DOI:
10.1039/D3RA02112B
(Paper)
RSC Adv., 2023,
13, 17121-17129
A comparative study on polyaluminum chloride (PACl) and Moringa oleifera (MO) chemically enhanced primary treatment (CEPT) in enhanced biogas production: anaerobic digestion performance and the Gompertz model
Received
31st March 2023
, Accepted 22nd May 2023
First published on 9th June 2023
Abstract
A comparative study was performed to estimate biogas production from sludge produced by organic and inorganic chemically enhanced primary treatments (CEPTs). To this end, the effects of two coagulants, polyaluminum chloride (PACl) and Moringa oleifera (MO), on CEPT and biogas production in anaerobic digestion were surveyed within an incubation period of 24 days. The optimal dosage and pH of PACl and MO were optimized in terms of sCOD, TSS and VS parameters in the CEPT process. Next, the digestion performance of anaerobic digestion reactors fed with sludge obtained from PACl and MO coagulants at a batch mesophilic reactor (37 ± 1 °C) was surveyed from the biogas production, volatile solid reduction (VSR) and Gompertz model. At the optimal conditions (pH = 7 and dosage = 5 mg L−1), the removal efficiency of COD, TSS and VS in CEPT assisted with PACL was 63, 81 and 56%, respectively. Moreover, CEPT assisted with MO led to the removal efficiency of COD, TSS and VS until 55, 68 and 25%, respectively. The highest methane yield (0.598 L gVS removed−1) was obtained in an anaerobic digestion reactor with sludge from the MO coagulant. The anaerobic digestion of CEPT sludge instead of primary sludge resulted in higher sCOD removal efficiency, and 43–50% of sCOD was observed compared with the removal of 32% for the primary sludge. Furthermore, the high coefficient of determination (R2) demonstrated the trustworthy predictive precision of the modified Gompertz model with actual data. The combination of CEPT and anaerobic digestion, especially using natural coagulants, provides a cost-effective and practical way to increase BMP from primary sludge.
1. Introduction
Nowadays, the rapid increase in population and urbanization has led to an increase in sewage production. Therefore, wastewater treatment has been known as a critical need in recent decades. Wastewater treatment recycles and reuses water and deals with energy and resource recovery.1 However, wastewater treatment plants (WWTPs) produce a significant amount of sludge as a byproduct of the treatment process; it is estimated to be 1% of the total raw wastewater.2 Sludge produced from municipal WWTPs commonly contains 60–80% biodegradable organic matter. Sludge management is a complicated and expensive process. However, if managed properly, it can save more than 50% of the cost of a treatment.3 Sludge management and treatment have five main stages: thickening, stabilization, conditioning, dewatering and drying.4 Among these stages, stabilization is a chemical and physical process that leads to the destruction of pathogens, reducing or eliminating the potential for odor production and reducing substances that have the potential to attract carriers.4 There are different methods for sludge stabilization, such as aerobic stabilization, alkaline stabilization, composting and anaerobic stabilization.5–8 One of the disadvantages of the alkaline stabilization method is the increase in mass due to the addition of alkaline substances. In addition, in the aerobic digestion method, the energy consumption of the process is high owing to mixing and oxygen transfer stage.4 However, among these methods, anaerobic digestion has gained much attention owing to the production of renewable energy.9,10 Anaerobic digestion is a biological process for the stabilization of biomass, and it produces biogas rich in methane.11,12 In addition, the anaerobic digestion process is also used to reduce the volume of sludge and pathogens.9 However, the entry of sludge with desirable nutrients and suitable total solids (TS) is one of the main concerns for anaerobic digesters. One of the treatment processes with the production of sludge rich in organic matter to feed anaerobic reactors is the chemically enhanced primary treatment (CEPT) process.13
CEPT is a procedure that involves the addition of coagulants to the initial sedimentation basin to enhance the capture of organic matter into the sludge and optimize the coagulation and flocculation process for better settling results.14 CEPT increases the removal efficiency of suspended solids, phosphorus, and organic matter compared to conventional primary sedimentation; these pollutants are concentrated in the produced sludge, which can be used as a suitable source to increase gas production in anaerobic digestion.15,16 Furthermore, CEPT is highly recommended because of its low energy consumption and easy operation and maintenance; it is economical compared to biological processes.17 Coagulants commonly used in the CEPT process include synthetic and natural coagulants.18 Among the synthetic coagulants, polyaluminum chloride (PACl) is known as the most active type of aluminum coagulant owing to the presence of Al137+ complex in its structure and its high positive charge and strong binding ability.19 In addition, some researchers have indicated that some metal ions resulting from the chemical coagulants in the CEPT process or the addition of PACl lead to the intensification of the process of the anaerobic digestion of sludge, consequently increasing the production of biogas by 10% and 21%.3,20,21 In addition, CEPT improves volatile organic solids by more than 40%.22 Another type of coagulant is a natural coagulant, which is non-toxic, biodegradable and environmentally friendly.23 One of these natural coagulants is Moringa oleifera (MO). The protein responsible for the coagulation activity in MO seeds is observed to have a low molecular weight and cationic peptides.24 In 1995, Gassenschmidt and colleagues studied the composition of amino acids in MO seeds. They discovered that the biopolymer was rich in glutamine, arginine, and proline, along with 60 other residues. The protein was found to have eight positively charged amino acids, including 7 arginine and 1 histidine, and 15 glutamine residues.24 The negatively charged particles in the system are attracted to the cationic charges of the MO protein through electrostatic interactions, resulting in particle collisions and neutralization of charges. This ultimately leads to the coagulation of the suspended particles and the formation of the coagulum.24 In addition, the organic matter in moringa contains more than 40% fat, providing a suitable substrate for biogas production via anaerobic digestion.25 Zhang et al. (2022) employed the CEPT process to produce net energy from sewage sludge through combined CEPT sludge and anaerobic digestion. The authors reported an increased net energy of treatment from 0.06 to 0.36 kW h per cubic meter of treated sewage, which reduces the aeration process.26 The transfer of organic carbon to CEPT sludge can either boost the production of gas in anaerobic digestion or trigger self-sustained combustion during sludge incineration.18 The methane produced from the anaerobic digestion process is used as renewable energy to keep the digesters warm, which are mainly operated at a temperature of 35 °C.27 Feng et al. (2016) also performed anaerobic digestion of the sludge produced from the CEPT process with iron chloride coagulant; the results showed that the amount of biogas produced from the sludge of the CEPT process was about 0.92 m3 kgVS−1.28
The open literature review shows that, so far, all the conducted studies have focused on applying the CEPT process to increase biogas production from anaerobic digestion using chemical coagulants. However, there are few studies on using natural coagulants and comparing them with chemical coagulants. Therefore, this study was developed to investigate the efficiency and comparison of two coagulants, polyaluminum chloride (PACl) and Moringa oleifera (MO) in the CEPT process to measure the production of biogas from the sludge obtained from CEPT in the anaerobic complete mixing digester and to use the Gompertz model to predict biogas production.
