DOI:
10.1039/D3RA01989F
(Paper)
RSC Adv., 2023,
13, 27088-27105
Supramolecular Cu(II) nanoparticles supported on a functionalized chitosan containing urea and thiourea bridges as a recoverable nanocatalyst for efficient synthesis of 1H-tetrazoles†
Received
26th March 2023
, Accepted 16th August 2023
First published on 11th September 2023
Abstract
A cost-effective and convenient method for supporting of Cu(II) nanoparticles on a modified chitosan backbone containing urea and thiourea bridges using thiosemicarbazide (TS), pyromellitic dianhydride (PMDA) and toluene-2,4-diisocyanate (TDI) linkers was designed. The prepared supramolecular (CS–TDI–PMDA–TS–Cu(II)) nanocomposite was characterized by using Fourier-transform infrared (FT-IR) spectroscopy, field emission scanning electron microscopy (FESEM), thermogravimetry/differential thermogravimetry analysis (TGA/DTA), energy-dispersive X-ray spectroscopy (EDS), EDS elemental mapping and X-ray diffraction (XRD). The obtained supramolecular CS–TDI–PMDA–TS–Cu(II) nanomaterial was demonstrated to act as a multifunctional nanocatalyst for promoting of multicomponent cascade Knoevenagel condensation/click 1,3-dipolar azide–nitrile cycloaddition reactions very efficiently between aromatic aldehydes, sodium azide and malononitrile under solvent-free conditions and affording the corresponding (E)-2-(1H-tetrazole-5-yl)-3-arylacrylenenitrile derivatives. Low catalyst loading, working under solvent-free conditions and short reaction time as well as easy preparation and recycling, and reuse of the catalyst for five consecutive cycles without considerable decrease in its catalytic efficiency make it a suitable candidate for the catalytic reactions promoted by Cu species.
1. Introduction
Pristine biopolymers and especially their chemically-modified derivatives have been recently found to serve as appropriate leading materials in many research and technology areas including vaccines, medical practice, antibacterial and wound healing dressing, naturally degradable sensors, environmental engineering, buildings, energy storage devices, electrochemistry, food packaging, and replacing conventional petrochemical-based polymers as well as appropriate supports for low-toxic and biodegradable catalytic systems.1–11 The use of appropriate catalytic systems to prepare important medicinal compounds through environmental-friendly protocols has received significant interest in both academia and industry in recent decades. Two types of catalytic systems are homogeneous and heterogeneous. Homogeneous catalysts are less frequently used in modern industrial and synthetic applications because they suffer from low thermal stability, harsh and prolonged work-up procedures, and waste generation.12,13 Interestingly, the supramolecular and non-covalent supporting of the homogeneous catalytic species or anchoring of the functional groups on the inorganic or organic supports as well as their hybrid ones to form heterogeneous catalysts can overcome the encountered problems.8,11,14–25 Various organic or inorganic supports including synthetic polymers, natural polymers such as chitosan and cellulose,3,4,10,26–28 bentonite,29,30 SiO2,31,32 perlite, graphene oxide,33,34 MOFs, etc. have been used for this purpose.35–38
The use of natural polymers, as proper supports, has currently attracted much attention to produce various types of heterogeneous catalysts. Chitosan, among other typical biopolymers, has diverse advantages such as biocompatibility, biodegradability, and non-toxicity. It's surface functional groups including NH2 and OH, make it an appropriate support for the preparation of heterogeneous catalysts.9–11,22,25,39–43 Although heterogeneous catalysts usually demonstrate lower catalytic efficiency and selectivity in comparison with homogeneous ones due to their insolubility in the reaction medium, the formation of active catalytic species nanoparticles or the use of nanoscale assemblies of supports may overcome this challenge.44–48 Indeed, nano-ordered heterogeneous catalytic systems can form a bridge between the gap of homogeneous and heterogeneous catalysts through combination of synergistic effects of both systems. The principal goal of nanochemistry and relevant technologies is to follow some useful conditions or chemical reactions for modifying the size, morphology, or surface chemical compositions of the obtained nanomaterials with proper and adjustable chemical activity and selectivity, thermal and mechanical stability, reusability, etc.49 Among them, nanocomposites formed by biopolymeric supports have a spread range of green and environmental friendly beneficial for different applications including catalysts, cosmetic orthodontics, modern construction, electronics, fuel cell, energy storage, etc.50–54 Hence, academia and chemical industry are moving to safer and cleaner strategies of synthesis and manufacturing required chemicals and products by the use of such nanomaterials.55–61
Heterogeneous nanocatalysts comprising modified chitosan can be prepared through a variety of chemical modifications such as metal chelation, carboxylation, acylation, sulfation, cyanoethylation, Schiff base formation, etc., owing to the existence of reactive amino and hydroxyl groups.8,11,62–68 Thus, the modified structures of chitosan are often produced by the chemical manipulation of active sites of OH and NH2 groups, with the object of improving their efficiency for particular applications. To expand the domain of application in the modified chitosan-based nanomaterials, new derivatives comprising diverse functional groups grafted onto the chitosan backbone are expected to be prepared competitively. For example, by using thiosemicarbazide (TS), pyromellitic dianhydride (PMDA) and toluene-2,4-diisocyanate (TDI), as linkers, the chitosan backbone can be modified to afford preferable structures having diverse and appropriate functional groups than the pristine chitosan.69–73
Indeed, PMDA is generally a powerful electrophilic compound due to the presence of anhydride functional groups.74,75 Consequently, it can react with both –NH2 and –OH functional groups of chitosan to form new amide or ester ones, respectively, as well as free carboxylic acid functional groups. Furthermore, the existence of TS, as a good donor ligand and hydrogen bond-forming moiety, increases the catalytic performance of the as-prepared nanomaterial by strong chelation of Lewis acid catalytic species such as transition metal ions on the surface of modified chitosan.76,77 On the other hand, TDI can be used as an appropriate linker due to the presence of reactive isocyanate functional groups to combine with both –NH2 and –OH functional groups of chitosan. Therefore, design and facile preparation of new chitosan-based nanomaterials by grafting of PMDA, TS and TDI would be more desirable.78–82
“Click Chemistry (CC)”, which was firstly introduced in 1999 by K. Barry Sharpless, has emerged in the synthesis of heterocyclic compounds as a fascinating research area. Indeed, this concept resulted in joint awarding the 2022 Nobel Prize in Chemistry to Carolyn R. Bertozzi, Morten Meldal and K. Barry Sharpless for the development of CC and bioorthogonal chemistry. In 2001, Sharpless defined CC reactions as a set of organic transformations with high thermodynamic stimulant energy that pushes them quickly and irreversibly to afford a single reaction product.83–87 As one of the most well-known CC reactions, 1,3-dipolar azide–alkyne and azide–nitrile cycloaddition reactions are widely used for the synthesis of important heterocyclic compounds, namely triazole and tetrazole derivatives, respectively.88–92
