti3C2 under solvothermal treatment, and a series of AgBr/Ti3C2@TiO2 composites were prepared by precipitation method. The in situ preparation of Ti3C2@TiO2 makes Ti3C2 and TiO2 closely contact, and improves the separation efficiency of photogenerated electron hole pairs. The photocatalytic performance of the AgBr/Ti3C2@TiO2 photocatalyst was evaluated by the photodehydrogenation of 1,4-DHP and photodegradation of TCH under visible light irradiation. The AgBr/Ti3C2@TiO2 photocatalyst showed enhanced photocatalytic performance in both photocatalytic reactions. The photocatalytic performance of the AgBr/Ti3C2@TiO2 composite with optimized composition is 3.9 and 24.5 times higher than that of pure AgBr and Ti3C2@TiO2, respectively, for photodehydrogenation of 1,4-DHP and 1.9 and 5.9 times higher than that of pure AgBr and Ti3C2@TiO2 for photodegradation of TCH. The composite photocatalyst were stable under light irradiation. The relative dehydrogenation efficiency of 1,4-DHP photolysis and the relative degradation efficiency of TCH degradation remained above 86% after four cycles.
2. Experimental
2.1. Preparation of AgBr powder
First, 1.0 g AgNO3 was dissolved in 50 mL of deionized water to obtain AgNO3 solution (solution A), 0.70 g KBr was mixed with 25 mL deionized water and dissolved to prepare KBr solution (solution B). Then, the solution B was added dropwise the solution A under magnetically stirring, and the mixed solution was stirred in the dark for 0.5 h. After centrifugation and washing, the AgBr precipitate was collected and dried at 60 °C.
2.2. Preparation of layered Ti3C2
Typically, 0.5 g Ti3AlC2 powder was added to 10 mL 30 wt% HF (within 5 min) and the reaction was allowed to proceed at room temperature for 5 h to remove the element of Al. The resulting powder was collected by centrifugation (3500 rpm, 5 min per cycle) and washed 5 times with deionized water. Then, the black precipitate was dried at 80 °C for 24 h to obtain multilayered Ti3C2.
2.3. Preparation of Ti3C2@TiO2 by in situ solvothermal method
First, 0.3 g Ti3C2, which was obtained from HF etching of Ti3AlC2, was mixed with 30 mL isopropanol (IPA). After sonication for 10 min, the mixture was poured into a 100 mL Teflon-lined reactor and heated at 150 °C for 24 h. The precipitate was centrifuged, repeatedly washed until the pH value of the eluate was about 6, and then dried at 60 °C to obtain Ti3C2@TiO2 sample.
2.4. Preparation of AgBr/Ti3C2@TiO2 composites
Solution A was prepared by dissolving 0.1808 g AgNO3 and dispersing 0.0225 g Ti3C2@TiO2 to 50 mL deionized water. Solution B was prepared by dissolving 0.1267 g KBr to 25 mL of deionized water. Then, solution B was added dropwise to solution A under magnetic stirring. After further stirred for 2 h, the reaction mixture was centrifuged, filtered and dried at 60 °C to obtain AgBr/Ti3C2@TiO2 composite. This sample was denoted as AgBr/Ti3C2@TiO2-10%, for the mass percentage of Ti3C2@TiO2 was accounting for 10% of the sample. Other AgBr/Ti3C2@TiO2 composite samples with different proportions of Ti3C2@TiO2 (10%, 20%, 30%, 40%, 50%, 60%, 70%) were prepared by changing the amount of each sample. The obtained AgBr/Ti3C2@TiO2 samples were denoted as AgBr/Ti3C2@TiO2-x% (x% referred to the mass percentage of Ti3C2@TiO2).
2.5. Characterization
The crystal structure, morphology, and elemental composition were characterized by X-ray diffraction (XRD), scanning electron microscopy (SEM), high-resolution transmission electron microscopy (HRTEM), energy dispersive spectrometer (EDS), X-ray photoelectron spectroscopy (XPS) and Fourier transform infrared spectrometer (FT-IR). An electrochemical workstation equipped with a three-electrode system was used to measure the electrochemical characteristics of the samples.
2.6. Photocatalytic performance evaluation
The photocatalytic performance of AgBr/Ti3C2@TiO2 was evaluated by the photodehydrogenation of 1,4-DHP and photodegradation of TCH under the irradiation of visible light. The light was provided by a 70 W metal halide lamp equipped with a 400 nm cutoff filter. Typically, 50 mg of AgBr/Ti3C2@TiO2 composite was dispersed into 50 mL of 0.1 mM 1,4-DHP or 20 mg L−1 TCH solution. In order to obtain adsorption–desorption equilibrium, the mixture was magnetically stirred for 30 min before illumination. The extent of the reaction was spectroscopically monitored by measuring the residual concentrations of 1,4-DHP and TCH at wavelengths of 374 and 357 nm, respectively.
3. Results and discussion
The XRD patterns of Ti3AlC2, Ti3C2, Ti3C2@TiO2, AgBr and AgBr/Ti3C2@TiO2 composites are shown in Fig. 1. Ti3C2 was obtained by etching Ti3AlC2 with HF, removing Al layer and retaining Ti and C layers, the XRD diffraction pattern of the precursor Ti3AlC2 shows that there was a main diffraction peak at 2θ = 39.3°. After reacting with HF, the diffraction pattern had undergone the following significant changes: firstly, the peak observed at the diffraction angle of 2θ = 39.3° almost disappeared, indicating that the Al layer was successfully removed by etching.31 Secondly, at the low diffraction angle, because of the expansion of the interlayer distance, the peaks at (002) and (004) move to a low angle and become wider and weaker, indicating that Ti3AlC2 has been transformed into Ti3C2.32,33 The characteristic peaks of multilayer Ti3C2 appear at 2θ values of 8.94° (002), 18.29° (006), 27.66° (008), 34.53° (101), 41.78° (105) and 60.62° (110), which are consistent with the literature.34,35 Ti3C2@TiO2 composites were obtained by in situ growth of TiO2 on Ti3C2 after solvothermal treatment of Ti3C2 and isopropanol (IPA) in a reactor. The characteristic peaks at 25.28°, 36.95°, 48.04° and 55.06° were attributed to the (101), (103), (200) and (211) crystal planes of anatase TiO2 (JCPDS no. 21-1272).36 And the characteristic peaks with 2θ values of 8.94°, 18.29°, 27.66°, 34.53° and 60.62° in Ti3C2@TiO2 correspond to the (002), (004), (006), (101) and (110) crystal planes of Ti3C2, respectively. These results indicate that Ti3C2@TiO2 composites were successfully synthesized (Fig. 1A). It can be noted from Fig. 1B that the diffraction peaks of AgBr can be attributed to the face-centered cubic crystal phase of AgBr, and the 2θ values are 26.72° (111), 30.96° (200), 44.34° (220), 52.48° (311), 55.04° (222), 64.47° (400), 71.09° (331), 73.26° (420) and 81.61° (422), respectively.17 It is consistent with the standard data of AgBr (JCPDS no. 06-0438). As for the AgBr/Ti3C2@TiO2 composites, except for the XRD patterns of AgBr, another two peaks appeared at 2θ values of 39.12° and 77.39°, which should be ascribed to the (111) and (311) crystal planes of Ag0 (JCPDS no. 65-2871)33,37 (Fig. 1B). With the increase of Ti3C2@TiO2 content, the diffraction peaks of AgBr in the ternary composites gradually weakened, and the diffraction peaks of (111) and (311) crystal planes of Ag increased. Due to the existence of low-valent Ti with strong reducing activity in Ti3C2 solution, Ag+ can be partially reduced to Ag0.33,38 It is worth noting that the XRD peaks of Ti3C2 and TiO2 were not observed in the XRD patterns of AgBr/Ti3C2@TiO2 composites, which may have resulted from their low degree of crystallization in the composite samples.39,40
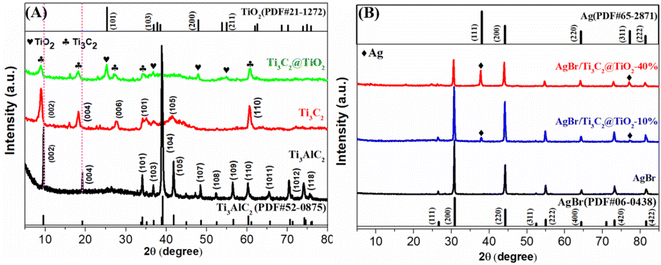 |
| Fig. 1 XRD patterns of Ti3AlC2, Ti3C2 and Ti3C2@TiO2 (A), AgBr and AgBr/Ti3C2@TiO2 composites (B). | |
As shown in Fig. 2A and B, due to the exothermic reaction of HF, Ti3AlC2 transforms from a dense, layered ternary carbide structure to an accordion-like structure of Ti3C2.35 After co-heat treatment of Ti3C2 and isopropanol (IPA) in a reactor, TiO2 nanosheets with a size of about 100 nm were in situ grown on the surface of multilayer Ti3C2 to form Ti3C2@TiO2 composites (Fig. 2C). The AgBr sample shows that irregular polyhedrons with a size of about 2–4 μm were clustered together (Fig. 2D). Compared with pure AgBr (Fig. 2D), AgBr particles in AgBr/Ti3C2@TiO2-10% samples showed better dispersibility and smaller size (Fig. 2E). With the increase of Ti3C2@TiO2 content, the shape of irregular polyhedral AgBr particles becomes more dispersed and the particle size becomes smaller (Fig. 2F). These results indicate that the addition of Ti3C2@TiO2 not only affects the morphology and size of AgBr particles, but also prevents the agglomeration of AgBr polyhedral.