2. Materials and methods
2.1. Material characteristics
In this study, municipal wastewater samples were withdrawn from the southern Tehran wastewater treatment plant (STWWTP), located in the south of Tehran, to produce sludge via the CEPT process. Samples of wastewater were taken from the grit chamber discharge and immediately transported to the laboratory in a cold box at a temperature of 4 °C for further examination. The characteristics of the wastewater samples are summarized in Table 1. The inoculum was taken from a full-scale mesophilic anaerobic digester from the same treatment facility. It was incubated at a temperature of 37 ± 1 °C for 10 days to reduce the biogas production from any residual biodegradable organic materials.
Table 1 Characteristics of raw wastewater and inoculum
Parameter |
Raw wastewater |
Inoculum |
pH |
7.2 |
7.4 |
TS (mg L−1) |
777.5 ± 74.24 |
18 630 |
VS (mg L−1) |
360 ± 70.71 |
12 275 |
sCOD (mg L−1) |
201.5 ± 47.37 |
10 401 |
COD (mg L−1) |
504 ± 79.19 |
— |
TSS (mg L−1) |
170 ± 76.36 |
— |
TVFA (mg L−1) |
— |
1351 |
Alkalinity (mg L−1) |
— |
5404 |
VS/DS (%) |
46 |
70 |
2.2. Chemically enhanced primary treatment experiment (CEPT experiment)
To perform the CEPT analysis, a jar test with six 1 L glass containers was used. In this study, two types of coagulants, PACl and Moringa oleifera, were used to produce sludge rich in an organic load before feeding to anaerobic digesters. To this end, different dosages of Moringa oleifera (10–50 mg L−1) and PACl (5–30 mg L−1) were added to jars by considering different pH values (PACl (6–8) and Moringa oleifera (3–9)).29–33 In addition, Zetag8180 cationic polyelectrolyte coagulant aid (1.5 mL L−1) for PACl and ferric chloride coagulant aid (20 mL L−1) for Moringa oleifera were added to jars and mixed in two steps (rapid mixing (250 rpm for 1 min) and slow mixing (60 rpm for 20 min)) and settling (30 min) to determine the optimal condition for further experiments. Following the coagulant jar test, the wastewater supernatant was collected, and the Total Suspended Solids (TSS), Chemical Oxygen Demand (COD), and Volatile Solids (VS) parameters were measured using the procedures described in the standard method for wastewater examination.
2.3. Anaerobic digestion reactors
In this study, to investigate the production of methane gas from the anaerobic digestion of sludge produced by the CEPT process, three batch bioreactors were incubated: (1) fed with sludge from PAC coagulant with cationic polyelectrolyte Zetag8180, (2) fed with sludge from MO coagulant with ferric chloride (FeCl3) and (3) control sludge (C) without adding coagulant. To assess the impact of CEPT on the Biochemical Methane Potential (BMP) in anaerobic digestion, a total of 3 glass anaerobic reactors were used; each has a total volume of 1 L and a working volume of 500 mL. The reactors were placed in a hot water bath. A constant volume of the inoculum (10% of the working volume) was added to each reactor. Before incubation, the batch reactors were purged with pure nitrogen gas for 5 minutes and sealed with gas-tight rubber stoppers to maintain anaerobic conditions. A stable mesophilic temperature of 37 ± 1 °C was maintained through hot water circulation and monitored with a thermometer. Mixing was achieved using a magnetic stirrer rotating at 60 rpm with 5 minutes on and 1 minute off. The volume of methane gas produced in the anaerobic reactors during digestion was measured using the water displacement method.34 A special gas transfer hose was connected to the valve installed on the lid of the bottles, and the end of this hose was placed inside a cylindrical glass column with a capacity of 1 L, which was placed upside down and filled with acidic water. The reason for acidifying the water inside the cylinder is to prevent the dissolution of carbon dioxide in the water. Once a day, the gas outlet valve is opened, and the amount of water displacement was recorded. After forming biogas and measuring its volume, the biogas was injected into a manometer tube filled with a 3 M soda solution. Carbon dioxide and hydrogen sulfide were dissolved in a 3 M soda solution, and the volume of methane gas was calculated by measuring the displacement of the soda column inside the manometer. A diagram of the laboratory setup for the anaerobic reactors is shown in Fig. 1. During the incubation period, other operational parameters, such as soluble Chemical Oxygen Demand (sCOD), Total Volatile Fatty Acids (TVFA), Dry Solids (DS), Volatile Solids (VS), and pH, were analyzed on specific days. All measurements were conducted three times to ensure accuracy.