Tetrazoles are known as a class of synthetic heterocyclic compounds that comprise a five-membered ring containing four nitrogen and one carbon atoms. Remarkably, since pentazoles are highly explosive even at low temperatures, tetrazoles possess the highest number of nitrogen atoms among the stable heterocycles.93–95 Furthermore, both donor and acceptor electronic characteristics of tetrazole derivatives arise from their planar ring skeleton as well as the nitrogen-rich multi-electron conjugated system.96–99 Tetrazoles have a broad range of applications including in medicinal, material and coordination chemistry, organometallic compounds and catalysis technology.100,101 The tetrazolyl functional group is often considered as a carboxylic acid substitution in drug discovery. Indeed, not only the pKa of tetrazolyl moiety is close to the –COOH functional group, but also it has nearly the planar delocalized system space requirements and provides maximum nitrogen content of any heterocyclic compound.102 Hence, tetrazole moiety can be considered as a bioisosteric alternative analogue for the carboxylic acid, Cl-amidine, or furan ring (Fig. 1a). Consequently, tetrazoles have found applications as antihypertensive,103 antiviral,104 antinociceptive,105 antibacterial,106 and anti-allergic compounds (Fig. 1b) as well as in agriculture and material engineering107 as herbicides, plant growth regulators, explosives and energy storage devices.20,23,108–110
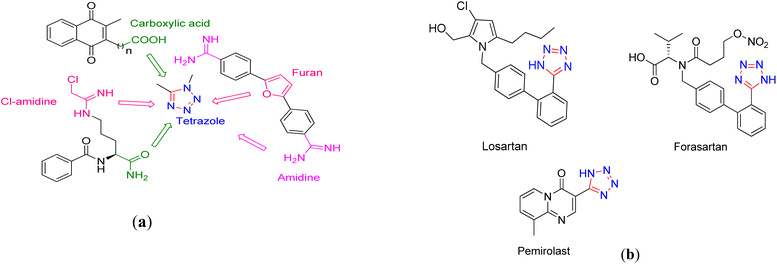 |
| Fig. 1 (a) Tetrazole scaffold as bioisostere of the carboxylic group, Cl-amidine, or heterocyclic furan ring in the medicinal chemistry; (b) active pharmaceutical ingredients having tetrazole moiety: losartan, forasartan, and pemirolast. | |
The first tetrazole compound was prepared more than one hundred years ago, and then many scientists have introduced various synthetic strategies for preparation of these compounds. A 1,3-dipolar cycloaddition reaction is the most common method which takes place between azide ion or hydrazoic acid with various simple nitrile derivatives under high pressure.111 Different catalytic systems have been used in this approach during different decades. Some recent examples consist of the use of zinc(II) salts,112 copper(I) salt,113 MCM-41-SO3H,114 Fe3O4@L-lysine-Pd(0),115 FeCl3–SiO2,116 Zn/Al hydrotalcite,117 Amberlyst-15
118,119 and urea-bridged PMO supporting Cu(II) nanoparticles73 mostly at high temperatures or dialkyl aluminum azides, as the substrate, in the presence of an appropriate catalyst. Indeed, many of these methods suffer from drawbacks for example the use of costly catalytic systems, undesirable yields, the use of toxic and carcinogenic solvents including DMF, long reaction times, and no recycling of the catalyst despite their merits. In continuation of our ongoing studies to explore efficient nanocatalysts for different organic transformations,9–11,21,22,33,43,66–68,120–123 our attention was deployed to the design and application of supramolecular Cu(II) nanoparticles on a modified chitosan backbone. It contains urea and thiourea bridges using thiosemicarbazide (TS), pyromellitic dianhydride (PMDA) and toluene-2,4-diisocyanate (TDI) linkers (CS–TDI–PMDA–TS–Cu(II), 1) for the synthesis of tetrazole derivatives through multicomponent cascade Knoevenagel condensation/click 1,3-dipolar azide–nitrile cycloaddition reactions. Various aromatic aldehydes 4a–q and malononitrile (2) were well survived in the presence of nanocomposite 1 to afford corresponding Knoevenagel intermediate and subsequent [3 + 2] cycloaddition desired products 5a–q by reacting with sodium azide (Scheme 1). Indeed, various tetrazole derivatives 5a–q were efficiently synthesized using a low loading of CS–TDI–PMDA–TS–Cu(II) nanocatalyst (1), as a reusable catalytic system, under solvent-free conditions in short reaction times.
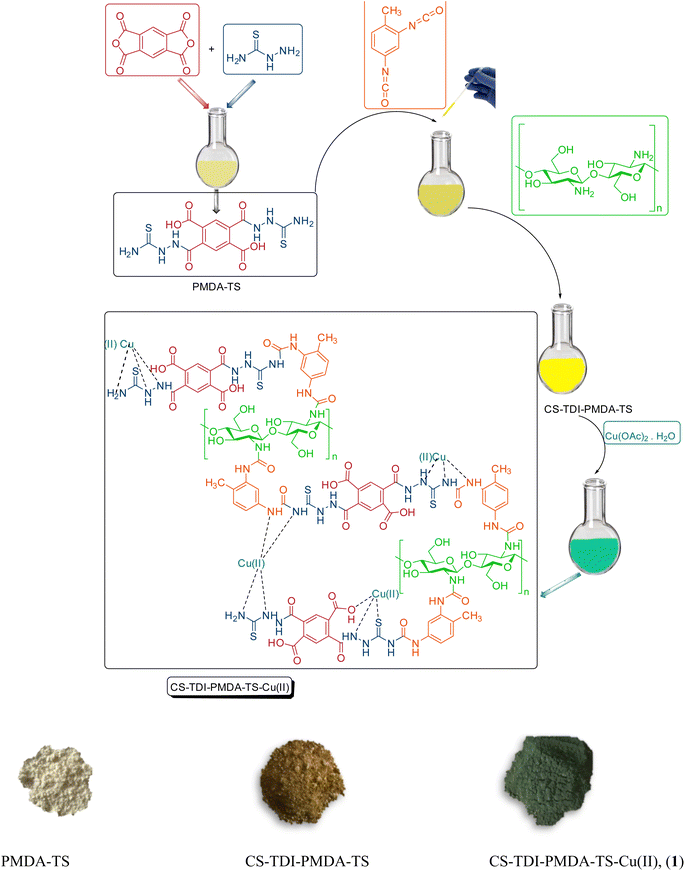 |
| Scheme 1 Schematic preparation of the CS–TDI–PMDA–TS–Cu(II) nanocomposite (1). | |
2. Experimental
2.1. Materials and instrumentation
All chemicals and reagents were purchased with high purity from Merck or Aldrich and used as received, except for liquid aldehydes which were distilled before their use. The progress of reactions and the purity of the obtained products were monitored by thin layer chromatography (TLC) using Merck aluminum plates coated with 0.2 mm silica gel F254. Melting points were measured using an electrothermal 9100 device and are uncorrected. Characterization of the CS–TDI–PMDA–TS–Cu(II) nanocatalyst (1), as well as identification of products, was performed using KBr discs on a Shimadzu FTIR-8400S spectrometer. A Bruker DRX-500 Avance spectrometer was used for the recording of 1H NMR (500 MHz) spectra of products in DMSO-d6 at ambient temperature. Thermal gravimetric analysis data were gathered by a Bahr company STA 504 equipment. The X-ray diffraction pattern was carried out using an STOE apparatus with a CuKα radiation source. Field emission scanning electron microscopy images were recorded by a TESCAN-MIRA III device. All the products are known compounds and were identified by comparison of their physical and spectral data with those of authentic samples.
2.2. Preparation of the chitosan modified with urea and thiourea bridges using TS, PMDA and TDI linkers (CS–TDI–PMDA–TS)
In a single-neck round-bottom 20 mL flask equipped with a condenser, a mixture of TS (2.00 mmol) and PMDA (1.00 mmol) in THF (10 mL) was heated under reflux conditions for 24 h. Then, the mixture was cooled to ambient temperature and the obtained white powder was washed with THF and dried at 70 °C for 6 h. The product was dispersed in 20 mL of dry toluene at room temperature. After 30 min of stirring, TDI (1.0 mmol) was added to the prepared mixture dropwise under an argon atmosphere. The mixture was heated in a two-neck round-bottom flask equipped with a condenser under reflux conditions for 12 h. Afterward, CS (0.2 g) was added to the mixture for 36 h under the same conditions. The obtained light-yellow powder was washed with dry toluene and dried at 70 °C for 24 h.
2.3. Preparation of CS–TDI–PMDA–TS–Cu(II) nanocatalyst (1)
At this stage, CS–TDI–PMDA–TS in the presence of copper(II) acetate monohydrate was used to embed copper into the CS–TDI–PMDA–TS backbone. Copper(II) acetate monohydrate (0.2 g) was added to a mixture of the CS–TDI–PMDA–TS (0.4 g) dispersed in EtOH 96% (15 mL) and stirred for 12 h at room temperature. Finally, the light green powder of CS–TDI–PMDA–TS–Cu(II) nanomaterial (1) was filtered off and washed with EtOH 96% (3 × 10 mL) and then dried at 70 °C for 24 h.
2.4. General procedure for the synthesis of 5-substituted-1H-tetrazoles derivatives 5a–q catalyzed by CS–TDI–PMDA–TS–Cu(II) nanocatalyst (1)
In a screwed test tube, a mixture of aryl aldehyde (4a–q, 1.00 mmol), malononitrile (2, 1.10 mmol), and sodium azide (3, 1.50 mmol) was heated in the presence of CS–TDI–PMDA–TS–Cu(II) nanocatalyst (1, 20 mg) under solvent-free conditions at 110 °C in an oil bath and stirred for the time indicated in Table 1. The reaction progress was monitored by TLC (n-hexane/EtOAc, 1
:
1). After completion of the reaction, the nanocatalyst 1 was easily separated through filtration of the obtained aqueous mixture by adding of distilled water (2.5 mL). To separate the products, aqueous HCl (2.0 N, 2.5 mL) and EtOAc (3 × 3.0 mL) were added to the filtrate with vigorous stirring. Then, the two-phase mixture was separated using a separatory funnel each time. The desired products 5a–q were crystallized by adding n-hexane and scraping the bottom of the container. After filtering and drying the precipitate at 70 °C, tetrazole compounds 5a–q were obtained. By employing 1H NMR, and FT-IR spectroscopy and melting point, the as-synthesized compounds 5 were characterized.