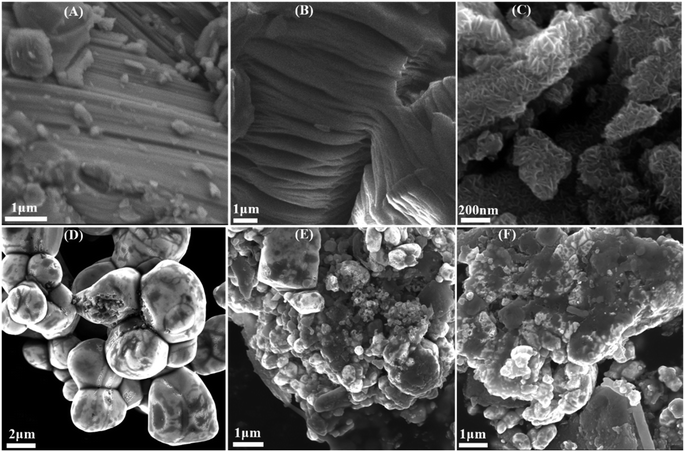 |
| Fig. 2 SEM images of Ti3AlC2 (A), Ti3C2 (B), Ti3C2@TiO2 (C), AgBr (D), AgBr/Ti3C2@TiO2-10% (E), AgBr/Ti3C2@TiO2-40% (F). | |
The microstructure of AgBr/Ti3C2@TiO2-40% composite was further analyzed by TEM and HRTEM. As shown in Fig. 3A and B, AgBr is in close contact with the Ti3C2@TiO2 sheets. According to Fig. 3C, the lattice fringe spacing of 0.189 nm and 0.204 nm correspond to the TiO2 (200) and AgBr (200) crystal planes, respectively. The lattice fringe spacings of 0.189 nm and 0.230 nm belong to TiO2 (200) and Ti3C2 (103) crystal planes, respectively31 (Fig. 3E). Furthermore, the lattice fringes at 0.214 nm and 0.204 nm correspond to Ti3C2 (005) and Ag (200) crystal planes, respectively41 (Fig. 3D and F). The above data show that after solvothermal method and precipitation method, stable heterojunctions are formed between AgBr and TiO2, thus improving the photocatalytic performance of the composites.42
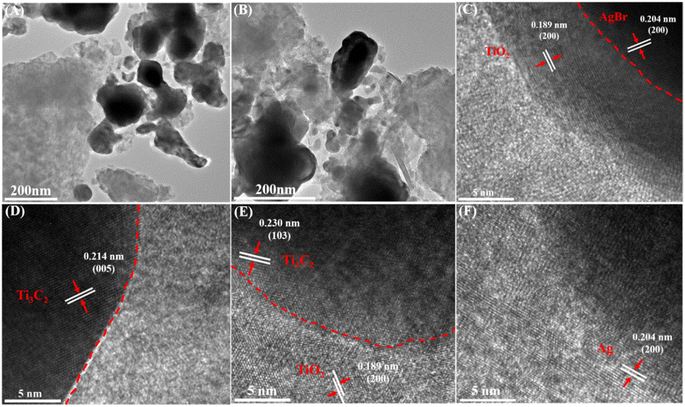 |
| Fig. 3 TEM (A and B) and HRTEM (C, D, E and F) images of AgBr/Ti3C2@TiO2-40% sample. | |
The element distribution and composition of the composites were detected by elemental mapping image. Fig. 4 shows the elemental scanning spectrum of AgBr/Ti3C2@TiO2-10% composites. As can be seen from the figure, the distribution of six elements, namely C, Ag, Br, O, Ti and F. Among them, the element F is the residue of Ti3AlC2 etched by HF, so its content is less.
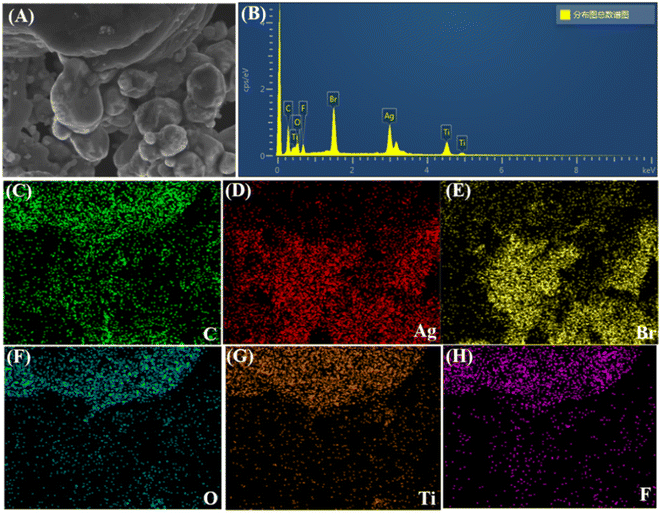 |
| Fig. 4 Selected SEM images (A), the EDS spectrum of the AgBr/Ti3C2@TiO2-10% composite (B), C (C), Ag (D), Br (E), O (F), Ti (G) and F (H) element. | |
The full XPS spectra (Fig. 5A) shows the characteristic peaks of Ti, C, O, Ag and Br in AgBr/Ti3C2@TiO2-40% sample. Fig. 5B–F shows the high-resolution spectra of each element. After carbon correction, curve fitting and background subtraction are carried out. As shown in Fig. 5B, five different peaks can be found in high resolution Ti 2p, of which 455.1 eV, 456.5 eV and 459.0 eV belong to Ti 2p3/2, 464.8 eV and 461.6 eV attribute to Ti 2p1/2. Specifically, the peaks at 456.5 eV, 459.0 eV and 464.8 eV can be belonged to Ti–O bond, and the peaks at 455.1 eV and 461.6 eV indicate the existence of Ti–C bond.43,44 The XPS C 1s spectrum of the composite (Fig. 5C) showed a strong peak at the binding energy of 284.8 eV, corresponding to the C–C bond. Two peaks appear at the binding energies of 281.6 eV and 288.8 eV, corresponding to the Ti–C bond and the C–F bond, respectively.43,45 In addition, the O 1s XPs spectrum showed two distinct peaks at 532.2 eV and 530.3 eV (Fig. 5D), the highest peak at 530.3 eV usually represent the lattice oxygen in TiO2, which also indicated that TiO2 was successfully grown in situ on Ti3C2.31 Fig. 5E shows the Ag 3d spectrum. There are two peaks at around 367.4 eV and 373.4 eV, which can be attributed to Ag 3d5/2 and Ag 3d3/2, respectively. Other studies have shown that the Ag 3d5/2 and Ag 3d3/2 peaks of Ag+ in AgBr usually locate at about 367.37 and 373.48 eV, and the Ag 3d5/2 and Ag 3d3/2 peaks of Ag0 are usually observed at 367.87 and 376.03 eV.14 The present Ag 3d data (367.4 eV for Ag 3d5/2 and 373.4 eV for Ag 3d3/2) should be the superposition of the 3d binding energy of Ag0 and Ag+.46 However, the peaks in Fig. 5E are difficult to divide into two sets of peaks belonging to Ag0 and Ag+, respectively. It may show that Ag0 particles and AgBr particles have a special composite structure, which is different from any of their individual particles.37,46 Fig. 5F shows the Br 3d XPS spectrum, the binding energies of 67.8 eV and 68.8 eV correspond to Br 3d3/2 and Br 3d5/2, respectively.47,48
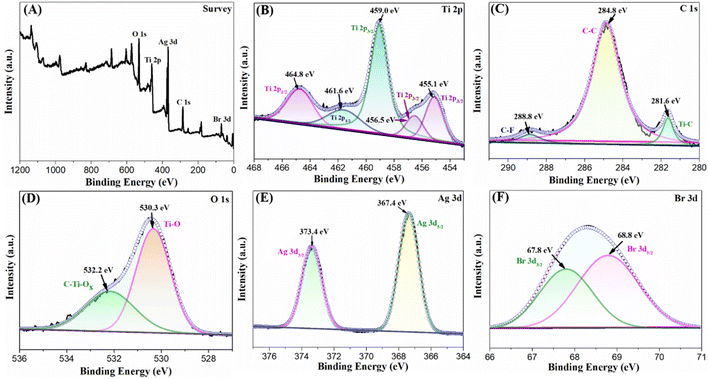 |
| Fig. 5 High-resolution XPS spectra of the AgBr/Ti3C2@TiO2-40%. Full spectrum (A), Ti 2p (B), C 1s (C), O 1s (D), Ag 3d (E) and (F) Br 3d. | |
The functional groups of Ti3C2, Ti3C2@TiO2, AgBr, AgBr/Ti3C2@TiO2-10% and AgBr/Ti3C2@TiO2-40% were detected by FT-IR (Fig. 6). The absorption peaks appeared at 3424 cm−1 and 1631 cm−1, respectively, corresponding to –OH asymmetric stretching and –OH bending vibration of adsorbed water.49 Furthermore, the band at about 2850 cm−1–2924 cm−1 corresponds to O–H stretching vibration, which reflects the existence of –OH.50 In Ti3C2@TiO2, the peaks at 616 cm−1 and 564 cm−1 correspond to Ti–O and Ti–C vibrations respectively,26,51 and the peak at 1393 cm−1 corresponds to C–O or C–N.52 With the increase of Ti3C2@TiO2 content, the two characteristic peaks of Ti3C2 (at 564 cm−1–670 cm−1) gradually increased when it was compounded with AgBr.