 |
| Fig. 1 Schematic diagram of a lab-scale batch mode anaerobic digestion reactor. | |
In addition, the methane content in the biogas composition was determined with a biogas analyzer portable (Portable gas detector, 3140 COSMOS, Japan) on a predetermined day within the incubation period.
2.4. Analytical method
Samples of the anaerobic digestate were collected at predetermined intervals during the incubation period to evaluate the performance of the anaerobic digestion reactors. The digestates from the control, PACl, and MO anaerobic reactors were centrifuged (using a K240 centrifuge from Centurion Scientific in the UK) at 3000 rpm for 10 minutes at room temperature.35 The resulting supernatants were then filtered through 0.45 μm pore-sized filters (made of PTFE-L) to measure soluble Chemical Oxygen Demand (sCOD), pH, alkalinity, and Total Volatile Fatty Acids (TVFAs). The concentration of soluble COD was measured using Hach COD high-range vials and the digestion and spectrophotometer method (MN Mano-color UV/vis). The pH of the solutions was determined using a pH meter (WTW inoLab pH 720, Xylem – WTW, Germany). The alkalinity and total volatile fatty acids were measured based on the Nordmann method (DOC316.52.93087) using 0.1 N H2SO4, with endpoints of pH 5.0 and 4.4, respectively, to determine alkalinity (in mg L−1 CaCO3) and TVFA (in mg L−1 CH3COOH).36 DS, VS and TS were measured based on procedures outlined in the standard method for wastewater examination. Additionally, the volatile solid reduction (VSR) was calculated using the Van Kleeck formula:
where Wvsin feed denotes the weight fraction of digested sludge volatile solid content per total dry solids and Wvs in digested sludge denotes the weight fraction of volatile out of digester per total dry solids.
The measurements were repeated three times, and the results are displayed as the average value along with the standard deviation.
2.5. Modeling the biogas production process
The kinetics of biogas production is investigated to describe and evaluate methanogenesis by matching the laboratory data of biogas production to kinetic equations.37,38 Assuming that methane production in the discontinuous bioreactor is a function of bacterial growth,38 the kinetic parameters are adapted to the modified Gompertz model. The modified Gompertz model is defined as follows:
where P is cumulative biogas production (mL gVS−1) at the end of the desired time for anaerobic digestion, i.e. t (days). A is the methane production efficiency (N mL gVS−1), U is the maximum methane production rate (N mL gVS−1 day−1), and e is the natural constant or Nepper number (2.71828). λ is delay phase duration (days), t is incubation time (days), and the regression coefficient and the relative error rate (R2) are used to evaluate the agreement of the laboratory data of methane biogas production with the modified Gompertz model.
2.6. Statistical analysis
A statistical analysis of variance (ANOVA) was employed to evaluate the accuracy of the response value and fitted data on the quadratic model.
3. Results and discussion
3.1. Characteristics of substrates and inoculums
The physico-chemical characteristics of the raw wastewater samples collected at the output of the grit chamber are summarized in Table 1. As shown in Table 1, the total COD and sCOD of the raw wastewater samples were 504 ± 79.19 and 201 ± 47.37 mg L−1, respectively. Notably, VS/TS ratio of raw sewage was determined to be 46%. In addition, detailed information on inoculum characteristics obtained from full-scale anaerobic reactors is summarized in Table 1. Considering the high VS/TS ratio in the inoculum (70%) sampled from anaerobic digesters, it has sufficient microbial inoculum resources to increase methane gas production potential.39
3.2. CEPT experiment
3.2.1. The effect of pH of coagulant on increased sedimentation of organic matter. pH is one of the important factors in most chemical reactions; some coagulants may show different performances under different pH conditions.40 Therefore, providing ideal conditions is necessary to improve the efficiency and effectiveness of the process. Fig. 2(a) and (b) shows the changes in TSS, COD and VS at different pH values (3–9) and (6–8) for MO and PACl, respectively. As shown in Fig. 2(a), the highest removal efficiency of TSS, COD and VS in the effluent of the CEPT process with PACl was observed to be 95, 68, and 65%, respectively, at a pH value of 7. Zarei et al. (2022) reported that the highest TSS (97.34%) and COD (75.76%) removal efficiency in the CEPT process with PACl was observed at pH 7.5, which is consistent with the results obtained in this study.41 The most possible reason for choosing the optimal pH of 7 in this study was the effect of neutral pH on the coagulant performance, proper sedimentation of materials and elements in wastewater and significant removal of TSS. In the case of the CEPT process with Moringa oleifera, the highest removal efficiency of TSS (72%), COD (63%) and VS (35%) in supernatant were observed at pH 7. Boulaadjoul et al. (2018) reported that the addition of 150 mg L−1 of MO at a pH range of 6–8 led to 97% turbidity removal efficiency.42
 |
| Fig. 2 Changes in operational parameters in different pH values of PACl (a) and MO (b) coagulants. | |
Additionally, according to a study conducted by Muthuraman and Sasikala (2014), among natural coagulants (such as M. oleifera, Strychnos potatorum, and Proteus vulgaris), the extract from MO seeds showed the best performance in reducing turbidity, reaching 99% in water containing artificially added kaolin turbidity (at a pH value of 7 and a dosage of 250 mg L−1).43 This is comparable to the results of this study.
