DOI:
10.1039/D3RA02072J
(Paper)
RSC Adv., 2023,
13, 14102-14109
β-Ketoadipic acid production from poly(ethylene terephthalate) waste via chemobiological upcycling†
Received
31st March 2023
, Accepted 2nd May 2023
First published on 9th May 2023
Abstract
The upcycling of poly(ethylene terephthalate) (PET) waste can simultaneously produce value-added chemicals and reduce the growing environmental impact of plastic waste. In this study, we designed a chemobiological system to convert terephthalic acid (TPA), an aromatic monomer of PET, to β-ketoadipic acid (βKA), a C6 keto-diacid that functions as a building block for nylon-6,6 analogs. Using microwave-assisted hydrolysis in a neutral aqueous system, PET was converted to TPA with Amberlyst-15, a conventional catalyst with high conversion efficiency and reusability. The bioconversion process of TPA into βKA used a recombinant Escherichia coli βKA expressing two conversion modules for TPA degradation (tphAabc and tphB) and βKA synthesis (aroY, catABC, and pcaD). To improve bioconversion, the formation of acetic acid, a deleterious factor for TPA conversion in flask cultivation, was efficiently regulated by deleting the poxB gene along with operating the bioreactor to supply oxygen. By applying two-stage fermentation consisting of the growth phase in pH 7 followed by the production phase in pH 5.5, a total of 13.61 mM βKA was successfully produced with 96% conversion efficiency. This efficient chemobiological PET upcycling system provides a promising approach for the circular economy to acquire various chemicals from PET waste.
Introduction
Increasing awareness of environmental issues has inspired an intensified search for novel solutions to counteract the accumulation of plastic waste in landfills and oceans.1,2 One contributor to plastic pollution is polyethylene terephthalate (PET), which is produced at a rate of 70 million tons annually and widely used in single-use packaging. In line with carbon-neutral policies and a circular economy approach, strategies for the upcycling of PET waste have gained increasing attention for their potential in reducing pollution and minimizing carbon dioxide emissions. When estimating the cost of recycled terephthalic acid (rTPA) sourced from conventional chemical recycling process of PET waste, the potential production costs of rTPA would be $1.93 per kg after production of rTPA with 69–83% less greenhouse gas (GHG) emissions but at a higher cost. Hence it is needed for further development in cost reduction. Suggesting further development in cost reduction.3,4
To address the problem, several upcycling methods have been suggested, including chemical, biological, and chemo-biological upcycling. While enzymatic hydrolysis offers a promising biotechnological approach to PET degradation under mild conditions, but it has serious limitations, such as the prerequisite for amorphous or low-crystallinity PET necessary for the proper enzyme activity.5 Recently, a combination of chemical depolymerization of PET and biological upcycling of TPA have been developed to produce value-added chemicals such as protocatechuic acid (PCA), gallic acid, pyrogallol, catechol, muconic acid, and vanillic acid. These chemicals have various industrial applications and can be produced through whole-cell bioconversion.4–8
Chemical depolymerization processes of PET, such as glycolysis, methanolysis, aminolysis, and hydrolysis have all been extensively studied and vary by the type of reagent used.9 Particularly, the hydrolysis process can directly produce TPA by cleavage of ester bonds in the PET chain in acidic or basic aqueous conditions without an organic solvent, thereby increasing the energy economy and allowing its direct utilization as the substrate for bioconversion. Microwave radiation can be further employed in hydrolysis to reduce the thermodynamic kinetic energies in PET depolymerization under mild conditions.4 For being energy effective, the hydrolysis reaction should incorporate a heterogenous catalyst with an active surface group that functions as Lewis/Brønsted acids and bases along with an easily recoverable rigid framework. Accordingly, Amberlyst-15, a sulfonic acid-based styrene-divinylbenzene copolymer, is used to catalyze a variety of acidic reactions such as esterification, phenol alkylation, and condensation, and can also be used for hydrolysis.10 Furthermore, Amberlyst-15's rigid non-corrosive macroporous structure provides physical and chemical stability allowing its recovery from the reaction solution via filtration and centrifugation.11
The biological upcycling process of monomers hydrolyzed from PET can be accomplished using a whole-cell microbial catalyst suitably constructed. Because of its ease of manipulation, culturing, and scalability, Escherichia coli (E. coli) is the most well-established production host strain in the cell factory fields.12 To initiate bioconversion, the substrates should not be toxic to microbial cells and should be able to pass through the cell membrane into the cytoplasmic space in the culture media, under suitable pH conditions. However, the bioconversion of TPA is limited by its potential toxicity which affects biocatalyst growth and metabolism capacity, and by its absolute concentration in reaction media resulting from the low solubility. In a previous study, TPA inhibited bioconversion and reduced the yield of a biocatalytic reaction by over 1 g L−1.5 Therefore, overcoming the TPA-induced inhibition is crucial to develop an efficient biological upcycling process with sustainability.