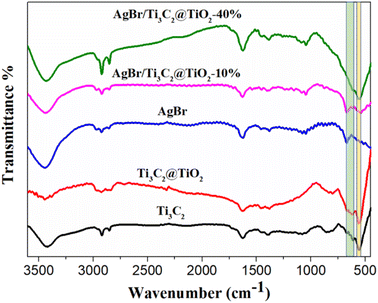 |
| Fig. 6 FT-IR spectra of Ti3C2, Ti3C2@TiO2, AgBr, AgBr/Ti3C2@TiO2-10% and AgBr/Ti3C2@TiO2-40% samples. | |
The photocatalytic performance of the prepared AgBr/Ti3C2@TiO2 composite was tested by the dehydrogenation of 1,4-DHP under visible light irradiation (λ > 400 nm). As shown in Fig. 7A, the absorption peak of 1,4-DHP at 374 nm decreased rapidly, companying with the rapid increase at 280 nm. According to the previous literature, the simultaneous peak intensity decrease at 374 nm and increase at 280 nm indicates the dehydrogenation of 1,4-DHP,53–55 namely the conversion of 1,4-DHP to its pyridine derivatives (Fig. 7A, inset). When AgBr and Ti3C2@TiO2 were used as photocatalyst, the changes of the peak intensity at 374 and 280 nm were also observed, but the changes were much slower (Fig. 7B and C). When no catalyst was added and only irradiated under visible light, the changes of the two peaks were hardly observed. After 9 min irradiation under visible light, the conversion rate of 1,4-DHP were 98.9%, 84.5%, and 21.9% in the presence of AgBr/Ti3C2@TiO2-40%, AgBr, and Ti3C2@TiO2 respectively. The above results show that the as-prepared AgBr/Ti3C2@TiO2 composites have much better photocatalytic performance for 1,4-DHP photodehydrogenation.
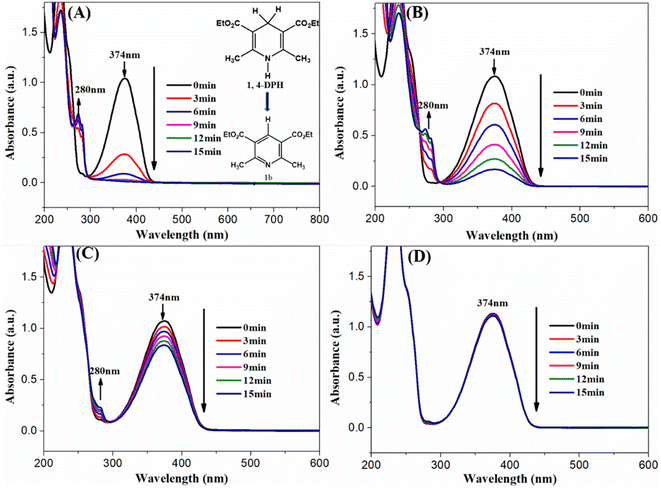 |
| Fig. 7 Under the irradiation of visible light (λ > 400 nm), the ultraviolet-visible spectrum changes of 1,4-DHP solution under different photocatalysts. (A) AgBr/Ti3C2@TiO2-40%, (B) AgBr, (C) Ti3C2@TiO2 and (D) no catalyst. | |
In order to further analyze the influence of Ti3C2@TiO2 content on the photocatalytic properties of AgBr/Ti3C2@TiO2 composites, we prepared a series of AgBr/Ti3C2@TiO2 composites with different Ti3C2@TiO2 content and evaluated their photocatalytic activities by the photodehydrogenation of 1,4-DHP. With the increase of Ti3C2@TiO2 content, the photocatalytic performance of the AgBr/Ti3C2@TiO2 composites first increased and then decreased. All of the composites displayed enhanced photocatalytic ability and the AgBr/Ti3C2@TiO2-40% composite showed the highest photocatalytic activity (Fig. 8A and B). The rate constants of the photocatalyst for 1,4-DHP dehydrogenation are obtained by plotting ln(C/C0) versus illumination time (Fig. 8C) and compared in Fig. 8D. The rate constant of AgBr/Ti3C2@TiO2-40% (0.4044 min−1) is the highest, which is about 3.9 times and 24.5 times as high as that of pure AgBr (0.1035 min−1) and Ti3C2@TiO2 (0.0165 min−1), respectively, further confirming the enhanced photocatalytic ability of AgBr/Ti3C2@TiO2 composites.
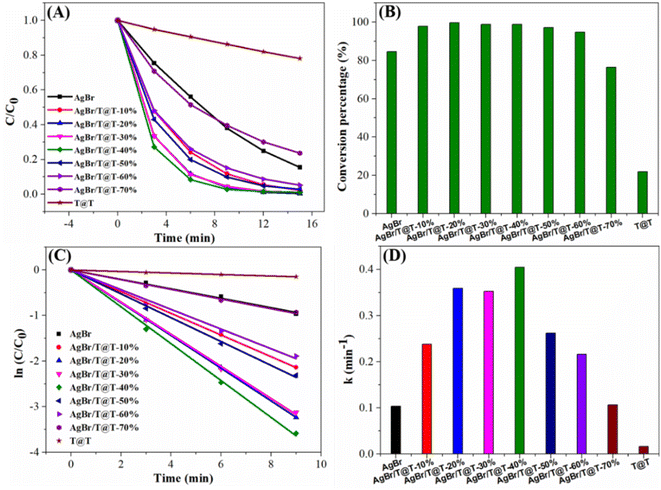 |
| Fig. 8 AgBr, Ti3C2@TiO2 (T@T for short) and AgBr/Ti3C2@TiO2-x% (AgBr/T@T-x% for short) photocatalytic oxidation of 1,4-DHP under visible light: (A) kinetic diagram, (B) degradation efficiency graph, (C) the linear kinetic fitting graph, (D) apparent rate constant. | |
Besides the photodehydrogenation of 1,4-DHP, the AgBr/Ti3C2@TiO2 composites was further applied for the photodegradation of TCH under visible light irradiation (λ > 400 nm). As shown in Fig. 9A, when the catalyst is AgBr/Ti3C2@TiO2-10%, the absorption peak of TCH at 357 nm decreases rapidly, indicating the efficient catalytic degradation of TCH. When AgBr and Ti3C2@TiO2 were used as photocatalyst, the changes of the peak intensity at 357 nm were also observed, but the changes were much slower (Fig. 9B and C). No obvious changes could be observed without any catalyst (Fig. 9D). After 20 min irradiation under visible light, the degradation rate of TCH were 76.5%, 59.6%, and 31.9% in the presence of AgBr/Ti3C2@TiO2-10%, AgBr, and Ti3C2@TiO2, respectively. These results show that the photocatalytic performance of AgBr/Ti3C2@TiO2-10% is much better than that of pure AgBr and Ti3C2@TiO2.