3.2.2. Effect of coagulant concentration on increased sedimentation of organic matter. Amongst the important parameters influencing the efficiency of a suitable method for wastewater treatment using coagulants or new processes, such as CEPT, the coagulant dose is of great importance. The production of sludge in the treatment plant and the amount of chemical consumption and coagulant should be reduced as much as possible.4 Moreover, the addition of excessive amounts of coagulant contributes to instability in the settling tank system.44 In this study, dosage variations were investigated for two coagulants, PACl and MO. Fig. 3(a) and (b) shows the variation of TSS, COD and VS in different dosages for MO and PACl. As shown in Fig. 3(a), the highest removal efficiency of COD, TSS and VS parameters in wastewater was observed at a dose of 10 mg L−1 for PACl with values 72, 90 and 60%, respectively. The removal efficiency of COD, TSS and VS parameters in the effluent for PACl at a dose of 5 mg L−1 were 63, 81 and 56%, respectively. As shown in Fig. 3(b), the highest removal efficiency of COD, TSS and VS parameters was observed for MO at a dose of 20 mg L−1 with values of 63, 70 and 30%. The removal efficiency of COD, TSS, and VS parameters in the effluent from the CEPT process was also observed using MO at doses of 10 mg L−1, 55, 68, and 25%, respectively. Considering that the removal of COD, TSS and VS efficiency in doses of 5 and 10 for PACl and doses of 10 and 20 mg L−1 for MO did not have much difference in terms of the sedimentation of wastewater pollutants, in this study, the lower dose of coagulant was chosen owing to economic points. In addition, Yulistri et al. (2016) reported that Moringa oleifera, as a coagulant, improves the quality of wastewater and groundwater. The authors concluded that when the MO concentration exceeded the optimal dose (100 mg L−1), the turbidity increased because all the colloids were neutralized and precipitated at the optimal dose; excess coagulants cause water turbidity; they do not interact with the oppositely charged colloidal particles.45 Therefore, an increase in the coagulant cycle could indicate the remaining turbidity beyond the optimal limit. Thus, the optimal dose in this section was 10 mg L−1 for MO and 5 mg L−1 for PACl. In another study, 10 mg L−1 of PACl and 1.5 mg L−1 of cationic polyelectrolyte zetag8180 were used as coagulants in CEPT, and a combination of CEPT process with a trickling filter led to a reduction of 96% and 89% in COD and TSS parameters, respectively,30 which is similar to our study with the removal efficiency of 72 and 90% of COD and TSS at a dose of 10 mg L−1 of PACL.
 |
| Fig. 3 Changes in COD, TSS, and VS variables in different doses of PACl (a) and MO (b) coagulants. | |
3.3. Biogas and methane production
In this study, the biomethane potential (BMP) of sewage sludge produced from the CEPT process with PACl and MO was examined via the anaerobic digestion process within 24 days of the incubation period. The results of the anaerobic digestion process in terms of daily and total methane production are shown in Fig. 4(a) and (b) for each of the reactors. The cumulative methane production potential obtained in anaerobic reactors fed with sludge produced by the CEPT process with MO and PACl under optimal conditions was observed to be 7622 mL and 5133 mL, respectively. The cumulative methane production for the control reactor was 1688 mL. In contrast, the cumulative methane production in the reactors fed with sludge treated using CEPT with MO and PACl increased 4.51 and 3.04 times, respectively, compared to the control. According to the results, the maximum cumulative production of methane (7622 mL) was observed in the MO, where the reactor fed with sludge was produced from the MO coagulate. In other similar studies, the use of MO coagulants yields good results for biogas and methane production.46 For instance, Yap et al. (2021) reported that Moringa oleifera constitutes 55% of the carbon source and is useful for microbial digestion.46 Therefore, the use of natural coagulants due to the presence of organic substances can operate much better than chemical coagulants and increase gas production. It can be observed as a viable and environmentally friendly option for the treatment of wastewater and the production of renewable energy from sludge in wastewater treatment plants. Fig. 4(b) shows the daily methane production for various anaerobic reactors. The initial methane production in the anaerobic reactors fed with sludge treated with CEPT and PACl or MO coagulants was observed on day 2, while the control reactor had a lag phase of 3 days. The highest daily methane production of 657 mL was observed in the MO reactor on day 10, followed by the PACl reactor with 482 mL on day 10, and the control reactor with 190 mL on day 7. The early high production of methane was due to rapid hydrolysis and the production of fatty acids from easily biodegradable organic materials.35 However, from the 10th day of the incubation period onwards, gas production experienced a decreasing trend due to the reduction of biodegradable organic materials.47,48
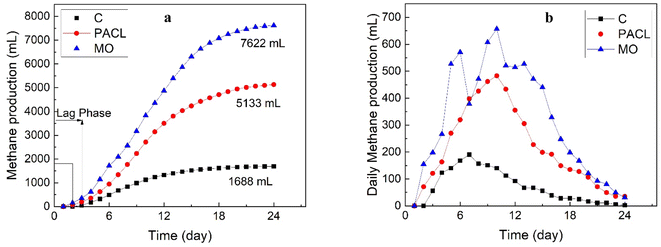 |
| Fig. 4 Process performance of anaerobic digested reactors in terms of daily methane profile (a) and cumulative methane production (b). | |
The methane yield (L gVS removed−1) and methane content for various reactors are summarized in Table 2. The highest methane yield in various anaerobic reactors belongs to the reactor containing sludge from the MO coagulant (0.598 L gVS removed−1), followed by the reactor from the CEPT process with PACl (0.499 L gVS removed−1). The lowest biogas potential (0.408 L gVS removed−1) was observed in the control reactor fed with the sludge obtained from the conventional primary sludge. Koojimn et al. (2017) also showed that the anaerobic digestion of sludge from the CEPT process resulted in a methane yield range of 0.27–0.3 L gVS−1.49 Furthermore, the methane content in different anaerobic digestion reactors was measured to be between 56 and 71%.