Table 1 Optimization of the conditions for the synthesis of 1H-tetrazoles in the model reaction of malononitrile (2), NaN3 (3) and 4-chlorobenzaldehyde (4a) under different conditionsa
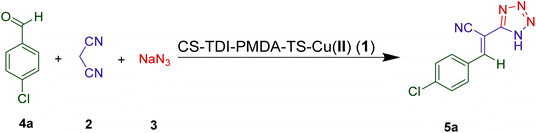
|
Entry |
Catalyst |
Catalyst loading (mg) |
Solvent |
Temperature (°C) |
Time (min) |
Yield of 5a (%)b |
Reaction conditions: malononitrile (2, 1.10 mmol), sodium azide (3, 1.50 mmol), and 4-chlorobenzaldehyde (4a, 1.00 mmol) in the presence of CS–TDI–PMDA–TS-Cu(II) nanocatalyst (1, 20 mg) and solvent (2.0 mL) unless otherwise noted. Isolated yield. |
1 |
— |
— |
Solvent-free |
110 |
24 h |
60 |
2 |
— |
— |
DMF |
Reflux |
20 h |
50 |
3 |
CS–TDI–PMDA–TS–Cu(II), (1) |
10 |
Solvent-free |
110 |
30 |
65 |
4 |
CS–TDI–PMDA–TS–Cu(II), (1) |
15 |
Solvent-free |
110 |
30 |
80 |
5 |
CS–TDI–PMDA–TS–Cu(II), (1) |
20 |
Solvent-free |
110 |
30 |
92 |
6 |
CS–TDI–PMDA–TS–Cu(II), (1) |
40 |
Solvent-free |
110 |
45 |
94 |
7 |
CS–TDI–PMDA–TS–Cu(II), (1) |
20 |
EtOH |
Reflux |
60 |
80 |
8 |
CS–TDI–PMDA–TS–Cu(II), (1) |
20 |
CH2Cl2 |
Reflux |
60 |
50 |
9 |
CS–TDI–PMDA–TS–Cu(II), (1) |
20 |
H2O |
Reflux |
60 |
87 |
10 |
CS–TDI–PMDA–TS–Cu(II), (1) |
20 |
DMF |
Reflux |
60 |
90 |
11 |
CS–TDI–PMDA–TS–Cu(II), (1) |
20 |
EtOAc |
Reflux |
60 |
55 |
12 |
CS–TDI–PMDA–TS–Cu(II), (1) |
20 |
Solvent-free |
120 |
50 |
93 |
13 |
CS–TDI–PMDA–TS–Cu(II), (1) |
20 |
Solvent-free |
100 |
70 |
90 |
14 |
CS–TDI–PMDA–TS–Cu(II), (1) |
20 |
Solvent-free |
80 |
110 |
60 |
2.5. Spectral data of the selected products
2.5.1 (E)-3-(2-Chlorophenyl)-2-(1H-tetrazole-5-yl) acrylonitrile (5b). Cream solid powder; Mp: 174–176 °C; FT-IR (KBr) ν: 3241, 3025, 2207, 1573, 1475, 973, 824, 672 cm−1; 1H NMR (500 MHz, DMSO-d6): δ (ppm) 7.55–7.57 (m, 2H), 7.65–7.67 (d, J = 8.4 Hz, 1H), 8.10–8.11 (d, J = 6.3 Hz, 1H), 8.42 (s, 1H, CH), 13.19 (s, 1H, NH).
2.5.2 (E)-3-(4-Methoxyphenyl)-2-(1H-tetrazole-5-yl) acrylonitrile (5l). Pale yellow powder; Mp: 155–158 °C; FT-IR (KBr) ν: 3148, 2256, 1573, 1416, 1118, 930, 810, 681, 649 cm−1; 1H NMR (500 MHz, DMSO-d6): δ (ppm) 7.13–7.14 (d, J = 8.3 Hz, 2H), 8.00–8.02 (d, J = 8.3 Hz, 2H), 8.27 (s, 1H, CH), 13.40 (s, 1H, NH).
2.5.3 (E)-3-(4-Methylphenyl)-2-(1H-tetrazole-5-yl) acrylonitrile (5n). Cream solid powder; Mp: 189–191 °C; FT-IR (KBr) ν: 3029, 2217, 1575, 1556, 1376, 1217, 1174, 813, 609 cm−1; 1H NMR (500 MHz, DMSO-d6): δ (ppm) 7.41–7.43 (d, J = 8.0 Hz, 2H), 7.92–7.94 (d, J = 8.0 Hz, 2H), 8.34 (s, 1H, CH), 13.40 (s, 1H, NH).
3. Results and discussion
3.1. Characterization of the heterogeneous CS–TDI–PMDA–TS–Cu(II) nanocatalyst (1)
After the preparation of the CS–TDI–PMDA–TS–Cu(II) nanomaterial (1), its structural, morphological, and textural properties were characterized by using different suitable methods and techniques such as Fourier-transform infrared (FT-IR) spectroscopy, field emission scanning electron microscopy (FESEM), thermogravimetry/differential thermogravimetry analysis (TGA/DTA), energy-dispersive X-ray spectroscopy (EDS), elemental mapping, and X-ray diffraction (XRD).
3.1.1. Fourier transform infrared (FTIR) analysis. In Fig. 2, the FT-IR spectra of commercial chitosan (CS, a), PMDA–TS (b), CS–TDI–PMDA–TS (c), and CS–TDI–PMDA–TS–Cu(II) nanomaterial (1, d) have been compared. In Fig. 2a, the stretching vibrations of N–H and O–H bonds of CS appears, as a broad absorption band, at 3100–3426 cm−1 in the FT-IR spectra. Also, the C–H stretching vibrations can be seen as the bands at 2926 cm−1. In Fig. 2b the C
S of thiourea group and carbonyl group of carboxylic acid bands are appeared at 1654 cm−1 and 1702 cm−1, respectively. In Fig. 2c, the band at 1708 cm−1 corresponds to the carbonyl group of carboxylic acid, while the bands at 1622 and 1504 cm−1 are assigned to the urea and thiourea groups, respectively. We believe that the small shift in locations of these characteristic bands can be attributed to their interactions with the –OH, –NH2, and N–H groups. The peaks at 1200–1400 cm−1 are assigned to the bending of –NH groups. The C–O stretching band is located at about 1100 cm−1. Consequently, the CS containing TS and PMDA by TDI linker were successfully prepared. By interacting the copper(II) species with the surface of catalyst, the vibration signal is observed at 814 cm−1, as shown in Fig. 2d, which confirms the efficient chelation of copper(II) on the modified biopolymeric support's surface.
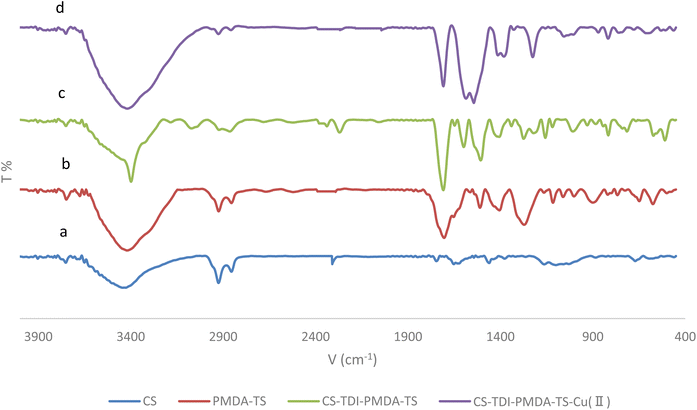 |
| Fig. 2 FT-IR spectra of the commercial CS (a), PMDA–TS (b), CS–TDI–PMDA–TS (c), and the CS–TDI–PMDA–TS–Cu(II) nanocomposite (1, d). | |
3.1.2. Scanning electron microscopy (FESEM) analysis. The morphology and particles size distribution of CS–TDI–PMDA–TS–Cu(II) nanomaterial (1) were determined by using FESEM technique (Fig. 3). Indeed, the layered modified chitosan backbones are observed obviously while grafted linkers as well as spherical chelated Cu(II) nanoparticles have been distributed on the surface of them. Furthermore, the average particle size is between 37 and 49 nm, denoting uniform distribution of particles and appropriate morphology for the CS–TDI–PMDA–TS–Cu(II) nanocatalyst (1).