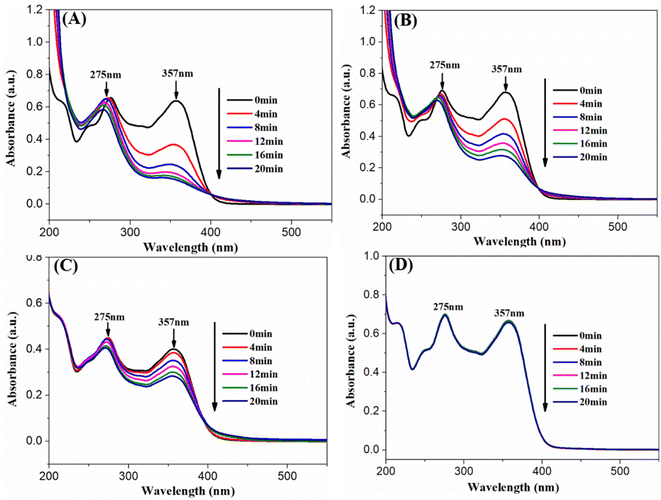 |
| Fig. 9 Under the irradiation of visible light (λ > 400 nm), the ultraviolet-visible spectrum changes of TCH solution under different photocatalysts. (A) AgBr/Ti3C2@TiO2-10%, (B) AgBr, (C) Ti3C2@TiO2 and (D) no catalyst. | |
Similarly, AgBr/Ti3C2@TiO2 composites with different Ti3C2@TiO2 contents were used to degrade TCH. With increase of Ti3C2@TiO2 content, the photodegradation efficiency of AgBr/Ti3C2@TiO2 composites increased first and then decreased. The photo-degradation results of TCH show that under visible light, the conversion rate of AgBr/Ti3C2@TiO2-10% to TCH reaches 76.5% within 20 min, showing the highest photocatalytic activity (Fig. 10A and B). The photodegradation rate constant of TCH (Fig. 10D) is obtained by plotting ln(C/C0) vs. time in Fig. 10C. Among them, the rate constant of AgBr/Ti3C2@TiO2-10% is the highest (0.1027 min−1), which is about 1.9 times and 5.9 times as high as that of pure AgBr (0.0540 min−1) and Ti3C2@TiO2 (0.0174 min−1), respectively. The above data show that AgBr/Ti3C2@TiO2 composite also has good photocatalytic performance for TCH degradation.
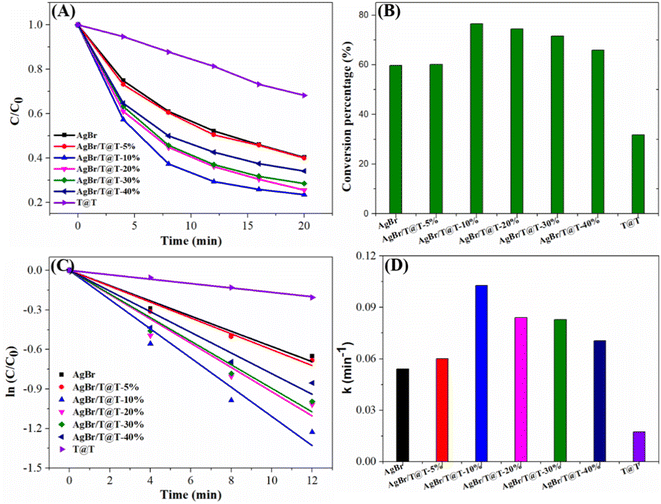 |
| Fig. 10 AgBr, Ti3C2@TiO2 (T@T for short) and AgBr/Ti3C2@TiO2-x% (AgBr/T@T-x% for short) photocatalytic degradation of TCH under visible light: (A) kinetic diagram, (B) degradation efficiency graph, (C) the linear kinetic fitting graph, (D) apparent rate constant. | |
The UV-vis DRS spectra of the materials are shown in Fig. 11A. In comparison with pure AgBr, with an increase of Ti3C2@TiO2 content, the light absorption ability of the composites gradually increases.45,56 The fast charge transfer properties of the prepared materials were analyzed by PL spectroscopy. As shown in Fig. 11B, compared with the pure AgBr, pure Ti3C2 and Ti3C2@TiO2 samples, the emission peaks of the AgBr/Ti3C2@TiO2 composite are of weaker intensity, indicating the beneficial effect of the heterojunction to promote the separation efficiency of photo-induced electron–hole pairs.35,57 Electrochemical impedance spectroscopy (EIS) tests is used to study the electrochemical performance. Fig. 11C shows the Nyquist diagram of EIS of AgBr, Ti3C2@TiO2 and AgBr/Ti3C2@TiO2 photocatalysts. In general, the arc radius in the Nyquist diagram describes the reaction rate at the electrode surface, the smaller the radius, the lower the corresponding electron transfer resistance, the higher the charge transfer and separation efficiency.58 The arc radii of AgBr/Ti3C2@TiO2 composites are much smaller than that of pure AgBr and Ti3C2@TiO2, which indicates that more efficient photogenerated charge carrier's separation, lower internal resistance and faster transfer of charge carriers can occur on the surface of AgBr/Ti3C2@TiO2 composites. These results demonstrate that AgBr is successfully compounded with Ti3C2@TiO2 and has good photogenerated charge carrier separation efficiency. Fig. 11D–F shows the Mott–Schottky curves of AgBr, Ti3C2@TiO2 and AgBr/Ti3C2@TiO2-10% materials. As can be seen from the figure that AgBr is considered as an n-type semiconductor because of its positive slope (Fig. 11D). Although TiO2 is also an n-type semiconductor,59 its Mott–Schottky diagram slope of Ti3C2@TiO2 composite material is negative after in situ growth on Ti3C2 by solvothermal method, and it has the potential of a p-type semiconductor2,23 (Fig. 11E). More importantly, the Mott–Schottky diagram of AgBr/Ti3C2@TiO2-10% composite shows an inverted “V-shape” as shown in Fig. 11F, which indicates that the p–n junction structure has been successfully formed.
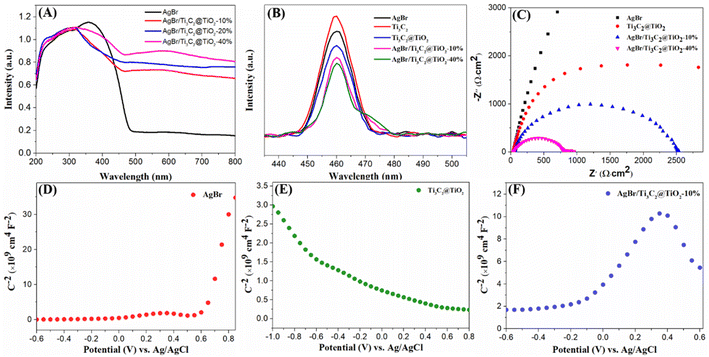 |
| Fig. 11 UV-vis DRS of the as-prepared samples (A), PL spectra (B), EIS Nyquist plots of samples (C), Mott–Schottky plots of AgBr (D), Ti3C2@TiO2 (E) and AgBr/Ti3C2@TiO2-10% (F). | |
Using AgBr/Ti3C2@TiO2 composite catalyst, four repeated experiments were carried out to determine the photodehydrogenation of 1,4-DHP and the photodegradation of TCH separately to evaluate the stability of the prepared photocatalyst. After four cycles, the relative hydrogenation efficiency of 1,4-DHP was 86.2% (Fig. 12A and B), and the relative degradation efficiency of TCH was 93.8% (Fig. 12C and D). Part of the reason for the decrease in efficiency may be due to the loss of the catalyst during the cycle. Therefore, AgBr/Ti3C2@TiO2 composite has good photocatalytic stability and potential practical application.