Table 2 Comparative assessment of different anaerobic digestion reactors in terms of methane yield and methane content
Parameter |
PACl |
MO |
C |
Methane yield (L gVS removed−1) |
0.499 |
0.598 |
0.408 |
Methane content |
71 ± 2 |
62 ± 2 |
56 ± 3 |
3.4. Digestion performance
3.4.1. Soluble COD reduction. Fig. 5 displays the trend of variations in soluble COD levels in various anaerobic reactors. The laboratory results indicated that the pattern of changes in sCOD was consistent across all reactors; at the beginning of the reactor operation period until the 10th day for PAC and MO sludge and until the 5th day for the control sludge, the sCOD concentration increased following the hydrolysis and acidification processes. After this period, and with the consumption of COD by methanogenic bacteria to produce biogas, sCOD gradually decreased. The highest sCOD (6900 mg L−1) was observed in the reactor fed with MO sludge. The first 10 days showed an increase in the initial sCOD concentration in the reactor using MO sludge, increasing from 1960 mg L−1 to 6900 mg L−1. The removal efficiency of sCOD in anaerobic reactors with CEPT sludge was significantly higher compared to that in the control reactors; the removal efficiency of sCOD in the PACl and MO reactors was 50% and 43%, respectively, and in the reactor with control was 32%. Bezirgianidis et al. (2020)3 investigated the production of biogas from anaerobic sludge reactors by adding 10 mg L−1 of PACl and 1.5 mg L−1 of cationic polyelectrolyte zetag8180, and they reported that the removal efficiency of tCOD was found to be from 46 to 51%, which agrees with the results of our study.
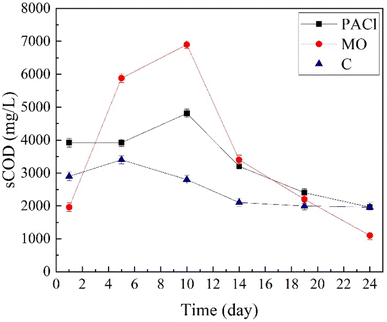 |
| Fig. 5 Changes in sCOD over time in different anaerobic digestion reactors treated with CEPT and the control were studied. | |
3.4.2. Volatile solid (VS) removal. According to previous studies, volatile solid removal (VSR) efficiency is one of the main parameters used to evaluate reactor performance.35 Information related to VSR and the fraction of volatile solids in the initial and final days of the incubation period in different anaerobic digestion reactors is summarized in Table 3. According to Table 3, the highest VSR was observed in the reactor fed with sludge from coagulation enhanced with Moringa oleifera. The most possible reason is that the seeds and extract extracted from MO, in addition to coagulation, flocculation, and precipitation of mineral pollutants, have an organic nature. Jang et al. (2017) also reported a removal percentage of 43.54 ± 70% in the anaerobic digestion of CEPT sludge with the addition of 100 mg L−1 of FeCl3.50
Table 3 Initial and final amounts of volatile solids in different anaerobic digestion reactors using CEPT and control processes
Parameter |
PACl |
MO |
C |
Initial VS/DS (%) |
0.68 |
0.71 |
0.68 |
Final VS/DS (%) |
0.56 |
0.58 |
0.57 |
VSR (%) |
40.1 |
43.59 |
37.6 |
The results obtained from this study are similar to those of Bezirgianidis et al. (2020), who reported that the removal efficiency of volatile suspended solids during the anaerobic digestion of primary sludge without a coagulant was 20.34 ± 3.39%. This was lower compared to the efficiency observed when using CEPT sewage sludge as the feed, which was 35–28%.3
3.4.3. pH and TVFA and alkalinity changes during bioreactor operation. Given that biogas production occurred in a pH range close to neutral and due to the activity of acidic or methanogenic bacteria,51 it is necessary to provide the optimal pH for the reactions. For efficient methanogenesis, the appropriate pH should be maintained in the range of 6.9–7.0; a pH less than 6 and more than 8 has an inhibitory effect on methanogens.52 The variations in the pH of the three anaerobic digestion reactors are shown in Fig. 6(a). As shown in Fig. 6(a), during the first 5 days of the reaction, the pH of the environment decreased, remained stable from the 5th to the 10th day, and then increased slightly from the 10th day to the 24th day. However, the pH of the reaction has often been in the appropriate range (mostly 6.9–7) for the activities of methanogenic and acidic bacteria and microorganisms of anaerobic digestion.35 In addition, the amount of TVFA and its ratio with alkalinity are important to check digester stability. The proportion of TVFA to alkalinity in a linear fashion is crucial for maintaining stability in the anaerobic digestion process. Table 4 summarizes the ratio of TVFA/alkalinity for anaerobic reactors on various days. As depicted in Table 4, the TVFA/alkalinity ratio in different anaerobic sludge digestion reactors was about 0.4, indicating the stability of the anaerobic digestion process.35,53 As demonstrated in Fig. 6(b), TVFA increased after the start of the experimental period until the fifth day, and the highest value (1917 mg L−1) among the reactors belonged to the reactor with MO sludge. The highest TVFA during the first five days indicated the hydrolysis and acidification phases. Following the production of volatile fatty acids, the pH also decreases after the maximum increase in TVFA in the reactors; owing to gas production in the remaining days and entering the methanation stage, the amount of TVFA decreased. In addition, the pH value was maintained in the range of 7.2–7.8 during the entire incubation period, which was suitable for bacterial activity and methane gas production.