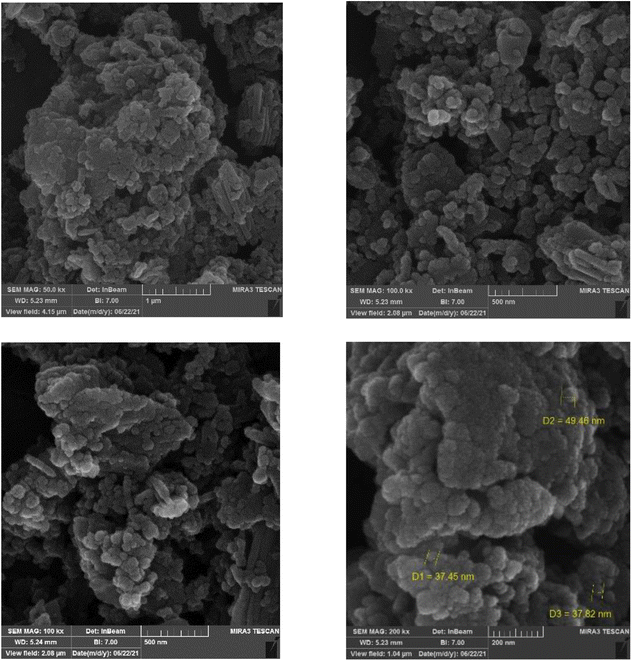 |
| Fig. 3 FESEM images of the CS–TDI–PMDA–TS–Cu(II) nanocomposite (1). | |
3.1.3. Thermogravimetric analysis (TGA). The thermogravimetry/differential thermogravimetry analysis (TGA/DTA) were measured for the as-prepared nanocatalyst in the range of 50–500 °C. Fig. 4 demonstrates that the physically adsorbed organic solvents' weight loss of CS–TDI–PMDA–TS–Cu(II) nanocatalyst (1) is observed at the range of 50–200 °C. Indeed, physically adsorbed THF, toluene, alcoholic or water molecules which were remained from the preparation steps are removed at first weight loss stage. The next two weight losses of the CS–TDI–PMDA–TS–Cu(II) nanocatalyst (1) at 200–500 °C are attributed to the elimination of urea and thiourea bridges grafted onto the surface of chitosan by using TDI and PMDA linkers. These data show the good thermal stability of the CS–TDI–PMDA–TS–Cu(II) nanocomposite (1), which is highly significant in the design and application of reusable heterogeneous catalytic systems.
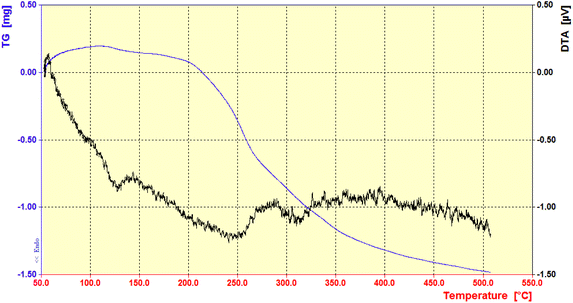 |
| Fig. 4 TGA/DTA analysis of the CS–TDI–PMDA–TS–Cu(II) nanomaterial (1). | |
3.1.4. Energy dispersive X-ray spectroscopy (EDX) analysis. The composition of nanomaterial 1 was determined by energy dispersive X-ray (EDX) spectroscopy. Fig. 5 shows the EDX spectrum of the nanocomposite 1. The signals of copper, nitrogen, sulfur, carbon, and oxygen were distinguished in the as-prepared material. In addition, this analysis proves that copper(II) species are well embedded in the structure of nanocatalyst successfully.
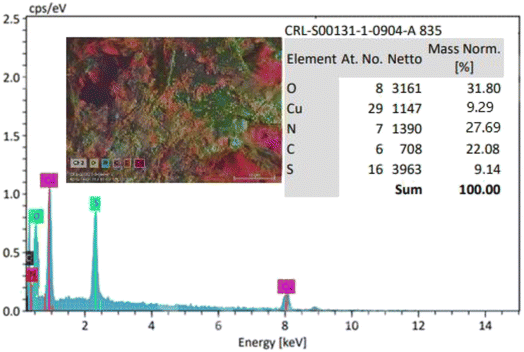 |
| Fig. 5 EDX spectrum of the CS–TDI–PMDA–TS–Cu(II) nanocatalyst (1). | |
3.1.5. Elemental mapping analysis. Another useful technique to demonstrate the distribution of elements in the structure of nanomaterials is elemental mapping. As shown in Fig. 6, the elements of copper, nitrogen, sulfur, carbon, and oxygen are well uniformly distributed throughout the chosen area of the EDX image. This property in the heterogeneous catalytic systems is very important to demonstrate higher catalytic activity at lower concentration of active species such as Cu cations in the structure of CS–TDI–PMDA–TS–Cu(II) nanocatalyst (1).
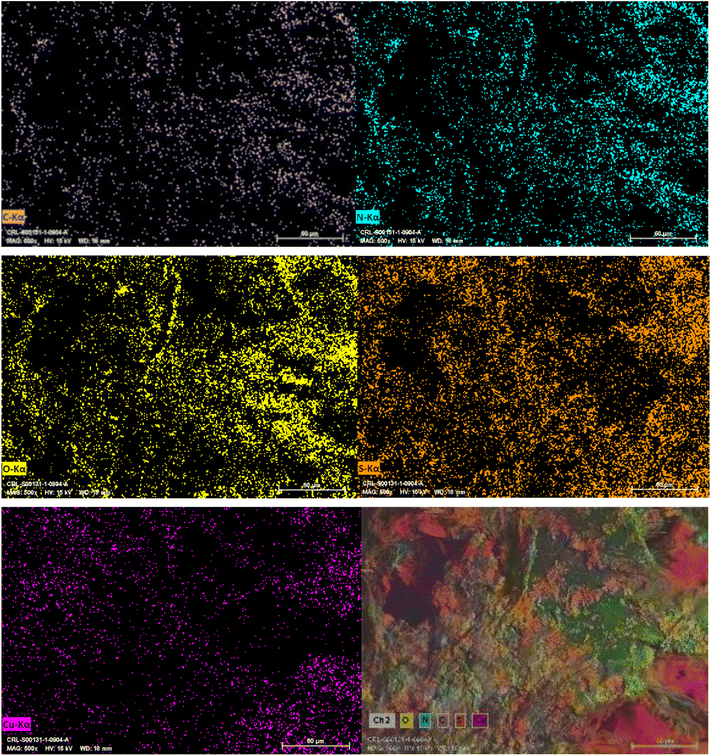 |
| Fig. 6 Elemental mapping of the CS–TDI–PMDA–TS–Cu(II) nanocatalyst (1). | |
3.1.6. X-Ray diffraction spectroscopy (XRD) analysis. The XRD was also employed to study the crystalline structures of CS–TDI–PMDA–TS–Cu(II) nanocatalyst (1). The wide-angle XRD patterns shown in Fig. 7 demonstrate the crystallinity and arrangement of CS–TDI–PMDA–TS–Cu(II) nanocomposite (1) and full compliance with the CS (JCPDS card no. 00-039-1894), PMDA (JCPDS card no. 00-024-1864), TS (JCPDS card no. 00-008-0726), copper(II) acetate monohydrate (JCPDS card no. 00-004-0836). Also, chemical modifications led to decrease of peak intensities of the chitosan.