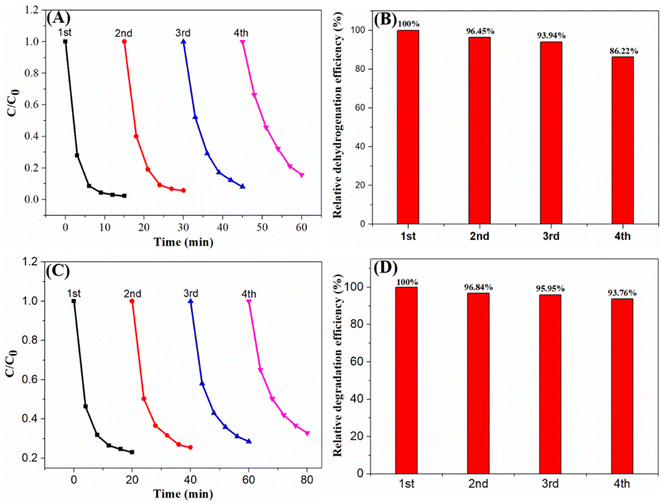 |
| Fig. 12 Cyclic kinetics curve and relative dehydrogenation efficiency of 1,4-DHP by AgBr/Ti3C2@TiO2-40% (A and B); cyclic kinetics curve and relative degradation efficiency of TCH by AgBr/Ti3C2@TiO2-10% (C and D). | |
Active species capture experiment was conducted to explore the three main active species in photocatalytic reaction,60 ammonium oxalate (AO), benzoquinone (BQ) and isopropanol (IPA) were used as scavengers for h+, ˙O2− and ˙OH, respectively.53 As shown in Fig. 13, the addition of IPA slightly decreased the degradation of TCH but has little effect on the dehydrogenation of 1,4-DHP, the addition of AO has a little effect on the degradation of TCH, but slightly decreased the dehydrogenation of 1,4-DHP. When BQ was added to the reaction system, the degradation of TCH was obviously inhibited (Fig. 13A), indicating the major role of ˙O2− in TCH degradation. The addition of BQ has significant influence on absorption spectrum of 1,4-DHP (data not shown), so BQ is not suitable for the detection of ˙O2− radicals in the reaction system of 1,4-DHP. It was generally thought that the dissolved oxygen (O2) in the reaction solution accepted photo-excited electrons from the conduction band of a semiconductor to produced ˙O2− (O2 + e− → ˙O2−).61 Therefore, the removal of O2 from the 1,4-DHP reaction system would affect the photodehydrogenation of 1,4-DHP, if ˙O2− was involved in the dehydrogenation process. With this in mind, we carried out the photodehydrogenation of 1,4-DHP under a nitrogen atmosphere. The dissolved O2 was removed from the reaction by nitrogen bubbling. The removal of O2 apparently suppressed the dehydrogenation of 1,4-DHP (Fig. 13B). This results suggested that ˙O2− was also played important role in 1,4-DHP dehydrogenation, just as in TCH degradation.
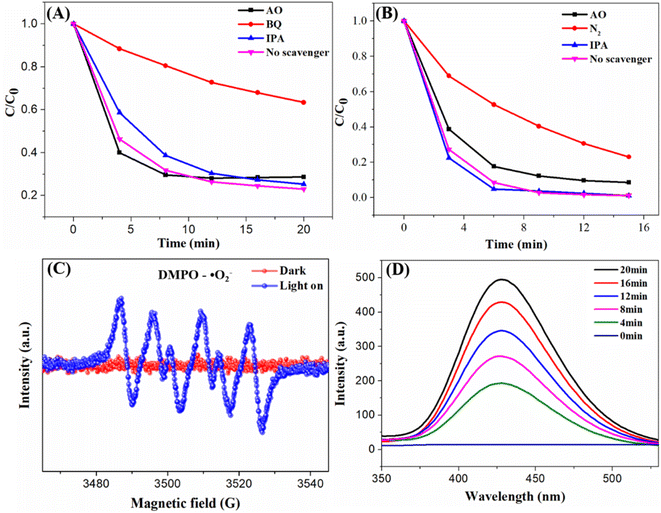 |
| Fig. 13 The effect of certain selected scavengers on the photooxidation of TCH (A) and 1,4-DHP (B) under visible light (λ > 400 nm) irradiation, EPR signals of AgBr/Ti3C2@TiO2 under dark and visible light irradiation (C) and fluorescence spectra of 0.5 mM alkaline terephthalic acid (TA) solution in the presence of AgBr/Ti3C2@TiO2 composite under visible light irradiation (D). | |
EPR technique was employed to further detect the generation of ˙O2− in the AgBr/Ti3C2@TiO2 composite involved photocatalytic system. DMPO was used as a radical trap. As shown in Fig. 13C, the DMPO-˙O2− characteristic peak was observed under visible light irradiation,62 but not observed in dark condition. The production of ˙OH by AgBr/Ti3C2@TiO2 composite during the photocatalysis process was probed by fluorescence technique.63 The fluorescence absorption peak around 425 nm obviously increased with visible-light irradiation time (Fig. 13D), indicating the formation of hydroxyl terephthalic acid (TA-OH), confirmed the generation of ˙OH on the surface of AgBr/Ti3C2@TiO2 composites.53
According to the results of active species capture experiment, EPR trapping experiment and terephthalic acid-based fluorescence experiment, a possible photocatalytic reaction mechanism was proposed (Fig. 14).
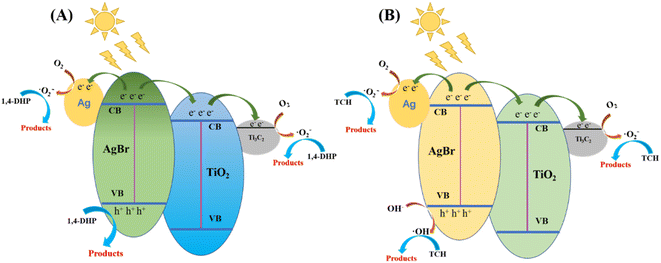 |
| Fig. 14 Hypothetical mechanism diagram of dehydrogenation of 1,4-DHP (A) and photodegradation of TCH (B) by AgBr/Ti3C2@TiO2 composites under visible light (λ > 400 nm). | |
Under the excitation of visible light, AgBr with narrow band gap (2.6 eV) absorbs photons and produces photo-generated electron–hole pairs (eqn (1)). According to theoretical analysis, the conduction band (CB) potential of AgBr is more negative than the CB potential of TiO2 and the Fermi level of Ag0 nanoparticles.64–66 Therefore, the electrons on the CB of AgBr can rapidly transfer to the CB of TiO2 (eqn (2)) or be captured by Ag0 nanoparticles through Schottky barrier (eqn (3)).67 The electrons on the CB of TiO2 can further transfer to Ti3C2 (eqn (4)), since the CB potentials of TiO2 is more negative than the Fermi level of the Ti3C2.30,68,69 Thus, the photoinduced charge carriers on AgBr is efficiently separated, and the photocatalytic activity of AgBr is improved by compositing with Ti3C2@TiO2. The electrons transferred to Ag0 and Ti3C2 are subsequently captured by O2 to produce ˙O2− (eqn (5) and (6)). At the same time, the h+ left on the valence band (VB) of AgBr can convert H2O and OH− (H2O/OH−) to ˙OH (eqn (7)). The produced ˙O2−, ˙OH and h+ may all be utilized as oxidizer for 1,4-DHP dehydrogenation and TCH degradation (eqn (8)).
|
AgBr + hv → AgBr (e− + h+)
| (1) |
|
AgBr (e−) + TiO2 → AgBr + TiO2 (e−)
| (2) |
|
AgBr (e−) + Ag0 → AgBr + Ag0 (e−)
| (3) |
|
TiO2 (e−) + Ti3C2 → TiO2 + Ti3C2 (e−)
| (4) |
|
Ag0 (e−) + O2 → ˙O2− + Ag0
| (5) |
|
Ti3C2 (e−) + O2 → ˙O2− + Ti3C2
| (6) |
|
AgBr (h+) + H2O/OH− → ˙OH + AgBr
| (7) |
|
˙O2−, ˙OH, h+ + 1,4-DHP/TCH → products
| (8) |
It is worth pointing out that the main active species are ˙O2− and h+ for 1,4-DHP dehydrogenation, while ˙O2− and ˙OH for TCH degradation. In other words, the photogenerated h+ in the VB of AgBr can react with H2O and OH− to produce ˙OH, then the generated ˙OH is consumed by the oxidation of TCH in the TCH-catalyst system. But for the 1,4-DHP-catalyst system, the generated ˙OH cannot be consumed by 1,4-DHP, which will influence the separation of photogenerated electron–hole pairs. The transfer of electrons in the CB of AgBr to separate it from the photogenerated holes in the VB of AgBr is more urgent in the 1,4-DHP-catalysts system. Therefore, the introduction of Ti3C2@TiO2 into AgBr is more pronounced to enhance the catalytic activity of AgBr for 1,4-DHP oxidation. This maybe also one of the reasons that the optimal proportion of Ti3C2@TiO2 is different for 1,4-DHP and TCH.