In this study, we present a chemobiological approach for producing β-ketoadipic acid (βKA) from PET waste. To validate our proposed system for βKA production, we utilized an actual disposable plastic coffee cup to establish proof-of-concept, as experiments conducted solely with reagents would not be sufficient to demonstrate real-world applicability. Our approach involves hydrolysis of PET using Amberlyst-15 (as shown in Fig. 1) under microwave conditions, followed by bioconversion with E. coli strains that overexpress enzymes involved in the βKA synthesis pathway. We chose to use the commercially available and cost-effective acidity catalyst, Amberlyst-15, to showcase the practicality of our system in industrial settings. To develop an efficient biological upcycling process and a TPA fed-batch conversion system, we profiled TPA conversion to identify deleterious factors under flask culturing conditions. Acetic acid accumulation, the critical deleterious factor for conversion, was controlled by deleting the acetate-forming gene (poxB) from the E. coli strain and modulating the dissolved oxygen (DO) level in the bioreactor. Finally, after determining the optimal pH for TPA transportation into the cytoplasm to improve substrate availability and enhance the bioconversion rate, a two-stage fed-batch bioconversion system was successfully developed. The proposed sequential waste PET upcycling composed of industrial catalyst-based PET depolymerization and followed by easily scalable whole-cell catalyst, E. coli strain-based βKA production, would be enabling a plastic circular economy.
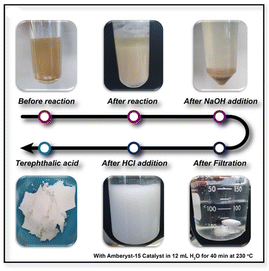 |
| Fig. 1 Schematic illustration of the preparation of TPA from PET using the acidic catalyst, Amberlyst-15. | |
Experimental
Materials
Amberlyst-15 and barium chloride (BaCl2) were purchased from Sigma-Aldrich (St. Louis, MO, USA). Sulfuric acid (H2SO4) was acquired from Samchun Chemicals (Seoul, Korea). E. coli XL1-Blue competent cells were purchased from Stratagene (La Jolla, CA). The genes used for plasmid construction were obtained through gene synthesis by Cure Bio (Seoul, Korea).
Activation of Amberlyst-15
The activation of Amberlyst-15 was performed as previously described, with a slight modification.12 Amberlyst-15 was ground to a fine powder and activated in sulfuric acid for 3 h at 25 °C. The activated Amberlyst-15 was filtered and washed with double distilled water (DIW) several times until no sulfate was detected in the supernatant using a BaCl2 solution.13
Microwave-assisted PET hydrolysis with Amberlyst-15
Microwave-assisted PET hydrolysis was performed using a microwave reaction system (Monowave 400, Anton Paar, Austria) equipped with temperature and pressure sensors. We added 0.1 g of PET powder to the reactor and 12 mL DIW containing 0.1 g of Amberlyst-15. To determine the optimal conditions for PET hydrolysis, we measured the reaction yields at various catalyst amounts (0.01 g, 0.05 g, and 0.1 g) and reaction times (10, 20, 30, 40, and 50 min) at 505 K. After the reaction, the produced TPA was purified using the following method. The solution was filtered to separate ethylene glycol (EG) from the reaction solution, and the solid residues were washed separately with 1 M NaOH to dissolve the produced TPA in the form of disodium-TPA (Na2-TPA). The Na2-TPA was then recrystallized by adding twice the equivalent amount of 2 M HCl solution. The resulting white precipitate was filtered, dried, and the filtered catalysts were reused for the above reaction. The PET conversion rate (%) and TPA yield (%) were calculated as follows:4 |
 | (1) |
|
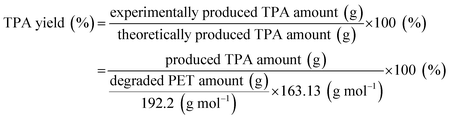 | (2) |
Bacterial strains, plasmids, and culture conditions
All bacterial strains and plasmids used in this study are listed in Table 1. Cloning, whole-cell bioconversion, and fermentation were performed using E. coli XL-1 Blue. E. coli was grown in Luria-Bertani (LB) medium containing 10 g L−1 tryptone, 5 g L−1 yeast extract, and 10 g L−1 NaCl at 37 °C with shaking at 200 rpm.