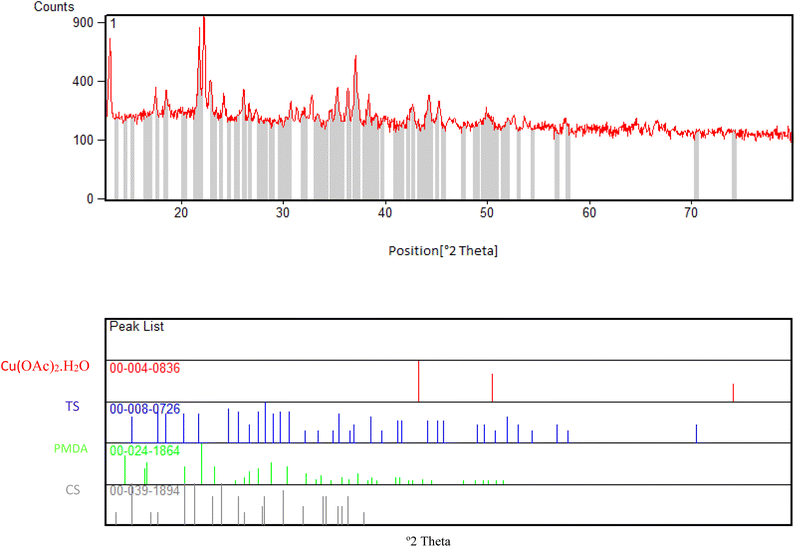 |
| Fig. 7 XRD pattern for the CS–TDI–PMDA–TS–Cu(II) nanocatalyst (1). | |
3.2. Optimization of conditions for the synthesis of tetrazole derivatives in the presence of CS–TDI–PMDA–TS–Cu(II) nanocatalyst (1)
After characterization of the CS–TDI–PMDA–TS–Cu(II) nanocatalyst (1), its performance for the preparation of 1H-tetrazole derivatives was assessed. Thus, the effect of parameters including various catalyst loadings, temperature, and solvent on the yield and reaction time were studied in this step systematically to recognize optimal conditions for the synthesis of 1H-tetrazole derivatives. Therefore, the reaction between 4-chlorobenzaldehyde (4a, 1.00 mmol), malononitrile (2, 1.10 mmol) and sodium azide (3, 1.50 mmol) was chosen as the model reaction in this study. Table 1 summarizes the obtained results. Initially, in the absence of any catalyst, the model reaction was performed in DMF under reflux conditions or solvent-free conditions. The dependency of the obtained yield of the model reaction to the catalyst and temperature was obvious because of moderate yields of the desired product (E)-3-(4-chlorophenyl)-2-(1H-tetrazole-5-yl) acrylonitrile (5a) after prolonged reaction times (entries 1–2, Table 1). Remarkably, the obtained yield of desired product of 5a was significantly improved in the presence of CS–TDI–PMDA–TS–Cu(II) nanocatalyst (1) after very short reaction times (entries 3–6, Table 1). These findings demonstrate appropriate performance of the nanocatalyst 1 in promoting of the model reaction to afford the desired product 5a, which originates from very good dispersion of active catalytic sites and proper surface area of the CS–TDI–PMDA–TS–Cu(II) nanomaterial (1).
In the next step, the effects of different polar and nonpolar solvents as well as solvent-free conditions were examined on the reaction times and yields (Table 1, entries 5 and 7–14). Consequently, when the model reaction was carried out under solvent-free conditions, the best results were achieved (Table 1, entry 5). Furthermore, with decreasing applied temperatures from 120 to 80 °C, the yield of desired product diminishes (Table 1, entries 5 and 12–14). It is noteworthy that when the model reaction was performed at 110 °C, the best results in terms of yield and reaction time were gained. Indeed, higher temperature cannot improve the amount of yield or reaction time (entry 12, Table 1). Furthermore, separation of the nanocatalyst 1 is much easier under solvent-free conditions compared to experiments in different solvents during work-up procedure and remaining the heterogeneous catalyst 1.
Afterward, the optimized conditions were expanded to other aromatic aldehydes 4b–q to investigate the scope of reaction for synthesizing of 1H-tetrazole derivatives in the presence of CS–TDI–PMDA–TS–Cu(II) nanocatalyst (1). A diversity of aromatic carbocyclic or heterocyclic aldehydes 4a–q well survived to involve smoothly in the optimized conditions. As can be observed in Table 2, high to excellent yields were obtained for the desired products 5a–q within very short reaction times. Accordingly, aromatic aldehydes with a carbocyclic ring bearing electron withdrawing groups 4a–h or electron-deficient heterocycle 4i were examined (entries 1–9, Table 2). On the other hand, aromatic aldehydes with a carbocyclic ring bearing electron donating groups 4j–q as well as electron-rich heterocycle 4o were studied to involve in the optimized conditions to afford corresponding 1H-tetrazole derivatives 5j–q (entries 10–17, Table 2). Indeed, the required reaction time for completion is usually lower for the aldehydes containing an electron-withdrawing group compared to those ones having a donor group. This implies that formation of the Knoevenagel intermediate is the rate-determining step for all studied aldehydes.
Table 2 Synthesis of 5-substituted-1H-tetrazole derivatives 5a–q catalyzed by the CS–TDI–PMDA–TS–Cu(II) nanomaterial (1)a
3.3. Proposed mechanism for the preparation of 5-substituted-1H-tetrazole derivatives catalyzed by CS–TDI–PMDA–TS–Cu(II) nanocatalyst (1)
A plausible mechanism for the one-pot synthesis of (E)-2-(1H-tetrazole-5-yl)-3-aryl/heteroarylacrylenenitrile derivatives 5a–q using CS–TDI–PMDA–TS–Cu(II) nanocatalyst (1) is shown in Scheme 2. Initially, the aromatic aldehydes 4 are activated by CS–TDI–PMDA–TS–Cu(II) nanocatalyst (1) step by step to condensate with the nucleophilic tautomer of malononitrile (2′) having C–H acids and affording Knoevenagel intermediate (II).129 In order to form the 5-membered tetrazole ring, the activated aryl/heteroarylidine malononitrile Knoevenagel intermediate (II) by the nanocatalyst 1 is subsequently involved in a cascade reaction with sodium azide (3) through the concerted [3 + 2] cycloaddition to afford corresponding tetrazole sodium salt (III). Finally, the desired tetrazole derivatives are formed by the addition of HCl (aq). Furthermore, the CS–TDI–PMDA–TS–Cu(II) nanocatalyst (1) is liberated and can start next catalytic cycle.
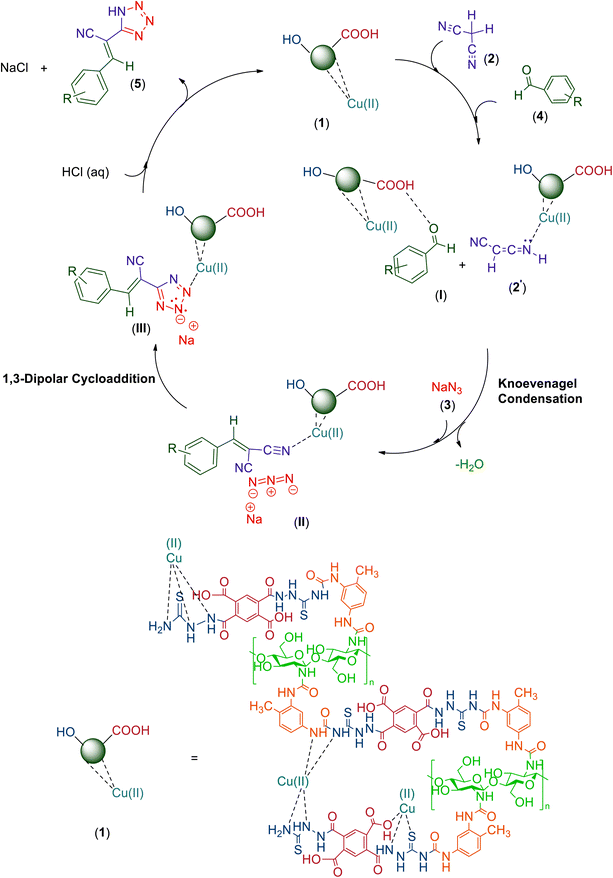 |
| Scheme 2 The proposed mechanism for the synthesis of tetrazole derivatives 5 catalyzed by the heterogeneous supramolecular CS–TDI–PMDA–TS–Cu(II)nanocatalyst (1). | |
3.4. Comparison of the catalytic activity of CS–TDI–PMDA–TS–Cu(II) nanocatalyst (1) in the synthesis of tetrazole derivatives with other catalytic systems
A comparison of the previously reported methods for the synthesis of tetrazole 5a with the present protocol is shown in Table 3. It is apparent that high to excellent yields, short reaction time, and avoiding the use of toxic or carcinogenic solvents such as DMF is the advantages of the present protocol compared to the most of previously reported methods.
