DOI:
10.1039/D3RA01812A
(Paper)
RSC Adv., 2023,
13, 14355-14360
Introducing a sulfone-embedded anhydride to the anhydride-imine reaction for the modular synthesis of N-heterocyclic sulfones bearing vicinal stereocenters†
Received
20th March 2023
, Accepted 1st May 2023
First published on 10th May 2023
Abstract
N-heterocyclic sulfones constitute the core of several pharmaceuticals, including the antityrpanosomal drug Nifurtimox. Their biological relevance and architectural complexity makes them valued targets and inspires the development of more selective and atom-economical strategies for their construction and post-modification. In this embodiment, we describe a flexible approach to sp3-rich N-heterocyclic sulfones, which hinges on the efficient annulation of a novel sulfone-embedded anhydride with 1,3-azadienes and aryl aldimines. Further elaboration of the lactam esters has facilitated the construction of a library of vicinally functionalized sulfone-embedded N-heterocycles.
Introduction
Biologically active chemical space continues to be more biased toward sp3-rich compounds, in part due to their conformational rigidity, excellent target specificity, and high three-dimensional shape complementarity. There is enormous evidence that molecular complexity, as measured by parameters such as the number of tetrahedral stereocenters and fraction of saturated carbon atoms (Fsp3), correlates with success rates in drug development.1 These observations notwithstanding, current drug-like libraries are still relatively short on three-dimensionality and skeletal diversity.2 A strategic and conscious effort to escape flatland and move toward approaches that efficiently create non-planar scaffolds undeniably represents an important research endeavor.3
N-heterocyclic sulfones (examples of which are depicted in Fig. 1) are commonplace structural motifs in small-molecule pharmaceuticals. For example, diazoxide (I) is a potassium channel activator, which is often used as a vasodilator in the treatment of acute hypertension or malignant hypertension.4 Other examples of bioactive N-heterocyclic sulfones include Trancopal (II),5 r-secretase inhibitor III,6 Nifurtimox® (IV),7 Artemisone (V),8 and Ciprofloxacin derivative VI.9 Fittingly, the biological relevance of these aforementioned motifs and their architectural complexity endear them to the medicinal and synthesis communities, thus, inspiring the development of more flexible, efficient, and cost-effective strategies for their construction, peripheral functionalization, and evaluation of structure–activity relationships.10
 |
| Fig. 1 Examples of bioactive N-heterocyclic sulfones. | |
From the perspective of chemical synthesis, sulfones are implicit in classic transformations such as the Ramberg–Backlund reaction11 and Julia olefination.12 As exemplified through the synthesis of antitrypanosomal drug, Nifurtimox®,13 N-heterocyclic sulfones are typically prepared by the late-stage oxidation of a precursor thioether.14 However, the use of this late-stage oxidative route sometimes precludes the presence of oxidation-sensitive functional groups.
Our prior attempts to oxidize in-house allylic thiomorpholinones such as 3 (Fig. 2A), obtained from thiodiglycolic (1) anhydride and 1,3-azadienes of type 2,15 to the corresponding N-heterocyclic sulfones, have so far been low-yielding due to challenges associated with chemoselectivity and stability. Meanwhile, Cossy and co-workers have reported an intramolecular dehydrative coupling approach to allylic N-heterocyclic sulfones, which features early-stage installation of the sulfone moiety prior to cyclization (Fig. 2B).16 Inspired by the pharmaceutical relevance of sp3-rich N-heterocyclic sulfones, we sought to evaluate the performance of anhydride 6 in formal cycloaddition reactions featuring several imines such as 7 (Fig. 2C). Gleaning from Shaw's decarboxylative annulation tactic featuring sulfone-bearing glutaric anhydrides and imines (Fig. 2D),17 we recognized that the electron-withdrawing capability of the sulfone would enhance the reactivity of 6 and potentially obviate the need for high temperatures and stringent cryogenic conditions. We herein report ways to create diverse sp3-enriched N-heterocyclic sulfones with considerable diversity. The approach is modular, scalable, and amenable to post-diversification.
 |
| Fig. 2 (a) Prior synthesis of allylic thiomorpholinones (b) Cossy's approach to allylic N-heterocyclic sulfones (c) proposed plan for accessing sp3-enriched N-heterocyclic sulfones (d) Shaw's AMR featuring sulfone-substituted glutaric anhydrides. | |
Results and discussion
We commenced studies on the construction and post-diversification of sp3-rich N-heterocyclic sulfones by assembling anhydride 6 using a scalable two-step sequence, featuring oxidation of commercially available thioether 12 and subsequent cyclization (Scheme 1).