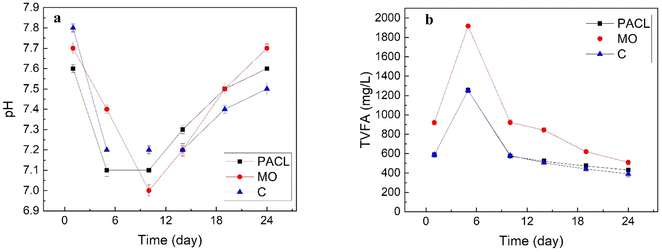 |
| Fig. 6 Changes in pH (a) and TVFA (b) over time in the different anaerobic digestion reactors with and without CEPT assistance. | |
Table 4 Relative changes in total volatile fatty acids to alkalinity in anaerobic reactors
TVFA/alkalinity |
Day (1) |
Day (5) |
Day (10) |
Day (14) |
Day (19) |
Day (24) |
PACl |
0.4 |
0.43 |
0.45 |
0.46 |
0.46 |
0.42 |
MO |
0.41 |
0.45 |
0.53 |
0.53 |
0.5 |
0.46 |
C |
0.42 |
0.47 |
0.48 |
0.48 |
0.43 |
0.4 |
3.5. Kinetic modeling
The modified Gompertz model predicts the amount of gas production using experimental data. This model is proposed to describe bacterial density with respect to time in the growth curve by considering the exponential growth rate and lag phase time. The Gompertz model, with modifications, predicts the rate at which methane gas is produced in an anaerobic reactor by considering the increase in bacterial growth in batch reactors.38,54 Table 5 shows the results obtained from the Gompertz model in terms of mL. The greatest yield of methane gas (8069.2 mL) was generated in the reactor using MO, which indicated the highest level of substrate utilization in the anaerobic reactors. The results obtained from the present study comparable with Ambrose et al. study (2020), who investigated the effect of pre-treated sludge with microwave and H2O2 on the efficiency of single-stage and two-stage anaerobic digesters, so that in this study, the amount of methane production potential in the two-stage reactor, 1305.72 mL and the maximum daily production rate of 117.1 were obtained.55 In this model, the negative values for the lag phase caused by the fitting gas are due to the fitting equation and are usually observed in many studies.56,57 These negative values had no physical period,57 which means that the microorganisms were active and the substrates were easily biodegradable; the lag phase of methanogen growth occurred faster than the time predicted by the Gompertz model.58 In this study, the values obtained for the lag phase were not negative. For the sludge containing MO, PACl, and the control, the values were 3.6, 4, and 3.2, respectively. In the study conducted by Sakaveli et al. (2021), the value of the number obtained for the lag phase was from 2.63 to 0.27, which was below zero and negative on average. The fact that the microorganisms started to become fully active in a short period is probably because the inoculum in this study was obtained from MWTP mesophilic digesters.58 Additionally, the high coefficient of determination demonstrates the trustworthy and precise forecasting abilities of the modified Gompertz model.35 Thus, in this study, the production of methane gas in the PAC sludge reactor follows a perfect linear regression (R2 = 1) from the Gompertz model. The results of Cheng et al.'s study showed a linear regression that is equal to 0.998.59
Table 5 Kinetic parameters of the Gompertz model
Parameters |
Unit |
PACL |
MO |
C |
Predicted methane |
mL |
5253.4 |
8069.2 |
1692.5 |
Maximum CH4 production rate |
mL |
452.1 |
610.5 |
174.4 |
Lag phase |
Day |
4 |
3.6 |
3.2 |
R2 |
— |
1 |
0.999 |
0.998 |
4. Conclusion
In this study, a comprehensive analysis was performed to determine the roles of two coagulants, chemical (PACl) and natural (Moringa oleifera) coagulants, in the CEPT process in anaerobic digestion and methane production. This study indicated that at the optimal pH of 7 for PACl and MO coagulants and at the optimal dosage of 5 mg L−1 for PACl and 10 mg L−1 for MO, the removal efficiency of COD, TSS and VS parameters increased. In addition, the analysis of anaerobic digestion performance showed that the anaerobic digestion of sludge from the CEPT process with Moringa oleifera produced 4.5 times more methane than the control sludge. In addition, the high R2 indicated agreement with the laboratory data based on the changes in the growth of microorganisms in the Gompertz model. Hence, utilizing the CEPT method as a preliminary step and employing Moringa oleifera as a natural coagulant is a favorable and economical approach. However, further studies are required to enhance the amount of methane produced and the economic benefits of using natural coagulants.
Conflicts of interest
The authors declare that they have no known competing financial interests.
Acknowledgements
This research was based on Vida Amoohadi's MSc thesis from Iran University of Medical Sciences. The current project was financially supported by a grant with the number 1400-1-2-20015 from Iran University of Medical Sciences.