Table 3 Comparison of the catalytic efficiency of the CS–TDI–PMDA–TS–Cu(II) nanocatalyst (1) with other catalytic systems
Entry |
Catalyst |
Catalyst loading |
Reaction conditions |
Time (h) |
Yield (%) |
Reference |
1 |
CS–TDI–PMDA–TS–Cu(II), (1) |
20.0 mg |
Solvent-free/110 °C |
30 min |
92 |
Present work |
2 |
Nano-Fe3O4 |
20 mol% |
Solvent-free/80 °C |
4.5 |
90 |
124 |
3 |
Fe3O4@fibroin–SO3H |
10 mol% |
Solvent-free/100 °C |
2.0 |
86 |
130 |
4 |
Fe3O4–CNT–SO3H nanocomposite |
20.0 mg |
Solvent-free/80 °C |
2.5 |
90 |
131 |
5 |
NiO nanoparticles |
4.5 mg |
DMF/70 °C |
6.0 |
90 |
132 |
6 |
NH–Cu(II)@MNP |
20.0 mg |
EtOH/80 °C |
5.0 |
92 |
133 |
7 |
Zn (mettalic) |
130.7 mg |
H2O/50 °C |
3.0 |
68 |
134 |
8 |
(CuOTf)2·C6H6 |
36.2 mg |
Toluene/r.t. |
7.0 |
81 |
135 |
9 |
Fe(OAc)2 |
17.4 mg |
DMF/H2O (9 : 1) 80 °C |
24.0 |
89 |
136 |
10 |
OPNSA |
45.4 mg |
Solvent-free/100 °C |
10.0 |
80 |
137 |
3.5. Examining the recyclability of CS–TDI–PMDA–TS–Cu(II) nanocatalyst (1) for the synthesis of 5-substituted-1H-tetrazole derivatives 5
Currently, reusability is a significant parameter in the field of nano-ordered heterogeneous catalytic systems to address green and sustainable chemistry principles and reducing the impact of chemical processes on the environment. In this context, the recyclability of as-prepared CS–TDI–PMDA–TS–Cu(II) nanocatalyst 1 was assessed under optimal conditions for the model reaction. Hence, after the completion of the model reaction, the nanocatalyst 1 was easily separated by filtration after addition of aqueous HCl. Then, the recycled CS–TDI–PMDA–TS–Cu(II) nanocatalyst (1) was dispersed in EtOH and H2O for 15 min, respectively. The recycled catalyst was kept in an oven at 70 °C for one hour and reused for the next run under optimized conditions. Fig. 8 provides the results of the catalyst recycling for five consecutive runs. As data in Fig. 8 demonstrate, a little decrease in the reaction yield was observed in each run under optimized conditions. These data indicate the capability of the catalyst to be reused with structural stability and high efficiency.
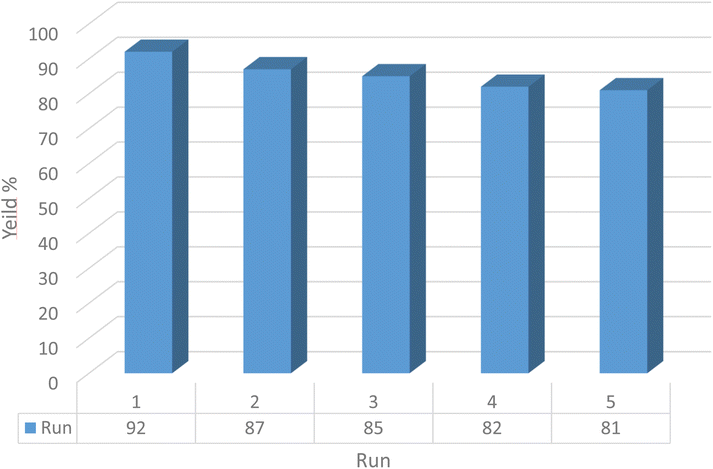 |
| Fig. 8 Reusability data for the CS–TDI–PMDA–TS–Cu(II) nanocatalyst (1) in the synthesis of 5a. | |
4. Conclusions
In conclusion, a new supramolecular CS–TDI–PMDA–TS–Cu(II) nanocomposite based on the naturally occurring chitosan biopolymer with urea and thiourea bridges was designed, easily prepared, and fully characterized by appropriate spectroscopic and analytical methods or techniques used for nanomaterials. The catalytic activity of CS–TDI–PMDA–TS–Cu(II) nanomaterial was demonstrated in the one-pot synthesis of tetrazole derivatives through cascade Knoevenagel condensation and concerted 1,3-cycloaddition reactions as a representative of the Click Chemistry concept. The desired 5-substituted-1H-tetrazole derivatives were prepared under solvent-free conditions in good purity and high to excellent yields under mild conditions. Low catalyst loading, solvent-free conditions, very short reaction times, and reusability of the catalyst for at least five consecutive runs without significant loss of its activity can be considered as desirable features of this new protocol.
Conflicts of interest
There are no conflicts to declare.
Acknowledgements
We are grateful for the financial support from The Research Council of Iran University of Science and Technology (IUST), Tehran, Iran (Grant No. 160/22061) for their support. We would also like to acknowledge the support of The Iran Nanotechnology Initiative Council (INIC), Iran.
References
- T. Chen, Y. Peng, M. Qiu, C. Yi and Z. Xu, Nanoscale, 2023, 15, 3594–3609 RSC.
- Y. Pei, L. Wang, K. Tang and D. L. Kaplan, Adv. Funct. Mater., 2021, 31, 2008552 CrossRef CAS.
- H. Mittal, S. S. Ray, B. S. Kaith, J. K. Bhatia, J. Sharma and S. M. Alhassan, Eur. Polym. J., 2018, 109, 402–434 CrossRef CAS.
- G. Crini, Environ. Chem. Lett., 2019, 17, 1623–1643 CrossRef CAS.
- J. Wróblewska-Krepsztul, T. Rydzkowski, I. Michalska-Pożoga and V. K. Thakur, Nanomaterials, 2019, 9, 404 CrossRef PubMed.
- L. Zhang, Y. Yang, J. Tan and Q. Yuan, Mater. Chem. Front., 2020, 4, 1315–1327 RSC.
- D. Das and S. Pal, RSC Adv., 2015, 5, 25014–25050 RSC.
- M. Dohendou, K. Pakzad, Z. Nezafat, M. Nasrollahzadeh and M. G. Dekamin, Int. J. Biol. Macromol., 2021, 192, 771–819 CrossRef CAS PubMed.
- E. Valiey, M. G. Dekamin and S. Bondarian, RSC Adv., 2023, 13, 320–334 RSC.
- M. G. Dekamin, M. Azimoshan and L. Ramezani, Green Chem., 2013, 15, 811–820 RSC.
- M. Dohendou, M. G. Dekamin and D. Namaki, Nanoscale Adv., 2023, 5, 3463–3484 RSC.
- V. S. Shende, V. B. Saptal and B. M. Bhanage, Chem. Rec., 2019, 19, 2022–2043 CrossRef CAS PubMed.
- D. Cole-Hamilton and R. Tooze, in Catalyst Separation, Recovery and Recycling, Springer, 2006, pp. 1–8 Search PubMed.
- C. J. Brown, F. D. Toste, R. G. Bergman and K. N. Raymond, Chem. Rev., 2015, 115, 3012–3035 CrossRef CAS PubMed.
- S. Reimann, J. Stötzel, R. Frahm, W. Kleist, J.-D. Grunwaldt and A. Baiker, J. Am. Chem. Soc., 2011, 133, 3921–3930 CrossRef CAS PubMed.
- J. H. Clark, in Handbook of Green Chemistry and Technology, 2002, pp. 1–9, DOI:10.1002/9780470988305.ch1.
- K. Bourikas, H. K. Matralis, C. Kordulis and A. Lycourghiotis, J. Phys. Chem., 1996, 100, 11711–11719 CrossRef CAS.
- A. Alinasab Amiri, S. Javanshir, Z. Dolatkhah and M. G. Dekamin, New J. Chem., 2015, 39, 9665–9671 RSC.
- M. G. Dekamin and Z. Mokhtari, Tetrahedron, 2012, 68, 922–930 CrossRef CAS.
- S. Safapoor, M. G. Dekamin, A. Akbari and M. R. Naimi-Jamal, Sci. Rep., 2022, 12, 10723 CrossRef CAS PubMed.
- S. Karami, M. G. Dekamin, E. Valiey and P. Shakib, New J. Chem., 2020, 44, 13952–13961 RSC.
- M. G. Dekamin, E. Kazemi, Z. Karimi, M. Mohammadalipoor and M. R. Naimi-Jamal, Int. J. Biol. Macromol., 2016, 93, 767–774 CrossRef CAS PubMed.
- E. Valiey and M. G. Dekamin, Sci. Rep., 2022, 12, 18139 CrossRef CAS PubMed.
- M. Dohendou, M. G. Dekamin and D. Namaki, Nanoscale Adv., 2023, 5, 2621–2638 RSC.
- B. Fattahi and M. G. Dekamin, Colloid Interface Sci. Commun., 2023, 54, 100711 CrossRef CAS.
- T. Fazal, B. N. Murtaza, M. Shah, S. Iqbal, M. Rehman, F. Jaber, A. A. Dera, N. S. Awwad and H. A. Ibrahium, RSC Adv., 2023, 13, 23087–23121 RSC.
- Y. Wang, Y. Xiong, J. Wang and X. Zhang, Catal. Commun., 2017, 90, 14–18 CrossRef CAS.
- K. Parvanak Boroujeni and P. Tahani, Inorg. Nano-Met. Chem., 2017, 47, 1150–1156 CrossRef CAS.