To sum up, under visible light irradiation, The enhanced photocatalytic performance of AgBr/Ti3C2@TiO2 composite should be attributed to the following aspects: (1) the formation of a p–n heterojunction between AgBr and TiO2 and the introduction of Ti3C2 as an electron transport medium and acceptor can rapidly transfer electrons, thus enhancing the separation rate of photo-generated electron–hole pairs; (2) as the size of AgBr particles in the composite decreases, the specific surface area of the composite increases, and the contact area between the interfaces of AgBr, TiO2 and Ti3C2 increases, which also promotes the transfer of photogenerated carriers. (3) Ti3C2 and AgBr has good absorption capacity for visible light, which can increase the utilization of visible light by composite materials.
4. Conclusions
In conclusion, AgBr/Ti3C2@TiO2 composite photocatalyst was prepared by solvothermal and precipitation method and the photocatalytic performance of the composite was evaluated by the photodehydrogenation of 1,4-DHP and photodegradation of TCH under visible light irradiation. The AgBr/Ti3C2@TiO2 composite photocatalyst showed improved photocatalytic performance compared with AgBr and Ti3C2@TiO2. The photocatalytic performance of the AgBr/Ti3C2@TiO2 composite with optimized composition is 3.9 and 24.5 times higher than that of pure AgBr and Ti3C2@TiO2, respectively, for photodehydrogenation of 1,4-DHP and 1.9 and 5.9 times higher than that of pure AgBr and Ti3C2@TiO2 for photodegradation of TCH. The improvement of photocatalytic performance of AgBr/Ti3C2@TiO2 composite is due to the formation of p–n heterojunction structure between AgBr and Ti3C2@TiO2, and the using of Ti3C2 as cocatalyst, which reduced the internal resistance, accelerated charge transfer and improved the separation efficiency of photogenerated carriers. In addition, AgBr/Ti3C2@TiO2 composite photocatalyst also showed good stability in photochemical reaction, indicating that the AgBr/Ti3C2@TiO2 composite has potential application in photooxidation reaction, wastewater treatment and environmental remediation.
Conflicts of interest
There are no conflicts of interest to declare.
Acknowledgements
This work was supported by the Opening Project of Key Laboratory of Green Chemistry of Sichuan Institutes of Higher Education (LZJ2002) and the Open Project of Chemical Synthesis and Pollution Control Key Laboratory of Sichuan Province (CSPC2016-3-2).
References
- X. Shi, B. Ren, X. Jin, X. C. Wang and P. Jin, Metabolic hazards of pharmaceuticals and personal care products (PPCPs) in sewers, J. Hazard. Mater., 2022, 432, 128539 CrossRef CAS PubMed.
- S. Li, Y. Wang, J. Wang, J. Liang, Y. Li and P. Li, Modifying g-C3N4 with oxidized Ti3C2 MXene for boosting photocatalytic U(VI) reduction performance, J. Mol. Liq., 2022, 346, 117937 CrossRef CAS.
- P. Zhang, J. Xu, X.-J. Wang, B. He, S.-Q. Gao and Y.-W. Lin, The Third Generation of Artificial Dye-Decolorizing Peroxidase Rationally Designed in Myoglobin, ACS Catal., 2019, 9, 7888–7893 CrossRef CAS.
- D. Ren, R. Shen, Z. Jiang, X. Lu and X. Li, Highly efficient visible-light photocatalytic H2 evolution over 2D-2D CdS/Cu7S4 layered heterojunctions, Chin. J. Catal., 2020, 41, 31–40 CrossRef CAS.
- C. Bie, H. Yu, B. Cheng, W. Ho, J. Fan and J. Yu, Design, Fabrication, and Mechanism of Nitrogen-Doped Graphene-Based Photocatalyst, Adv. Mater., 2021, 33, 2003521 CrossRef CAS PubMed.
- X. Bao, D. Lu, Z. Wang, H. Yin, B. Zhu, B. Chen, M. Shi, Y. Zhang, Q. Xu, Y. Qin, X. C. Shen and K. Wu, Significantly enhanced photothermal catalytic CO2 reduction over TiO2/g-C3N4 composite with full spectrum solar light, J. Colloid Interface Sci., 2023, 638, 63–75 CrossRef CAS.
- M. Tian, J. Wang, R. Sun, D. Lu, N. Li, T. Liu, M. Yao, G. Zhang and L. Li, Facile synthesis of rod-like TiO2-based composite loaded with g-C3N4 for efficient removal of high-chroma organic pollutants based on adsorption-photocatalysis mechanism, Inorg. Chem. Commun., 2022, 141, 109517 CrossRef CAS.
- Y. Elviera, D. O. B. Yulizar, R. Apriandanu and S. Marcony, Fabrication of novel SnWO4/ZnO using Muntingia calabura L. leaf extract with enhanced photocatalytic methylene blue degradation under visible light irradiation, Ceram. Int., 2022, 48, 3564–3577 CrossRef CAS.
- P. Hemmatpour and A. Nezamzadeh-Ejhieh, A Z-scheme CdS/BiVO4 photocatalysis towards Eriochrome black T: An experimental design and mechanism study, Chemosphere, 2022, 307, 135925 CrossRef CAS PubMed.
- H. Lu, Y. Liu, S. Zhang, J. Wan, X. Wang, L. Deng, J. Kan and G. Wu, Clustered tubular S-scheme ZnO/CdS heterojunctions for enhanced photocatalytic hydrogen production, Mater. Sci. Eng., B, 2023, 289, 116282 CrossRef CAS.
- T. Ren, Y. Dang, Y. Xiao, Q. Hu, D. Deng, J. Chen and P. He, Depositing Ag nanoparticles on g-C3N4 by facile silver mirror reaction for enhanced photocatalytic hydrogen production, Inorg. Chem. Commun., 2021, 123, 108367 CrossRef CAS.
- F. Dong, Z. Wang, Y. Li, W. K. Ho and S. C. Lee, Immobilization of polymeric g-C3N4 on structured ceramic foam for efficient visible light photocatalytic air purification with real indoor illumination, Environ. Sci. Technol., 2014, 48, 10345–10353 CrossRef CAS.
- Z. Miao, G. Wang, X. Zhang and X. Dong, Oxygen vacancies modified TiO2/Ti3C2 derived from MXenes for enhanced photocatalytic degradation of organic pollutants: The crucial role of oxygen vacancy to Schottky junction, Appl. Surf. Sci., 2020, 528, 146929 CrossRef CAS.
- Y. Yan, X. Zhou, P. Yu, Z. Li and T. Zheng, Characteristics, mechanisms and bacteria behavior of photocatalysis with a solid Z-scheme Ag/AgBr/g-C3N4 nanosheet in water disinfection, Appl. Catal., A, 2020, 590, 117282 CrossRef CAS.
- A. Ren, C. Liu, Y. Hong, W. Shi, S. Lin and P. Li, Enhanced visible-light-driven photocatalytic activity for antibiotic degradation using magnetic NiFe2O4/Bi2O3 heterostructures, Chem. Eng. J., 2014, 258, 301–308 CrossRef CAS.
- Y. Shi, Z. Yan, Y. Xu, T. Tian, J. Zhang, J. Pang, X. Peng, Q. Zhang, M. Shao, W. Tan, H. Li and Q. Xiong, Visible-light-driven AgBr–TiO2-Palygorskite photocatalyst with excellent photocatalytic activity for tetracycline hydrochloride, J. Cleaner Prod., 2020, 277, 124021 CrossRef CAS.
- P. Laokul, N. Kanjana, R. Ratchatanee, S. Ruangjan, N. Kotsarn, A. Chingsungnoen and P. Poolcharuansin, Preparation of AgBr decorated ZnO/ZnS nanocomposite for photocatalytic and antibacterial applications, Mater. Chem. Phys., 2023, 295, 127112 CrossRef CAS.