Table 1 Primers used in this study
Genes |
Forward and reverse primers |
Source strain |
Ref. |
catA |
F: GAATTCATGACCGTGAAAATTTCCCACAC |
Pseudomonas putida KT2440 |
This study |
R: GGTACCTCAGCCCTCCTGCAACGC |
catB |
F: GGTACCTTTCACACAGGAAACAGACCATGACAAGCGTGCTGATTGAAC |
Pseudomonas putida KT2440 |
This study |
R: GGATCCTCAGCGACGGGCGAAGC |
catC/pcaD |
F: GGATCCTTTCACACAGGAAACAGACCATGTTGTTCCACGTGAAGATGAC |
Pseudomonas putida KT2440 |
This study |
R: AAGCTTTTAGTGAGCCAGCAGG |
aroY |
F: GGATCCATGCAGAACCCGATCAACGA |
Klebsiella pneumoniae |
This study |
R: CCTGCAGGTTACTTCTTGTCGCTGAACA |
tphB |
Gene synthesis at IDT |
Comamonas sp. E6 |
1 |
tphAabc |
Gene synthesis at IDT |
Comamonas sp. E6 |
1 |
Construction of E. coli strain for βKA production
Gene cloning was performed using the vectors pKE112, pKM212, and pKA312 which are listed in Table 2. The TPA degradation module (pKM212 vector) was used to convert TPA to TPA-1,2-cis-dihydro diol and further to PCA. The PCA decarboxylation-encoded AroY enzyme for PCA conversion to catechol was inserted into the pKA312 vector.5 The cat operon genes for catechol-1,2-dioxygenase (CatA), muconate cycloisomerase (CatB), and muconolactone D isomerase (CatC), as well as PcaD, were inserted into the pKE112 vector to generate βKA from catechol through meta-cleavage.14 For pKE112-catABCpcaD, DNA fragments from each gene were cloned sequentially using EcoRI, KpnI, BamHI, SbfI, and HindIII restriction enzymes.15 pKM212-tphBAabc was also created by inserting four genes into five restriction enzyme sites. The synthesized aroY was digested with BamHI and SbfI before being ligated into pKA312 and digested with the same restriction enzymes. All cloned DNA was transferred into E. coli XL1-Blue cells and grown in LB medium at 37 °C with shaking at 200 rpm.
Table 2 Characteristics of bacterial strains and plasmids used in this study
Strain and plasmid |
Characteristics of the strain and plasmid |
Ref. |
Strains |
E. coli DH5α |
F− (80d lacZ M15) (lacZYA-argF) U169 hsdR17(r − m+) recA1 endA1 relA1 deoR |
Real biotech |
E. coli XL1-Blue |
recA1 endA1 gyrA96 thi-1 hsdR17 supE44 relA1 lac [FA1proAB lacIqZΔM15 Tn10 (TetR)] |
Stratagene |
E. coli XL1-Blue ΔpoxB |
E. coli XL1-Blue ΔpoxB |
|
E. coli βKA |
E. coli XL1-Blue harboring pKE112CatABCPcaD, pKM212TphABabc and pKA312AroY |
This study |
E. coli βKA (ΔpoxB) |
E. coli XL1-Blue ΔpoxB harboring pKE112CatABCPcaD, pKM212TphABabc and pKA312AroY |
This study |
![[thin space (1/6-em)]](https://www.rsc.org/images/entities/char_2009.gif) |
Plasmids |
pKE112CatABCPcaD |
pKE112; Ptac promoter, Pseudomonas putida KT2440 catABC and pcaD genes, AmpR |
This study |
pKM212TphBAabc |
pKM212; Ptac promoter, Comamonas sp. E6 tphB and tphAabc genes, KmR |
This study |
pKA312AroY |
pKA31212; Ptac promoter, Enterobacter cloacae aroY, CmR |
This study |
Cultivation and fermentation
E. coli XL1-Blue with appropriate plasmids was grown overnight in 2 mL LB medium at 37 °C and 220 rpm. The culture was transferred to a 250 mL baffled flask with 25 mL of minimal medium containing 5 g L−1 glucose and 10 g L−1 glycerol as a complex carbon source and 3 mM TPA as a substrate. Cultivation was carried out for 72 h at 30 °C, with samples taken every 24 h for analysis. Fermentation was carried out in a 2.5 L bioreactor (BioCNS, Daejeon, Korea) with a working volume of 500 mL. A volume of 4 mL of culture was prepared as described, in the baffled flask and added to a minimal medium containing the same concentrations of substrates to obtain an initial optical density (OD) of 30 at 600 nm. Fermentation was carried out at 30 °C and 600 rpm; the agitation speed was adjusted to maintain a level of DO above 30%. When its concentration dropped to 5 g L−1, glycerol was added as part of a solution containing minimal medium, and the pH was adjusted to 5.5 with a solution containing 28% (v/v) ammonia.