- K. Bahrami and M. Khodamorady, Catal. Lett., 2019, 149, 688–698 CrossRef CAS.
- M. Khodamorady and K. Bahrami, Catal. Lett., 2020, 150, 1571–1590 CrossRef CAS.
- Z. Alirezvani, M. G. Dekamin and E. Valiey, ACS Omega, 2019, 4, 20618–20633 CrossRef CAS PubMed.
- A. Yaghoubi and M. G. Dekamin, ChemistrySelect, 2017, 2, 9236–9243 CrossRef CAS.
- M. G. Dekamin, F. Mehdipoor and A. Yaghoubi, New J. Chem., 2017, 41, 6893–6901 RSC.
- S. Hasanzadeh Banakar, M. G. Dekamin and A. Yaghoubi, New J. Chem., 2018, 42, 14246–14262 RSC.
- N. Norouzi, M. K. Das, A. J. Richard, A. A. Ibrahim, H. M. El-Kaderi and M. S. El-Shall, Nanoscale, 2020, 12, 19191–19202 RSC.
- J. Liang, Z. Liang, R. Zou and Y. Zhao, Adv. Mater., 2017, 29, 1701139 CrossRef PubMed.
- R. Schlögl, Angew. Chem., Int. Ed., 2015, 54, 3465–3520 CrossRef PubMed.
- C. M. Friend and B. Xu, Acc. Chem. Res., 2017, 50, 517–521 CrossRef CAS PubMed.
- T. Jin, T. Liu, E. Lam and A. Moores, Nanoscale Horiz., 2021, 6, 505–542 RSC.
- S. S. Khutsishvili, T. y. V. Ganenko and B. G. Sukhov, J. Carbohydr. Chem., 2021, 1–15 Search PubMed.
- C. P. Jiménez-Gómez and J. A. Cecilia, Molecules, 2020, 25, 3981 CrossRef PubMed.
- P. S. Bakshi, D. Selvakumar, K. Kadirvelu and N. Kumar, Int. J. Biol. Macromol., 2020, 150, 1072–1083 CrossRef CAS PubMed.
- Z. Alirezvani, M. G. Dekamin, F. Davoodi and E. Valiey, ChemistrySelect, 2018, 3, 10450–10463 CrossRef CAS.
- M. Miceli, P. Frontera, A. Macario and A. Malara, Catalysts, 2021, 11, 591 CrossRef CAS.
- H.-J. Qiu, H.-T. Xu, L. Liu and Y. Wang, Nanoscale, 2015, 7, 386–400 RSC.
- J. Govan and Y. K. Gun'ko, Nanomaterials, 2014, 4, 222–241 CrossRef PubMed.
- L. A. Kolahalam, I. K. Viswanath, B. S. Diwakar, B. Govindh, V. Reddy and Y. Murthy, Mater. Today: Proc., 2019, 18, 2182–2190 CrossRef.
- T. A. Saleh, Environ. Technol. Innovation, 2020, 20, 101067 CrossRef CAS.
- N. Narayan, A. Meiyazhagan and R. Vajtai, Materials, 2019, 12, 3602 CrossRef CAS PubMed.
- J. R. Peralta-Videa, L. Zhao, M. L. Lopez-Moreno, G. de la Rosa, J. Hong and J. L. Gardea-Torresdey, J. Hazard. Mater., 2011, 186, 1–15 CrossRef CAS PubMed.
- D. Astruc, Chem. Rev., 2020, 120, 461–463 CrossRef CAS PubMed.
- N. Baig, I. Kammakakam and W. Falath, Mater. Adv., 2021, 2, 1821–1871 RSC.
- B. Goswami, Int. J. Nanomater. and Nanostruct., 2021, 7, 13–18 Search PubMed.
- K. Hemalatha, G. Madhumitha, A. Kajbafvala, N. Anupama, R. Sompalle and S. Mohana Roopan, J. Nanomater., 2013, 2013, 341015 CrossRef.
- S. R. Attar and S. B. Kamble, Nanoscale, 2022, 14, 16761–16786 RSC.
- F. Ganjali, A. Kashtiaray, S. Zarei-Shokat, R. Taheri-Ledari and A. Maleki, Nanoscale Adv., 2022, 4, 1263–1307 RSC.
- M. B. Gawande, A. K. Rathi, J. Tucek, K. Safarova, N. Bundaleski, O. M. Teodoro, L. Kvitek, R. S. Varma and R. Zboril, Green Chem., 2014, 16, 4137–4143 RSC.
- E. Colusso and A. Martucci, J. Mater. Chem. C, 2021, 9, 5578–5593 RSC.
- S. B. Singh and P. K. Tandon, J. Energy Chem. Eng., 2014, 2, 106–115 Search PubMed.
- V. Polshettiwar and R. S. Varma, Green Chem., 2010, 12, 743–754 RSC.
- M. Zvaigzne, P. Samokhvalov, Y. K. Gun'ko and I. Nabiev, Nanoscale, 2021, 13, 20354–20373 RSC.
- T. Freire, L. M. Dutra, D. Queiroz, N. Ricardo, K. Barreto, J. Denardin, F. R. Wurm, C. Sousa, A. Correia and P. de Lima-Neto, Carbohydr. Polym., 2016, 151, 760–769 CrossRef CAS PubMed.
- S. G. Kou, L. M. Peters and M. R. Mucalo, Int. J. Biol. Macromol., 2021, 169, 85–94 CrossRef CAS PubMed.
- W. Wang, C. Xue and X. Mao, Int. J. Biol. Macromol., 2020, 164, 4532–4546 CrossRef CAS PubMed.
- H. El Knidri, R. Belaabed, A. Addaou, A. Laajeb and A. Lahsini, Int. J. Biol. Macromol., 2018, 120, 1181–1189 CrossRef CAS PubMed.
- N. Rostami, M. G. Dekamin, E. Valiey and H. Fanimoghadam, Sci. Rep., 2022, 12, 8642 CrossRef CAS PubMed.
- N. Rostami, M. G. Dekamin and E. Valiey, Carbohydr. Polym. Technol. Appl., 2023, 5, 100279 CAS.
- Z. Alirezvani, M. G. Dekamin and E. Valiey, Sci. Rep., 2019, 9, 17758 CrossRef PubMed.
- M. Ahmad, S. Ahmed, B. L. Swami and S. Ikram, Carbohydr. Polym., 2015, 132, 164–172 CrossRef CAS PubMed.
- M. Li, Z. Zhang, R. Li, J. J. Wang and A. Ali, Int. J. Biol. Macromol., 2016, 86, 876–884 CrossRef CAS PubMed.
- Y.-c. Lin, H.-p. Wang, F. Gohar, M. H. Ullah, X. Zhang, D.-f. Xie, H. Fang, J. Huang and J.-x. Yang, Int. J. Biol. Macromol., 2017, 95, 476–483 CrossRef CAS PubMed.
- S. P. G. Zaferani, M. R. S. Emami, M. K. Amiri and E. Binaeian, Int. J. Biol. Macromol., 2019, 139, 307–319 CrossRef CAS PubMed.
- E. Valiey and M. G. Dekamin, Nanoscale Adv., 2022, 4, 294–308 RSC.
- J. Deng, Y. Liu, S. Liu, G. Zeng, X. Tan, B. Huang, X. Tang, S. Wang, Q. Hua and Z. Yan, J. Colloid Interface Sci., 2017, 506, 355–364 CrossRef CAS PubMed.
- M. Mohammadikish, M. Masteri-Farahani and S. Mahdavi, J. Magn. Magn. Mater., 2014, 354, 317–323 CrossRef CAS.
- G. Zhang, Y. Zhou, Z. Ding, L. Fu and S. Wang, RSC Adv., 2017, 7, 55215–55223 RSC.
- M. Mozaffari, M. R. S. Emami and E. Binaeian, Int. J. Biol. Macromol., 2019, 123, 457–467 CrossRef CAS PubMed.
- Y. Xing, X.-M. Sun and B.-H. Li, Environ. Eng. Sci., 2009, 26, 551–558 CrossRef CAS.
- R. Huang, G. Chen, M. Sun, Y. Hu and C. Gao, J. Membr. Sci., 2006, 286, 237–244 CrossRef CAS.
- K. M. Zia, S. Anjum, M. Zuber, M. Mujahid and T. Jamil, Int. J. Biol. Macromol., 2014, 66, 26–32 CrossRef CAS PubMed.
- F. Kara, E. A. Aksoy, Z. Yuksekdag, N. Hasirci and S. Aksoy, Carbohydr. Polym., 2014, 112, 39–47 CrossRef CAS PubMed.