- X. Xue, X. Chen, Z. Zhang, G. Fan and T. Ma, Improved ionic organic pollutant degradation under visible light by Ag SPR-promoted phosphorus-doped g-C3N4/AgBr/Bi2WO6 with excellent charge transfer capacity and high surface area, J. Alloys Compd., 2023, 930, 167457 CrossRef CAS.
- W. Wang, L. Jing, Y. Qu, Y. Luan, H. Fu and Y. Xiao, Facile fabrication of efficient AgBr-TiO2 nanoheterostructured photocatalyst for degrading pollutants and its photogenerated charge transfer mechanism, J. Hazard. Mater., 2012, 243, 169–178 CrossRef CAS PubMed.
- K. Dai, J. Lv, L. Lu, Q. Liu, G. Zhu and D. Li, Synthesis of micro-nano heterostructure AgBr/ZnO composite for advanced visible light photocatalysis, Mater. Lett., 2014, 130, 5–8 CrossRef CAS.
- P. Singh, Sonu, P. Raizada, A. Sudhaik, P. Shandilya, P. Thakur, S. Agarwal and V. K. Gupta, Enhanced photocatalytic activity and stability of AgBr/BiOBr/graphene heterojunction for phenol degradation under visible light, J. Saudi Chem. Soc., 2019, 23, 586–599 CrossRef CAS.
- M. Abou Asi, C. He, M. Su, D. Xia, L. Lin, H. Deng, Y. Xiong, R. Qiu and X.-Z. Li, Photocatalytic reduction of CO2 to hydrocarbons using AgBr/TiO2 nanocomposites under visible light, Catal. Today, 2011, 175, 256–263 CrossRef CAS.
- Y. Cui, Z. Zhang, B. Li, R. Guo, X. Zhang, X. Cheng, M. Xie and Q. Cheng, Ultrasound assisted fabrication of AgBr/TiO2 nano-tube arrays photoelectrode and its enhanced visible photocatalytic performance and mechanism for detoxification of 4-chlorophenol, Sep. Purif. Technol., 2018, 197, 189–196 CrossRef CAS.
- M. Naguib, M. Kurtoglu, V. Presser, J. Lu, J. Niu, M. Heon, L. Hultman, Y. Gogotsi and M. W. Barsoum, Two-dimensional nanocrystals produced by exfoliation of Ti3AlC2, Adv. Mater., 2011, 23, 4248–4253 CrossRef CAS PubMed.
- R. Zhao, M. Wang, D. Zhao, H. Li, C. Wang and L. Yin, Molecular-Level Heterostructures Assembled from Titanium Carbide MXene and Ni–Co–Al Layered Double-Hydroxide Nanosheets for All-Solid-State Flexible Asymmetric High-Energy Supercapacitors, ACS Energy Lett., 2017, 3, 132–140 CrossRef.
- Y. Yang, D. Zhang and Q. Xiang, Plasma-modified Ti3C2Tx/CdS hybrids with oxygen-containing groups for high-efficiency photocatalytic hydrogen production, Nanoscale, 2019, 11, 18797–18805 RSC.
- M. Wu, M. He, Q. Hu, Q. Wu, G. Sun, L. Xie, Z. Zhang, Z. Zhu and A. Zhou, Ti3C2 MXene-Based Sensors with High Selectivity for NH3 Detection at Room Temperature, ACS Sens., 2019, 4, 2763–2770 CrossRef CAS PubMed.
- J. Guo, Q. Peng, H. Fu, G. Zou and Q. Zhang, Heavy-Metal Adsorption Behavior
of Two-Dimensional Alkalization-Intercalated MXene by First-Principles Calculations, J. Phys. Chem. C, 2015, 119, 20923–20930 CrossRef CAS.
- C. Cai, R. Wang, S. Liu, X. Yan, L. Zhang, M. Wang, Q. Tong and T. Jiao, Synthesis of self-assembled phytic acid-MXene nanocomposites via a facile hydrothermal approach with elevated dye adsorption capacities, Colloids Surf., A, 2020, 589, 124468 CrossRef CAS.
- J. Low, L. Zhang, T. Tong, B. Shen and J. Yu, TiO2/MXene Ti3C2 composite with excellent photocatalytic CO2 reduction activity, J. Catal., 2018, 361, 255–266 CrossRef CAS.
- Z. Liu, Y. Zhou, L. Yang and R. Yang, Green preparation of in situ oxidized TiO2/Ti3C2 heterostructure for photocatalytic hydrogen production, Adv. Powder Technol., 2021, 32, 4857–4861 CrossRef CAS.
- B. Tan, Y. Fang, Q. Chen, X. Ao and Y. Cao, Construction of Bi2O2CO3/Ti3C2 heterojunctions for enhancing the visible-light photocatalytic activity of tetracycline degradation, J. Colloid Interface Sci., 2021, 601, 581–593 CrossRef CAS.
- B. Sun, F. Tao, Z. Huang, W. Yan, Y. Zhang, X. Dong, Y. Wu and G. Zhou, Ti3C2 MXene-bridged Ag/Ag3PO4 hybrids toward enhanced visible-light-driven photocatalytic activity, Appl. Surf. Sci., 2021, 535, 147354 CrossRef CAS.
- Y. Cao, L. Yue, Z. Li, Y. Han, J. Lian, H. Qin and S. He, Construction of Sn-Bi-MOF/Ti3C2 Schottky junction for photocatalysis of tetracycline: Performance and degradation mechanism, Appl. Surf. Sci., 2023, 609, 155191 CrossRef CAS.
- H. Wang, R. Zhao, J. Qin, H. Hu, X. Fan, X. Cao and D. Wang, MIL-100(Fe)/Ti3C2 MXene as a Schottky Catalyst with Enhanced Photocatalytic Oxidation for Nitrogen Fixation Activities, ACS Appl. Mater. Interfaces, 2019, 11, 44249–44262 CrossRef CAS PubMed.
- Y. Li, X. Deng, J. Tian, Z. Liang and H. Cui, Ti3C2 MXene-derived Ti3C2/TiO2 nanoflowers for noble-metal-free photocatalytic overall water splitting, Appl. Mater. Today, 2018, 13, 217–227 CrossRef.
- J. Shu, Z. Wang, G. Xia, Y. Zheng, L. Yang and W. Zhang, One-pot synthesis of AgCl@Ag hybrid photocatalyst with high photocatalytic activity and photostability under visible light and sunlight irradiation, Chem. Eng. J., 2014, 252, 374–381 CrossRef CAS.
- G. Zou, Z. Zhang, J. Guo, B. Liu, Q. Zhang, C. Fernandez and Q. Peng, Synthesis of MXene/Ag Composites for Extraordinary Long Cycle Lifetime Lithium Storage at High Rates, ACS Appl. Mater. Interfaces, 2016, 8, 22280–22286 CrossRef CAS PubMed.
- C. Xu, D. Li, H. Liu, D. Wang, X. Liu, S. Lin, Y. Yang, D. Fan and H. Pan, Construction of 1D/0D CdS nanorods/Ti3C2 QDs Schottky heterojunctions for efficient photocatalysis, J. Environ. Chem. Eng., 2023, 11, 109191 CrossRef CAS.
- Y. Wang, X. Wang, Y. Ji, R. Bian, J. Li, X. Zhang, J. Tian, Q. Yang and F. Shi, Ti3C2 MXene coupled with CdS nanoflowers as 2D/3D heterostructures for enhanced photocatalytic hydrogen production activity, Int. J. Hydrogen Energy, 2022, 47, 22045–22053 CrossRef CAS.
- H. Liu, C. Yang, X. Jin, J. Zhong and J. Li, One-pot hydrothermal synthesis of MXene Ti3C2/TiO2/BiOCl ternary heterojunctions with improved separation of photoactivated carries and photocatalytic behavior toward elimination of contaminants, Colloids Surf., A, 2020, 603, 125239 CrossRef CAS.
- X. Liu, D. Zhang, B. Guo, Y. Qu, G. Tian, H. Yue and S. Feng, Recyclable and visible light sensitive Ag–AgBr/TiO2: Surface adsorption and photodegradation of MO, Appl. Surf. Sci., 2015, 353, 913–923 CrossRef CAS.
- K. Huang, C. Lv, C. Li, H. Bai and X. Meng, Ti3C2 MXene supporting platinum nanoparticles as rapid electrons transfer channel and active sites for boosted photocatalytic water splitting over g-C3N4, J. Colloid Interface Sci., 2023, 636, 21–32 CrossRef CAS PubMed.