Analytical procedures
Attenuated total reflection Fourier transform infrared (ATR-FT-IR) spectra of Amberlyst-15 were recorded before and after the catalytic reaction region within 4000–400 cm−1 using an FTIR spectrometer (Thermo Fisher Scientific Nicolet is50, USA) equipped with a smart iTR diamond/ZnSe ATR accessory. The TPA hydrolysate structure was confirmed with 1H NMR spectroscopy using an AVANCE III 400 MHz spectrometer (Bruker Biospin, Rheinstetten, Germany). A spectrophotometer was used to measure the OD of the cells at 600 nm (Shimadzu, Japan). High-performance liquid chromatography (Agilent Technologies, Santa Clara, CA, USA) was used to determine the concentrations of substrates and products within the culture. TPA levels were determined using a C18 column (Rstech, Daejeon, Korea) at 30 °C with an eluent containing a 9
:
1 mixture of water and acetonitrile and, 0.1% trifluoroacetic acid at a flow rate of 1 mL min−1. TPA was detected using a variable-wavelength detector at 254 nm. At 50 °C, glucose, glycerol, acetic acid, and βKA were separated on an Aminex HPX-87H column (300 × 7.8 mm, Bio-Rad Laboratories, Hercules, CA, USA). The mobile phase was 5 mM sulfuric acid at a flow rate of 0.8 mL min−1, and compounds were detected using a refractive index detector with the following retention times: 6.5 minutes for glucose, 8.3 minutes for βKA, 9.7 minutes for glycerol, and 11.1 minutes for acetic acid.
Results and discussion
Production of TPA by microwave-assisted PET hydrolysis with Amberlyst-15
We chose the PET waste model as a clear plastic disposable coffee cup to verify the ability of our proposed chemobiological PET upcycling system. The coffee cups were cut into small squares with dimensions of 1 × 1 and then grounded using a SPEX 6857D Freezer/Mill to obtain a powdered form for efficient hydrolysis (Fig. S1†). To hydrolyse the grounded PET powder in DI water without any additives under microwave irradiation, we employed the commercial heterogeneous catalyst Amberlyst-15, which has an active surface sulfonate group that promotes acidity (as shown in Fig. 2a). The use of microwave heating during hydrolysis facilitated the transfer of heat to specific regions of PET, thereby accelerating degradation and potentially reducing the required reaction kinetic energy. The yield of TPA from PET was calculated to confirm the feasibility of the method and optimize the reaction conditions using Amberlyst-15. With increasing amounts of catalyst, the corresponding Brønsted acidic sites in the sulfonate group increased the level of hydroxonium ions, which in turn induced the nucleophilic attack of water on PET for depolymerization into TPA (Fig. 2b).4 During the initial 10 min of the reaction, the efficiency of PET hydrolysis was constant; however, after 40 min, it increased linearly in time until it reached saturation at about 100% conversion efficiency. The initial pause in the reaction might be due to the random chain scission of PET, while the participation of depolymerized TPA accelerated the rate of the reaction (Fig. 2c).16 In this study, we investigated the proposed chemobiological upcycling of hydrolysate TPA into high purity βKA. To achieve this, we filtered the solution for ethylene glycol (EG) that had also degraded from the PET granules. NMR spectroscopy confirmed the production of TPA by Amberlyst-15-mediated PET hydrolysis. The hydrolysate showed two main peaks representing aromatic single protons and hydroxyl protons at 8.03 and 13.31 ppm, respectively (Fig. S2†). We found that Amberlyst-15 could be easily recovered from the reaction solution by simple filtration and used up to the 4th cycle with a conversion efficiency of over 80% (Fig. 2d). We compared the FT-IR spectra of Amberlyst-15 at the first and 4th reaction to determine the state of the surface functional group (Fig. 2e). The characteristic peaks of the catalyst after the 4th round of reaction was comparable to those of the pure catalyst, except in the region 3500–3300 cm−1 around the peak at 3425 cm−1 corresponding to the O–H region in the sulfonate group.17 Microwave radiation only affects the energy transfer during PET depolymerization, but has a negligible effect on the functional groups present on the surface of the Amberlyst-15 catalyst until its fourth reusability. To regenerate the sulfonate groups on the outer surface of the catalyst, we treated it with sulfuric acid, which unlocked the sulfonate groups and restored the catalytic efficiency of Amberlyst-15 to its initial state, as shown in Fig. 2e. The recoverable catalytic efficiency of Amberlyst-15 under the microwave irradiation following this simple treatment with sulfuric acid can increase energy efficiency.