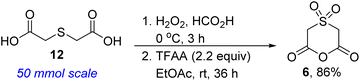 |
| Scheme 1 Synthesis of sulfone-embedded anhydride 6. | |
One of the prominent features of the three-component reaction between amines, aldehydes, and enolizable cyclic anhydrides (i.e., the Castagnoli–Cushman reaction (CCR) or anhydride-Mannich reaction (AMR)), is its exceptional atom-economy, given that with the exception of the water formed during the formation of the Schiff base, all atoms originating from the starting materials are incorporated into the product structure. With anhydride 6 in hand, its performance in annulation protocols featuring α,β-unsaturated imines of type 7a (R′ = alkenyl) was evaluated. Intrinsic to our design of commencing this investigation with 7a was the observation that allylic cyclic amines are resident in many alkaloids, including pinidine and aurantioclavine.18 We were also keen on accessing allylic N-heterocyclic sulfones upon recognizing that the alkenyl motif could pave the way for harnessing several reactivity modes, including hydroarylation,19 oxoamination,20 trifluoromethylation,21 oxacyclopropanation,22 aziridination,23 and boration.24 We were however not oblivious to the promiscuous reactivity of 7a with cyclic anhydrides bearing relatively acidic α-CH protons. For example, homophthalic anhydride reacts with 7a to afford a plethora of products, including Tamura-like, Castagnoli-like, and Perkin-type products. Meanwhile, 7a reacts efficiently with glutaric anhydride and diglycolic anhydride, but modestly with thiodiglycolic anhydride to furnish exclusively the Castagnoli–Cushman products.15 At the outset, we questioned if the strong electron-withdrawing prowess of the sulfone group would over enhance the α-CH acidity of 6, leading to an increase in reactivity, but also to a compromise in chemoselectivity. Nevertheless, we proceeded to test the feasibility of the annulation reaction using model 1,3-azadiene 7a1. Ultimately, we established that 2-methyltetrahydrofuran (2-MeTHF) out-performs other reaction media with respect to efficiency, chemo- and stereoselectivity. The exclusive formation of 8a1 (Scheme 2) suggests a pathway whereby anhydride-imine formal cycloaddition (CCR) predominates over anhydride-alkene formal cycloaddition (Tamura reactivity). For purposes of easier isolation and purification, the initially formed carboxylic acid has been converted into the corresponding methyl ester in all but one case.
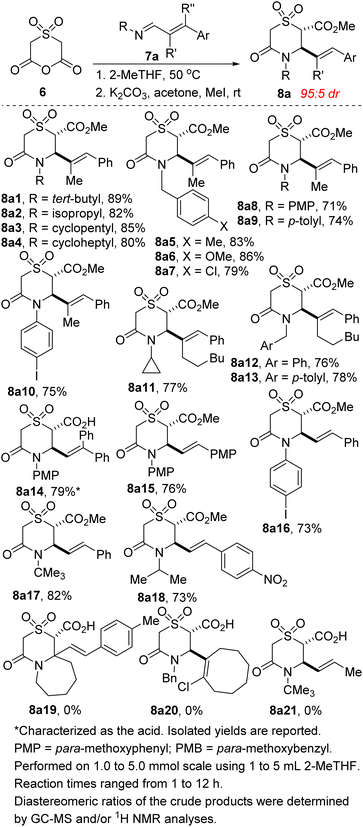 |
| Scheme 2 Annulation of 1,3-azadienes with sulfone-embedded anhydride 6. | |
The scope of the reaction with respect to the sterics and electronics of the N-substituent as well as the alkenyl moiety was then explored. We find that 1,3-azadienes bearing diverse substituents on nitrogen react with varying degrees of success (8a1–8a10). Significantly, from the standpoint of modularity, 1,3-azadienes harboring both electron-rich and electron-deficient styryl groups react competently (8a6 vs. 8a7). There is little to no compromise in the E/Z stereoselectivity of the alkene when the internal substituent is changed from methyl to n-hexyl (8a5 vs. 8a13). Halogenated styrenes and anilines are well tolerated (see 8a7, 8a10, and 8a16), which bodes well for late-stage diversification as the halogen group may be utilized as a functional handle for cross-coupling purposes (see Schemes 4 and 5).