- W. Li, H. Chen, Y. Liu, T. Cai, W. Dong and X. Xia, A novel Ti3C2 MXene/PDI supramolecules composite with enhanced photocatalytic activities for degradation of tetracycline hydrochloride under visible-light, J. Environ. Chem. Eng., 2022, 10, 107978 CrossRef CAS.
- B. Saini, K. Harikrishna, D. Laishram, R. Krishnapriya, R. Singhal and R. K. Sharma, Role of ZnO in ZnO Nanoflake/Ti3C2 MXene Composites in Photocatalytic and Electrocatalytic Hydrogen Evolution, ACS Appl. Nano Mater., 2022, 5, 9319–9333 CrossRef CAS.
- D. Chen, M. Liu, Q. Chen, L. Ge, B. Fan, H. Wang, H. Lu, D. Yang, R. Zhang, Q. Yan, G. Shao, J. Sun and L. Gao, Large-scale synthesis and enhanced visible-light-driven photocatalytic performance of hierarchical Ag/AgCl nanocrystals derived from freeze-dried PVP-Ag+ hybrid precursors with porosity, Appl. Catal., B, 2014, 144, 394–407 CrossRef CAS.
- Z. Zhang, J. Yu, L. Ma, Y. Sun, P. Wang, T. Wang and S. Peng, Preparation of the plasmonic Ag/AgBr/ZnO film substrate for reusable SERS detection: Implication to the Z-scheme photocatalytic mechanism, Spectrochim. Acta, Part A, 2020, 224, 117381 CrossRef CAS PubMed.
- X. Peng, J. Tian, S. Zhang, W. Xiao, X. Tian, Y. Wang, J. Xue and D. Lei, Z-scheme transfer pathway assisted photoelectrocatalyst Zn2SnO4/rGO/Ag/AgBr for organic pollutants treatment, Colloids Surf., A, 2023, 657, 130552 CrossRef CAS.
- L. Xiu, Z. Wang, M. Yu, X. Wu and J. Qiu, Aggregation-resistant 3D MXene-based architecture as efficient bifunctional electrocatalyst for overall water splitting, ACS Nano, 2018, 12, 8017–8028 CrossRef CAS PubMed.
- N. Liu, N. Lu, H. Yu, S. Chen and X. Quan, Efficient day-night photocatalysis performance of 2D/2D Ti3C2/porous g-C3N4 nanolayers composite and its application in the degradation of organic pollutants, Chemosphere, 2020, 246, 125760 CrossRef CAS PubMed.
- Z. Mao, W. Hao, W. Wang, F. Ma, C. Ma and S. Chen, BiOI@CeO2@Ti3C2 MXene composite S-scheme photocatalyst with excellent bacteriostatic properties, J. Colloid Interface Sci., 2022, 633, 836–850 CrossRef PubMed.
- S. Shen, T. Ke, D. Fang and D. Lin, N and S co-doping of TiO2@C derived from in situ oxidation of Ti3C2 MXene for efficient persulfate activation and sulfamethoxazole degradation under visible light, Sep. Purif. Technol., 2022, 297, 121460 CrossRef CAS.
- X. Tian, H. Wu, X. Hu, Z. Wang, C. Ren, Z. Cheng, L. Dou and Y.-W. Lin, Enhanced photocatalytic performance of ZnO/AgCl composites prepared by high-energy mechanical ball milling, New J. Chem., 2022, 46, 9155–9171 RSC.
- Y. Cheng, L. He, G. Xia, C. Ren and Z. Wang, Nanostructured g-C3N4/AgI composites assembled by AgI nanoparticles-decorated g-C3N4 nanosheets for effective and mild photooxidation reaction, New J. Chem., 2019, 43, 14841–14852 RSC.
- J. Zhang, X. An, N. Lin, W. Wu, L. Wang, Z. Li, R. Wang, Y. Wang, J. Liu and M. Wu, Engineering monomer structure of carbon nitride for the
effective and mild photooxidation reaction, Carbon, 2016, 100, 450–455 CrossRef CAS.
- H. Li, B. Sun, T. Gao, H. Li, Y. Ren and G. Zhou, Ti3C2 MXene co-catalyst assembled with mesoporous TiO2 for boosting photocatalytic activity of methyl orange degradation and hydrogen production, Chin. J. Catal., 2022, 43, 461–471 CrossRef CAS.
- X. Zhang, H. Zhang, J. Yu, Z. Wu and Q. Zhou, Preparation of flower-like Co3O4 QDs/Bi2WO6 p–n heterojunction photocatalyst and its degradation mechanism of efficient visible-light-driven photocatalytic tetracycline antibiotics, Appl. Surf. Sci., 2022, 585, 152547 CrossRef CAS.
- S. Li, L. Shao, Z. Yang, S. Cheng, C. Yang, Y. Liu and X. Xia, Constructing Ti3C2 MXene/ZnIn2S4 heterostructure as a Schottky catalyst for photocatalytic environmental remediation, Green Energy Environ., 2022, 7, 246–256 CrossRef CAS.
- W. Gan, J. Zhang, H. Niu, L. Bao, H. Hao, Y. Yan, K. Wu and X. Fu, Fabrication of Ag/AgBr/TiO2 composites with enhanced solar-light photocatalytic properties, Colloids Surf., A, 2019, 583, 123968 CrossRef CAS.
- B. Zhou, H. Hong, H. Zhang, S. Yu and H. Tian, Heterostructured Ag/g-C3N4/TiO2 with enhanced visible light photocatalytic performances, J. Chem. Technol. Biotechnol., 2019, 94, 3806–3814 CrossRef CAS.
- Y. Ren, D. Guo, Z. Zhao, P. Chen, F. Li, J. Yao, H. Jiang and Y. Liu, Singlet oxygen mediated photocatalytic Antimonite decontamination in water using nanoconfined TiO2, Chem. Eng. J., 2022, 435, 134832 CrossRef CAS.
- H. Sun, C. Zou and W. Tang, Designing double Z-scheme heterojunction of g-C3N4/Bi2MoO6/Bi2WO6 for efficient visible-light photocatalysis of organic pollutants, Colloids Surf., A, 2022, 654, 130105 CrossRef CAS.
- H. Huang, N. Huang, Z. Wang, G. Xia, M. Chen, L. He, Z. Tong and C. Ren, Room-temperature synthesis of carnation-like ZnO@AgI hierarchical nanostructures assembled by AgI nanoparticles-decorated ZnO nanosheets with enhanced visible light photocatalytic activity, J. Colloid Interface Sci., 2017, 502, 77–88 CrossRef CAS PubMed.
- Y. Wang, H. Yang, H. Yun, J. Gao, Y. Liu, M. Zhang, G. He and Z. Sun, Crystallization time-induced microstructural evolution and photoelectrochemical properties of ternary Ag@AgBr/TiO2 nanorod arrays, J. Alloys Compd., 2022, 904, 163370 CrossRef CAS.
- B. Chen, X. Chen, R. Li, W. Fan, F. Wang, B. Mao and W. Shi, Flame Reduced TiO2 Nanorod Arrays with Ag Nanoparticle Decoration for Efficient Solar Water Splitting, Ind. Eng. Chem. Res., 2019, 58, 4818–4827 CrossRef CAS.
- K. Dai, D. Li, L. Lu, Q. Liu, C. Liang, J. Lv and G. Zhu, Plasmonic TiO2/AgBr/Ag ternary composite nanosphere with heterojunction structure for advanced visible light photocatalyst, Appl. Surf. Sci., 2014, 314, 864–871 CrossRef CAS.
- Y. Wan, C. Liang, Y. Xia, W. Huang and Z. Li, Fabrication of graphene oxide enwrapped Z-scheme Ag2SO3/AgBr nanoparticles with enhanced visible-light photocatalysis, Appl. Surf. Sci., 2017, 396, 48–57 CrossRef CAS.
- V. Q. Hieu, T. K. Phung, T. Q. Nguyen, A. Khan, V. D. Doan, V. A. Tran and V. T. Le, Photocatalytic degradation of methyl orange dye by Ti3C2-TiO2 heterojunction under solar light, Chemosphere, 2021, 276, 130154 CrossRef CAS PubMed.
- M. A. Hossen, H. M. Solayman, K. H. Leong, L. C. Sim, N. Yaacof, A. Abd Aziz, L. Wu and M. U. Monir, Recent progress in TiO2-Based photocatalysts for conversion of CO2 to hydrocarbon fuels: A systematic review, Results Eng., 2022, 16, 100795 CrossRef CAS.
|
This journal is © The Royal Society of Chemistry 2023 |