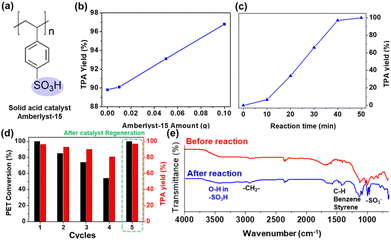 |
| Fig. 2 (a) Chemical structure of Amberlyst-15 (b) and(c) TPA yield over catalyst amount and reaction time, respectively (d) recyclability and efficiency of the catalyst after regeneration by immersion in sulfuric acid (e) FT-IR spectra of Amberlyst-15 before (red) and after (blue) hydrolysis of PET. | |
Development of a biological upcycling process for βKA from TPA
Building on our previous efforts to produce value-added aromatic monomers from PCA as a precursor, we designed artificial pathways composed of a TPA degradation module (pKM212 vector) for PCA production and a βKA synthesis module (pKA312 and pKE112 vector), a three-vector system overexpressing the enzymes needed for an effective PCA conversion (Fig. 3).18 E. coli XL1-Blue expressing these vectors (E. coli βKA hereafter) was used to produce βKA from the substrate hydrolysate TPA in the presence of carbon sources such as glucose for initial cell growth and glycerol to supply redox potential for TPA conversion. The main carbon source used in this study was glycerol, which is a major byproduct of the biodiesel industry. The surplus amount of crude glycerol produced can cause environmental issues, and thus, there is a demand to utilize it efficiently. Flask cultivations of E. coli βKA were repeatedly conducted to identify potentially deleterious factors hindering the biological upcycling process. We found that all enzymes encoded in E. coli βKA were expressed, enabling the successful conversion of TPA into βKA with a 98% molar yield, as shown in Fig. 4. Furthermore, the rate of TPA conversion was boosted in parallel with the activation of glycerol metabolism, indicating a correlation between glycerol consumption and βKA bioconversion. However, glycerol was slowly metabolized and was still present at the end of the conversion reaction. Because glycerol enters E. coli cells passively via the GlpF glycerol facilitator protein, where it undergoes anaerobic metabolism to produce the glycolytic intermediate dihydroxyacetone phosphate, we postulated that the delayed glycerol consumption in the flask cultures of recombinant E. coli could be attributed to the limited oxygen level. From these observations, we hypothesized that the TPA conversion rate could be enhanced by improving glycerol metabolism. Furthermore, the steady accumulation of acetic acid as a byproduct was also observed, its concentration reaching 0.9 mM at the end of the reaction. Acetic acid is a potentially toxic compound, having deleterious effects on cell fitness by decreasing intracellular pH and leading to metabolic perturbation. Thus, its accumulation results in low bioconversion efficiency and should be appropriately regulated.19 Taken together, bioconversion profiling of TPA in E. coli βKA indicated that improving glycerol metabolism as well as properly regulating acetic acid accumulation could be critical in developing a fed-batch conversion system to produce βKA.
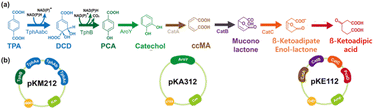 |
| Fig. 3 Schematic illustrations of (a) the bioconversion pathway of TPA to βKA in the E. coli βKA strain and (b) expression vectors to construct βKA biosynthetic pathway from TPA. | |
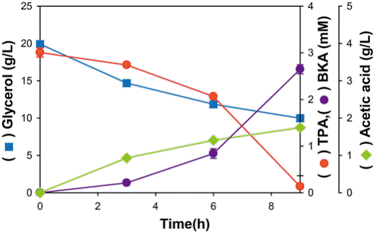 |
| Fig. 4 Flask cultivation profiling by E. coli βKA in a minimal medium containing TPA as the substrate and glucose and glycerol as carbon sources for βKA production containing TPA as the substrate and glucose and glycerol as carbon sources for βKA production. All cultivations were done in duplicates. | |
Improved biological upcycling process of βKA from TPA by controlling deleterious factors
To support oxidative glycerol respiration, bioconversion of TPA into βKA was performed using E. coli βKA in a bioreactor with supplied oxygen (dissolved oxygen, DO level of 30%). As a result, glycerol metabolism was improved, and a total of 36 g L−1 glycerol was consumed, which is 3 times higher than that in the flask cultivation (12 g L−1). However, 4 g L−1 acetic acid was synthesized, which is 5 times higher than that in shaking flask cultivation (Fig. 5a). Additionally, 0.5 mM TPA was not entirely converted into βKA until the end of the bioconversion reaction, even though the initial TPA conversion rate was improved compared to that in the shaking flask cultivation. Under flask cultivation conditions, glycerol metabolism can easily switch from oxidative respiration to fermentative metabolism, resulting in an excess of nicotinamide adenine dinucleotide hydrogen (NADH). Therefore, fermentative glycerol metabolism generates enough NADH pools to support the initial TPA conversion. However, because the bioconversion of TPA into PCA occurs in a self-compensated manner, fermentative metabolism may trigger an intrinsic redox imbalance, leading to the formation of byproducts such as acetic acid to replenish NAD+ content. Consequently, exposure to the toxic organic acid could deteriorate cell fitness and hinder bioconversion. We hypothesized that