References
- H. Guven, R. K. Dereli, H. Ozgun, M. E. Ersahin and I. Ozturk, Prog. Energy Combust. Sci., 2019, 70, 145–168 CrossRef
. - C. Di Iaconi, M. De Sanctis and V. G. Altieri, J. Environ. Manage., 2020, 269, 110714 CrossRef CAS PubMed
. - A. Bezirgiannidis, P. Chatzopoulos, A. Tsakali, S. Ntougias and P. Melidis, Renewable Energy, 2020, 162, 1811–1818 CrossRef CAS
. - Metcalf & Eddy, M. Abu-Orf, G. Bowden, F. L. Burton, W. Pfrang, H. D. Stensel, G. Tchobanoglous, R. Tsuchihashi and AECOM, Wastewater engineering: treatment and resource recovery, McGraw Hill Education, 2014 Search PubMed
. - P. Alvarenga, C. Mourinha, M. Farto, T. Santos, P. Palma, J. Sengo, M.-C. Morais and C. Cunha-Queda, Waste Manage., 2015, 40, 44–52 CrossRef CAS PubMed
. - J. Mendez, B. Jimenez and J. Barrios, Water Sci. Technol., 2002, 46, 139–146 CrossRef CAS PubMed
. - Z. Mucha and J. Mikosz, Int. J. Energy Environ. Eng., 2021, 12, 69–76 CrossRef CAS
. - Q. Zhang, J. Hu, D.-J. Lee, Y. Chang and Y.-J. Lee, Bioresour. Technol., 2017, 243, 1159–1172 CrossRef CAS PubMed
. - P. K. Obulisamy, D. Chakraborty, A. Selvam and J. W. Wong, Environ. Technol., 2016, 37, 3200–3207 CrossRef CAS PubMed
. - A. Pfluger, R. Erickson, G. Vanzin, M. Hahn, J. Callahan, J. Munakata-Marr and L. Figueroa, Environ. Sci.: Water Res. Technol., 2020, 6, 117–131 RSC
. - E. Salminen and J. Rintala, Bioresour. Technol., 2002, 83, 13–26 CrossRef CAS PubMed
. - V. B. Barua and A. S. Kalamdhad, J. Cleaner Prod., 2017, 166, 273–284 CrossRef CAS
. - W. A. Shewa, T. Dong, W. Mu, K. Murray and M. Dagnew, Water Environ. Res., 2020, 92, 359–368 CrossRef CAS PubMed
. - J. Shin, S. Choi, C. M. Park, J. Wang and Y. M. Kim, Chemosphere, 2022, 286, 131569 CrossRef CAS PubMed
. - S. Haydar and J. A. Aziz, J. Hazard. Mater., 2009, 163, 1076–1083 CrossRef CAS PubMed
. - H. Jing, Y. Wang, P.-H. Lee and S.-Y. Leu, Energy Procedia, 2019, 158, 926–933 CrossRef CAS
. - S. Aiyuk, J. Amoako, L. Raskin, A. Van Haandel and W. Verstraete, Water Res., 2004, 38, 3031–3042 CrossRef CAS PubMed
. - W. A. Shewa and M. Dagnew, Sustainability, 2020, 12, 5928 CrossRef CAS
. - N. Li, C. Hu, H. Lan, R. Liu, H. Liu and J. Qu, ACS Sustainable Chem. Eng., 2019, 7, 9544–9552 CrossRef CAS
. - J. Guo, Y. Huang, C. Chen, Y. Xiao, J. Chen and B. Jian, Sci. Rep., 2017, 7, 1–10 CrossRef PubMed
. - X. Hao, J. Wei, M. C. van Loosdrecht and D. Cao, Water Res., 2017, 117, 58–67 CrossRef CAS PubMed
. - C. M. Park and J. T. Novak, Water Sci. Technol., 2013, 68, 2391–2396 CrossRef CAS PubMed
. - A. K. Verma, R. R. Dash and P. Bhunia, J. Environ. Manage., 2012, 93, 154–168 CrossRef CAS PubMed
. - U. Gassenschmidt, K. D. Jany, T. Bernhard and H. Niebergall, Biochim. Biophys. Acta, Gen. Subj., 1995, 1243, 477–481 CrossRef PubMed
. - F. Tambone, M. Pradella, F. Bedussi and F. Adani, Biomass Convers. Biorefin., 2020, 10, 1083–1089 CrossRef CAS
. - H. Zhuang, G.-Y. A. Tan, H. Jing, P.-H. Lee, D.-J. Lee and S.-Y. Leu, Chem. Eng. J., 2022, 431, 133416 CrossRef CAS
. - F. Xu, Y. Li, X. Ge, L. Yang and Y. Li, Bioresour. Technol., 2018, 247, 1047–1058 CrossRef CAS PubMed
. - F. Ju, Y. Wang, F. T. Lau, W. Fung, D. Huang, Y. Xia and T. Zhang, Appl. Microbiol. Biotechnol., 2016, 100, 8975–8982 CrossRef CAS PubMed
. - M. Faraji, A. Ebrahimi, H. Nourmoradi, A. Nikoonahad, A. Abdolahnejad, R. Ghanbari and A. Mohammadi, Desalin. Water Treat., 2019, 139, 297–304 CrossRef CAS
. - A. Bezirgiannidis, A. Plesia-Efstathopoulou, S. Ntougias and P. Melidis, J. Environ. Sci. Health, Part A: Toxic/Hazard. Subst. Environ. Eng., 2019, 54, 1227–1232 CrossRef CAS PubMed