- M. A. Javaid, R. A. Khera, K. M. Zia, K. Saito, I. A. Bhatti and M. Asghar, Int. J. Biol. Macromol., 2018, 115, 375–384 CrossRef CAS PubMed.
- Z. P. Demko and K. B. Sharpless, Angew. Chem., 2002, 114, 2217–2220 CrossRef.
- J. Kaur, M. Saxena and N. Rishi, Bioconjugate Chem., 2021, 32, 1455–1471 CrossRef CAS PubMed.
- C. D. Hein, X.-M. Liu and D. Wang, Pharm. Res., 2008, 25, 2216–2230 CrossRef CAS PubMed.
- I. Tijunelyte, E. Guenin, N. Lidgi-Guigui, F. Colas, J. Ibrahim, T. Toury and M. Lamy de la Chapelle, Nanoscale, 2016, 8, 7105–7112 RSC.
- J. Nicks and J. A. Foster, Nanoscale, 2022, 14, 6220–6227 RSC.
- Y. Shi, X. Cao and H. Gao, Nanoscale, 2016, 8, 4864–4881 RSC.
- H. A. Younus, M. Al-Rashida, A. Hameed, M. Uroos, U. Salar, S. Rana and K. M. Khan, Expert Opin. Ther. Pat., 2021, 31, 267–289 CrossRef CAS PubMed.
- S. E. John, S. Gulati and N. Shankaraiah, Org. Chem. Front., 2021, 8, 4237–4287 RSC.
- C. G. Neochoritis, T. Zhao and A. Dömling, Chem. Rev., 2019, 119, 1970–2042 CrossRef CAS PubMed.
- A. C. Boukis, K. Reiter, M. Frölich, D. Hofheinz and M. A. Meier, Nat. Commun., 2018, 9, 1–10 CrossRef CAS PubMed.
- R. Mittal and S. K. Awasthi, Synthesis, 2019, 51, 3765–3783 CrossRef CAS.
- V. Ostrovskii, E. Popova and R. Trifonov, in Advances in heterocyclic chemistry, Elsevier, 2017, vol. 123, pp. 1–62 Search PubMed.
- A. Singh and R. Kumar, Chem. Commun., 2021, 57, 9708–9711 RSC.
- R. J. Herr, Bioorg. Med. Chem., 2002, 10, 3379–3393 CrossRef CAS PubMed.
- L. V. Myznikov, S. V. Vorona and Y. E. Zevatskii, Chem. Heterocycl. Compd., 2021, 57, 224–233 CrossRef CAS.
- E. A. Popova, R. E. Trifonov and V. A. Ostrovskii, Russ. Chem. Rev., 2019, 88, 644 CrossRef CAS.
- N. R. Penthala, S. Bommagani, J. Yadlapalli and P. A. Crooks, Tetrahedron Lett., 2016, 57, 1807–1810 CrossRef CAS.
- N. Kaushik, N. Kumar, A. Kumar and U. K. Singh, Immunol., Endocr. Metab. Agents Med. Chem., 2018, 18, 3–21 CrossRef CAS.
- N. M. Aljamali, R. M. U. Mahmood and R. A. Baqi, Int. J. Anal. Appl. Chem., 2020, 6, 50–60 Search PubMed.
- A. Nguyen, E. Dallerba and A. B. Lowe, Macromol. Rapid Commun., 2022, 2200096 CrossRef CAS PubMed.
- J. H. Mohammed, Eur. Acad. Res., 2016, 3, 12803 Search PubMed.
- M. Asif, Pharm. Methods, 2014, 5, 39–46 Search PubMed.
- P. Mohite and V. Bhaskar, J. Optoelectron. Biomed. Mater., 2011, 3, 87–93 Search PubMed.
- V. Ostrovskii, R. Trifonov and E. Popova, Russ. Chem. Bull., 2012, 61, 768–780 CrossRef CAS.
- L. Li, T. Wang, Z. Xu, W. Zhou and X.-F. Yu, Nanoscale, 2022, 14, 3929–3934 RSC.
- S. Song, T. Yang, R. Shi and Q. Li, RSC Adv., 2021, 11, 711–720 RSC.
- S. Swami, S. N. Sahu and R. Shrivastava, RSC Adv., 2021, 11, 39058–39086 RSC.
- H. Tourani, M. R. Naimi-Jamal and M. G. Dekamin, ChemistrySelect, 2018, 3, 8332–8337 CrossRef CAS.
- U. Bhatt, Modern Heterocyclic Chemistry, 2011, pp. 1401–1430 Search PubMed.
- F. Himo, Z. P. Demko, L. Noodleman and K. B. Sharpless, J. Am. Chem. Soc., 2003, 125, 9983–9987 CrossRef CAS PubMed.
- S. Zhao, S. Zhang, J. Xu and L. Hu, Tetrahedron, 2020, 76, 131517 CrossRef CAS.
- F. Matloubi Moghaddam, M. Eslami and N. Ghadirian, Sci. Iran., 2019, 26, 1463–1473 Search PubMed.
- M. Nikoorazm, P. Moradi and N. Noori, J. Porous Mater., 2020, 27, 1159–1169 CrossRef CAS.
- M. Nasrollahzadeh, Y. Bayat, D. Habibi and S. Moshaee, Tetrahedron Lett., 2009, 50, 4435–4438 CrossRef CAS.
- M. L. Kantam, K. B. Shiva Kumar and K. Phani Raja, J. Mol. Catal. A: Chem., 2006, 247, 186–188 CrossRef CAS.
- R. Shelkar, A. Singh and J. Nagarkar, Tetrahedron Lett., 2013, 54, 106–109 CrossRef CAS.
- E. Aali, M. Gholizadeh and N. Noroozi-Shad, J. Mol. Struct., 2022, 1247, 131289 CrossRef CAS.
- A. H. Fattahi, M. G. Dekamin and J. H. Clark, Sci. Rep., 2023, 13, 2803 CrossRef CAS PubMed.
- B. Fattahi and M. G. Dekamin, Sci. Rep., 2023, 13, 401 CrossRef CAS PubMed.
- M. G. Dekamin, E. Arefi and A. Yaghoubi, RSC Adv., 2016, 6, 86982–86988 RSC.
- A. Zebardasti, M. G. Dekamin, E. Doustkhah and M. H. N. Assadi, Inorg. Chem., 2020, 59, 11223–11227 CrossRef CAS PubMed.
- P. Akbarzadeh, N. Koukabi and E. Kolvari, Res. Chem. Intermed., 2019, 45, 1009–1024 CrossRef CAS.
- Z. N. Tisseh, M. Dabiri, M. Nobahar, H. R. Khavasi and A. Bazgir, Tetrahedron, 2012, 68, 1769–1773 CrossRef CAS.
- Z. Hajizadeh, F. Hassanzadeh-Afruzi, D. F. Jelodar, M. R. Ahghari and A. Maleki, RSC Adv., 2020, 10, 26467–26478 RSC.
- M. Khodamorady and K. Bahrami, Chemistryselect, 2019, 4, 8183–8194 CrossRef CAS.
- N. Ahmed and Z. N. Siddiqui, RSC Adv., 2015, 5, 16707–16717 RSC.
- E. Valiey and M. G. Dekamin, RSC Adv., 2022, 12, 437–450 RSC.
- A. Nouri Parouch, N. Koukabi and E. Abdous, Res. Chem. Intermed., 2020, 46, 3295–3310 CrossRef CAS.
- P. Akbarzadeh, N. Koukabi and M. M. Hosseini, J. Heterocycl. Chem., 2020, 57, 2455–2465 CrossRef CAS.
- J. Safaei-Ghomi and S. Paymard-Samani, Chem. Heterocycl. Compd., 2015, 50, 1567–1574 CrossRef CAS.
- X. Yuan, Z. Wang, Q. Zhang and J. Luo, RSC Adv., 2019, 9, 23614–23621 RSC.
- C. Behloul, M. Benlahrech, F. Foubelo, C. Nájera and M. Yus, Synthesis, 2018, 50, 3430–3435 CrossRef CAS.
- B. Chattopadhyay, C. I. R. Vera, S. Chuprakov and V. Gevorgyan, Org. Lett., 2010, 12, 2166–2169 CrossRef CAS PubMed.
- J. Bonnamour and C. Bolm, Chem.–Eur. J., 2009, 15, 4543–4545 CrossRef CAS PubMed.
- S. Khaghaninejad, M. M. Heravi, T. Hosseinnejad, H. A. Oskooie and M. Bakavoli, Res. Chem. Intermed., 2016, 42, 1593–1610 CrossRef CAS.
|
This journal is © The Royal Society of Chemistry 2023 |
Click here to see how this site uses Cookies. View our privacy policy here.