improved glycerol metabolism in the bioreactor induced the excessive formation of pyruvate or acetyl-CoA, which are precursors of acetic acid, leading to increased acetic acid formation. The accumulated acetic acid negatively affected the bioconversion of TPA into βKA. Therefore, they deleted the acetic acid-forming gene to regulate the acetic acid formation and improve TPA bioconversion into βKA. E. coli strains have three independent acetate-producing pathways: phosphotransacetylase (Pta), acetate kinase (AckA), acetyl-CoA synthetase (Acs), and pyruvate oxidase (PoxB). In the E. coli strain, PoxB only catalyzes the irreversible conversion of pyruvate into acetic acid, unlike the other acetic acid-forming genes. Thus, the poxB gene was deleted to regulate acetic acid production and improve the efficacy of βKA production, and E. coli βKA (ΔpoxB) was further constructed. In the presence of E. coli βKA (ΔpoxB) at a DO level of 30%, TPA bioconversion led to a dramatically reduced acetic acid accumulation–below 0.94 g L−1, which was 4.2-fold lower than that measured when using E. coli βKA (Fig. 5b). In addition, E. coli βKA (ΔpoxB) improved bioconversion, as 3.26 mM βKA was synthesized from TPA with a 100% molar conversion yield. Thus, deletion of poxB gene efficiently regulated the acetic acid metabolism and reduced its accumulation enhancing bioconversion of TPA into βKA. The steady increase in cell growth and rates of glycerol consumption during bioconversion can be regarded as positive parameters indicating that E. coli βKA (ΔpoxB) could be applied for fed-batch bioconversion to maximize the production of βKA. However, the slow TPA conversion rate can be regarded as a negative parameter of the fed-batch bioconversion of TPA into βKA, indicating that TPA was not participating in the TPA conversion owing to poor transportation into the cytoplasmic space.
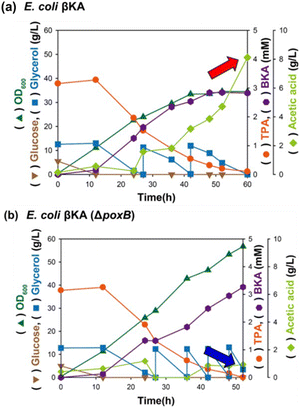 |
| Fig. 5 Bioconversion of TPA into βKA in (a) E. coli βKA and (b) E. coli βKA (ΔpoxB) at a 30% DO level with stepwise glycerol feeding. The red and blue arrows indicate the accumulation of acetic acid, respectively. All fermentations were done in duplicates. | |
βKA production enhancement by two-stage fed-batch bioconversion
In neutral pH (≈7), optimal for favourable bacterial growth, charge repulsion might occur between the aromatic carboxylic groups in the substrate and phosphate groups in the outer membrane of Gram-negative bacteria.21,24 TPA, one of the aromatic carboxylates, exists as a negatively charged ion; thus, the bacterial membrane may prevent its diffusion through the surface of E. coli.22,23 Instead, TPA uptake is known to occur in a moderate acid condition (<6) because carboxylic groups protonate allowing the passive diffusion of TPA through the bacterial membrane.20 Therefore, considering the final pH of 5.5 at the end of the flask cultivation shown in Fig. 4 is consistent with the conditions required for maintaining E. coli strain viability, we designed a two-stage bioconversion process. Initially, appropriate E. coli βKA (ΔpoxB) was grown at pH 7 to express the relevant enzyme for the bioconversion of TPA into βKA. Then, pH was shifted from 7 to 5.5 to increase protonated TPA in reaction media and efficiently convert it to βKA.20 At pH 5.5, the initial 3.2 mM TPA conversion was terminated within 24 h, which was 2.1-fold faster than the sole conversion stage occurring at pH 7 (Fig. 6). Based on the increased reaction rate, TPA stepwise feeding was conducted using E. coli βKA (ΔpoxB). As a result, from a total TPA amount of 14.13 mM with stepwise feeding of TPA, we produced 13.61 mM βKA; this corresponds to a 96% conversion rate at the end of fermentation. As a result of stepwise feeding of TPA, a total amount of 14.13 mM TPA was used to produce 13.61 mM βKA, which corresponds to a 96% conversion rate at the end of fermentation. In addition, the two-stage fed-batch bioconversion system presented in this study exhibited comparable cell density (OD600 = 51) to that used at pH 7 (OD600 = 56). Collectively, our findings indicate that we have successfully constructed a chemobiological system for hydrolysing PET waste using Amberlyst-15 and biologically converting TPA into βKA. The synergetic effect of polar sulfonate groups producing hydrogen ions and the non-corrosive matrix of Amberlyst-15 was clearly demonstrated in the high catalytic activity and reusability for producing the virgin monomeric component of actual plastic cups, TPA. Subsequently, TPA was used as a sequential substrate to produce βKA with high conversion efficiency using a recombinant E. coli strain with deletion of the related byproduct-producing gene under a two-stage fed-batch bioconversion process. However, the inherent issue of insufficient absolute glycerol utilization suggests that further research on glycerol utilization pathways in E. coli is required to enhance βKA production titers to levels that are suitable for industrial applications.