. - N. Shirasaki, T. Matsushita, Y. Matsui, A. Oshiba, T. Marubayashi and S. Sato, Water Res., 2014, 48, 375–386 CrossRef CAS PubMed
. - W. M. Desta and M. E. Bote, Heliyon, 2021, 7, e08451 CrossRef CAS PubMed
. - A. Ndabigengesere and K. S. Narasiah, Environ. Technol., 1998, 19, 789–800 CrossRef CAS
. - S. M. Hallaji, M. Kuroshkarim and S. P. Moussavi, BMC Biotechnol., 2019, 19, 1–10 CrossRef PubMed
. - H. Pasalari, A. Esrafili, A. Rezaee, M. Gholami and M. Farzadkia, Chem. Eng. J., 2021, 422, 130046 CrossRef CAS
. - M. Lili, G. Biró, E. Sulyok, M. Petis, J. Borbély and J. Tamás, Analele Universităţ ii din Oradea, Fascicula Protecţia Mediului, 2011, 17, 713–718 Search PubMed
. - G. K. Latinwo and S. E. Agarry, Int. J. Renewable Energy Dev., 2015, 4, 55–63 CrossRef CAS
. - X. Pan, I. Angelidaki, M. Alvarado-Morales, H. Liu, Y. Liu, X. Huang and G. Zhu, Bioresour. Technol., 2016, 218, 796–806 CrossRef CAS PubMed
. - M. Wojcieszak, A. Pyzik, K. Poszytek, P. S. Krawczyk, A. Sobczak, L. Lipinski, O. Roubinek, J. Palige, A. Sklodowska and L. Drewniak, Front. Microbiol., 2017, 8, 1881 CrossRef PubMed
. - Q. Jin and M. F. Kirk, Front. Environ. Sci., 2018, 6, 21 CrossRef
. - T. Zarei Mahmoudabadi, M. H. Ehrampoush, P. Talebi and H. Eslami, Journal of Rafsanjan University of Medical Sciences, 2022, 21, 533–550 CrossRef
. - S. Boulaadjoul, H. Zemmouri, Z. Bendjama and N. Drouiche, Chemosphere, 2018, 206, 142–149 CrossRef CAS PubMed
. - G. Muthuraman and S. Sasikala, J. Ind. Eng. Chem., 2014, 20, 1727–1731 CrossRef CAS
. - K. Jafari, M. Heidari and O. Rahmanian, Ultrason. Sonochem., 2018, 45, 248–256 CrossRef CAS PubMed
. - I. R. Yuliastri, E. Rohaeti, H. Effendi and L. K. Darusman, IOP Conf. Ser.: Earth Environ. Sci., 2016, 1, 12–33 Search PubMed
. - C. C. Yap, S. K. Loh, Y. J. Chan, C. V. Supramaniam, M. F. Chong, A. C. Soh, L. K. Lim and L. S. Loo, Biomass Bioenergy, 2021, 144, 105885 CrossRef CAS
. - Y. Li, Y. Li, D. Zhang, G. Li, J. Lu and S. Li, Bioresour. Technol., 2016, 217, 50–55 CrossRef CAS PubMed
. - Y. Li, W. Luo, J. Lu, X. Zhang, S. Li, Y. Wu and G. Li, Bioresour. Technol., 2018, 267, 117–125 CrossRef CAS PubMed
. - G. Kooijman, M. De Kreuk and J. Van Lier, Water Sci. Technol., 2017, 76, 1629–1639 CrossRef CAS PubMed
. - H. M. Jang, J. Shin, S. Choi, S. G. Shin, K. Y. Park, J. Cho and Y. M. Kim, Bioresour. Technol., 2017, 244, 433–444 CrossRef CAS PubMed
. - R. Palomo-Briones, J. Xu, C. M. Spirito, J. G. Usack, L. H. Trondsen, J. J. Guzman and L. T. Angenent, Chem. Eng. J., 2022, 137170 CrossRef CAS
. - H. Bouallagui, R. B. Cheikh, L. Marouani and M. Hamdi, Bioresour. Technol., 2003, 86, 85–89 CrossRef CAS PubMed
. - L. Dong, Y. Zhenhong and S. Yongming, Bioresour. Technol., 2010, 101, 2722–2728 CrossRef PubMed
. - P. Tsapekos, P. G. Kougias, S. Kuthiala and I. Angelidaki, Energy Convers. Manage., 2018, 159, 1–6 CrossRef CAS
. - H. W. Ambrose, C. T.-L. Chin, E. Hong, L. Philip, G. Suraishkumar, T. K. Sen and M. Khiadani, Process Saf. Environ. Prot., 2020, 136, 194–202 CrossRef CAS
. - S. Jijai, G. Srisuwan, I. Norli and C. Siripatana, Iranian (Iranica) Journal of Energy & Environment, 2016, 7, 94–101 Search PubMed
. - J. Shen and J. Zhu, Adv. Biotechnol. Microbiol., 2017, 4, 64–74 Search PubMed
. - F. Sakaveli, M. Petala, V. Tsiridis and E. Darakas, Water, 2021, 13, 348 CrossRef CAS
. - Y. Cheng, A. Thebo, Y. Chen, N. Shen, G. Wang and X. Xue, ACS ES&T Engg, 2022, 1523–1530 Search PubMed
.
|
This journal is © The Royal Society of Chemistry 2023 |
Click here to see how this site uses Cookies. View our privacy policy here.