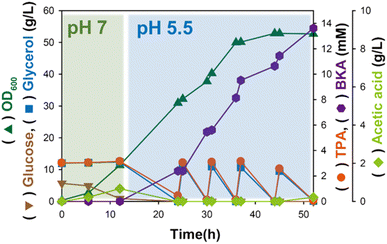 |
| Fig. 6 Two-stage fed-batch bioconversion of TPA into βKA in E. coli βKA (ΔpoxB) under optimized conditions (pH 5.5 and 30% DO level) with stepwise TPA and glycerol feeding. Fed-batch fermentation was carried out once. | |
Conclusions
In this study, we report a chemobiological upcycling strategy to produce βKA from PET waste. PET depolymerization into the TPA substrate was achieved by microwave-assisted hydrolysis with the heterogeneous acid catalyst Amberlyst-15. The sulfonate group of Amberlyst-15 was easily recovered by sulfuric acid treatment and showed 97% catalytic efficiency for TPA conversion. The fed-batch bioconversion of TPA into βKA was successfully developed by regulating acetic acid accumulation and applying two-stage bioconversion. The engineered E. coli (E. coli βKA (ΔpoxB)) developed in this study enabled the production of 13.61 mM βKA with a 96% conversion rate. Despite the successful development of the bioconversion system, several issues should be considered. First, the development of the bioconversion system may require operating high concentration TPA by chassis engineering, such as adaptive laboratory evolution. Next, introducing an efficient TPA transportation system, such as the TPA transporter system of TPA-degrading bacteria Comamonas sp. E6 may be also necessary. The proposed chemobiological system for the valorization of PET waste provides an innovative strategy to reduce the overall environmental impact of plastic-based industries by energy-efficient bioconversion.
Author contributions
H. G. C., H. T. K., and J. C. J. designed the study. S. S. L., M. H. R., M. S. K. H. M. S., B. H. S., E. C. S., and Y. J. J. performed experiments on the chemobiological process for the production of βKA. S. M. Y. and S. J. P guided experiments and data interpretation. S. M. Y., H. T. K. and H. G. C. wrote the manuscript.
Conflicts of interest
The author declares no conflict of interest.
Acknowledgements
This research was supported by the Cooperative Research Program for Agriculture Science and Technology Development (PJ01708201 and PJ01708204) through the Rural Development Administration and the National Research Foundation of Korea (2022R1A4A1033015).
References
- M. J. Kang, H. T. Kim, M.-W. Lee, K.-A. Kim, T. U. Khang, H. M. Song, S. J. Park, J. C. Joo and H. G. Cha, Green Chem., 2020, 22, 3461–3469 RSC
. - Y. Peng, P. Wu, A. T. Schartup and Y. Zhang, Proc. Natl. Acad. Sci. U. S. A., 2021, 118, e2111530118 CrossRef CAS PubMed
. - Y. Luo, E. Selvam, D. G. Vlachos and M. Ierapetritou, ACS Sustainable Chem. Eng., 2023, 11(10), 4209–4218 CrossRef CAS
. - A. Singh, N. A. Rorrer, S. R. Nicholson, E. Erickson, J. S. DesVeaux, A. F. T. Avelino, P. Lamers, A. Bhatt, Y. Zhang, G. Avery, L. Tao, A. R. Pickford, A. C. Carpenter, J. E. McGeehan and G. T. Beckham, Joule, 2021, 5(9), 2479–2503 CrossRef CAS
. - N. F. S. Khairul Anuar, F. Huyop, G. Ur-Rehman, F. Abdullah, Y. M. Normi, M. K. Sabullah and R. Abdul Wahab, Int. J. Mol. Sci., 2022, 23, 12644 CrossRef CAS PubMed
. - H. T. Kim, M. Hee Ryu, Y. J. Jung, S. Lim, H. M. Song, J. Park, S. Y. Hwang, H.-S. Lee, Y. J. Yeon, B. H. Sung, U. T. Bornscheuer, S. J. Park, J. C. Joo and D. X. Oh, ChemSusChem, 2021, 14, 4251–4259 CrossRef CAS PubMed
. - J. Mudondo, H.-S. Lee, Y. Jeong, T. H. Kim, S. Kim, B. H. Sung, S.-H. Park, K. Park, H. G. Cha, Y. J. Yeon and H. T. Kim, J. Microbiol. Biotechnol., 2023, 33, 1–14 CrossRef CAS PubMed
. - H. T. Kim, J. K. Kim, H. G. Cha, M. J. Kang, H. S. Lee, T. U. Khang, E. J. Yun, D.-H. Lee, B. K. Song, S. J. Park, J. C. Joo and K. H. Kim, ACS Sustainable Chem. Eng., 2019, 7, 19396–19406 CrossRef CAS
. - D. H. Kim, D. O. Han, K. In Shim, J. K. Kim, J. G. Pelton, M. H. Ryu, J. C. Joo, J. W. Han, H. T. Kim and K. H. Kim, ACS Catal., 2021, 11, 3996–4008 CrossRef CAS
. - H. Kim, S. Yang and D. H. Kim, Environ. Res., 2020, 187, 109667 CrossRef CAS PubMed
. - M. J. Kang, H. J. Yu, J. Jegal, H. S. Kim and H. G. Cha, Chem. Eng. J., 2020, 398, 125655 CrossRef CAS
. - D. Yang, S. Y. Park, Y. S. Park, H. Eun and S. Y. Lee, Trends Biotechnol., 2020, 38, 745–765 CrossRef CAS PubMed
. - H. Zhang, F. Tian, L. Xu, R. Peng, Y. Li and J. Deng, Chem. Eng. J., 2020, 388, 124214 CrossRef CAS
. - Y. Tian, H. Shen, Q. Wang, A. Liu, W. Gao, X.-W. Chen, M.-L. Chen and Z. Zhao, Anal. Chem., 2018, 90, 7843–7847 CrossRef CAS PubMed
. - S. Vasileiadis, C. Perruchon, B. Scheer, L. Adrian, N. Steinbach, M. Trevisan, P. Plaza-Bolaños, A. Agüera, A. Chatzinotas and D. G. Karpouzas, Environ. Microbiol., 2022, 5105–5122 CrossRef CAS PubMed
. - J. E. Yang, J. W. Kim, Y. H. Oh, S. Y. Choi, H. Lee, A. R. Park, J. Shin, S. J. Park and S. Y. Lee, Biotechnol. J., 2016, 11, 1572–1585 CrossRef CAS PubMed
. - V. Štrukil, ChemSusChem, 2021, 14, 330–338 CrossRef PubMed
. - L. Zhong, A. Tang, P. Yan, J. Wang, Q. Wang, X. Wen and Y. Cui, J. Colloid Interface Sci., 2019, 537, 450–457 CrossRef CAS PubMed
. - A. Z. Werner, R. Clare, T. D. Mand, I. Pardo, K. J. Ramirez, S. J. Haugen, F. Bratti, G. N. Dexter, J. R. Elmore, J. D. Huenemann, G. L. Peabody, C. W. Johnson, N. A. Rorrer, D. Salvachúa, A. M. Guss and G. T. Beckham, Metab. Eng., 2021, 67, 250–261 CrossRef CAS PubMed
. - N. I. Kuznetsova, L. I. Kuznetsova, O. A. Yakovina, V. N. Zudin, B. S. Bal'zhinimaev, A. Bhattacharyya and J. T. Walenga, Ind. Eng. Chem. Res., 2020, 59, 1038–1044 CrossRef CAS
. - I. Pardo, R. K. Jha, R. E. Bermel, F. Bratti, M. Gaddis, E. McIntyre, W. Michener, E. L. Neidle, T. Dale, G. T. Beckham and C. W. Johnson, Metab. Eng., 2020, 62, 260–274 CrossRef CAS PubMed
. - Z. Yang, D. Saeki, R. Takagi and H. Matsuyama, J. Membr. Sci., 2020, 595, 117529 CrossRef CAS
. - M.-L. Chen, X. Tang, T.-H. Lu, X.-Q. Zhan and Z.-H. Zhou, J. Coord. Chem., 2019, 72, 1547–1559 CrossRef CAS
. - S.-M. You, K.-B. Jeong, K. Luo, J.-S. Park, J.-W. Park and Y.-R. Kim, Anal. Chim. Acta, 2021, 1151, 338252 CrossRef CAS PubMed
.
Footnote |
† Electronic supplementary information (ESI) available: Image of plastic sample, NMR of TPA from PET hydrolysis details. See DOI: https://doi.org/10.1039/d3ra02072j |
|
This journal is © The Royal Society of Chemistry 2023 |
Click here to see how this site uses Cookies. View our privacy policy here.