Our studies show that the annulation of 6 with externally substituted 1,3-azadienes derived from β-aryl cinnamaldehydes is possible (see 8a14). Diarylethenes of type 8a14 are highly desirable given their prevalence in molecular motors.25 It is a testament to the enhanced reactivity of 6 that it undergoes satisfactory annulation with highly electron-deficient α,β-unsaturated imines (see 8a18) since the use of strongly deactivating p-nitrobenzaldehyde in the CCR is a rarity.17 No concomitant decarboxylation is observed in all of these examples, as was the case in Shaw's studies.17 Not all 1,3-azadienes that we have surveyed undergo productive cyclization with anhydride 6. For steric and/or fragility reasons, products 8a19–21 were not formed under the identified reaction conditions. Although the crucial philosophical criterion of green chemistry (i.e., atom economy) is clearly fulfilled in the CCR, it is yet to be considered as a tool for sustainable synthetic chemistry.26 This is presumably because the reaction is typically performed in high-boiling aromatic hydrocarbon solvents (e.g., toluene or xylenes). It is our view that the successful use of 2-MeTHF as the reaction medium in these studies will help confer CCR methodology the coveted ‘green chemistry status’ given the well-documented merits of 2-MeTHF.27
Following successful construction of sp3-enriched vicinally functionalized allylic N-heterocyclic sulfones, and owing to the continuing emergence of sulfone-embedded, aryl-substituted cyclic amines with medicinal potential (see Trancopal, Fig. 1), we next sought to extend the scope of the annulation reaction to aryl aldimines of type 7b. In the event, we find that this base- and transition metal-free annulation proceeds with good tolerance for several aryl aldimines (8b1–8b14). N-Alkyl, allyl, and benzyl substituents are well tolerated. However, the efficiency is somewhat compromised when N-aryl substituents are employed (see 8b13/14).
One of the merits of this methodology is the scalable nature of the reactions given that anhydride 6 and some of its annulation products (e.g., 8a10 and 8a16) have been prepared in gram scale, with little compromise in efficiency.28 This has set the stage for post-diversification studies. For example, iodide 8a16 undergoes efficient Cu-catalyzed alkenylation29 with electronically diverse (i.e., electron-rich, electron-neutral, and electron-deficient) styrenes to afford the N-stilbeno cyclic sulfones depicted in Scheme 3. A highly electron-deficient 2-vinylpyridinyl group can be stereoselectively installed in synthetically useful yield (see 13c). Additionally, a vinylthiazole derivative also couples prudently to afford sulfone 13d. This protocol is not limited to styrene coupling partners given that coupling with an electron-deficient vinyl sulfone proceeds stereoselectively to furnish bis-sulfone 13e. In this case, linear regioselectivity is also observed. The successful functional group-tolerant, E-stereoselective and linear-regioselective alkenylation described herein is noteworthy given the prevalence of the styrene and stilbene structural motifs in natural products, pharmaceuticals, and fragrances.30
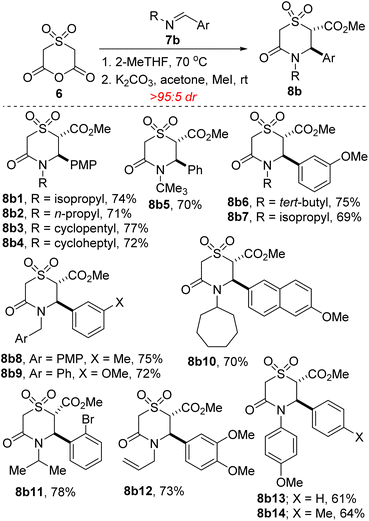 |
| Scheme 3 Annulation of aryl aldimines with sulfone-embedded anhydride 6. | |
 |
| Scheme 4 Cu-catalyzed alkenylation of iodoarylated sulfone 8a16 with electronically diverse alkenes. | |
Alkynes are fundamental building blocks in synthetic chemistry and in material science.31 Aryl alkynes are readily prepared from aryl halides by the Sonogashira reaction.32 Meanwhile, nickel is a privileged metal in cross-couplings from the standpoint of versatility and cost.33 We were therefore pleased to find that Ni-catalyzed coupling of iodoarylated sulfones 8a10 and 8a16 with terminal alkynes proceeds smoothly to afford the alkynylated sulfones depicted in Scheme 5 (see 14a–e). These directing group-free34 conditions employ Cs2CO3 as the base and 1,4-dioxane as the reaction medium. The copper co-catalyst, CuI, is desirable seeing as the reaction does not proceed under copper-free conditions.
 |
| Scheme 5 Ni-catalyzed alkynylation of iodoarylated sulfones with terminal alkynes. | |
Regarding the mechanistic underpinnings of the anhydride-imine annulation protocol described herein, congruent with prior studies,35 we postulate that the reaction could proceed via either of two possible paths: (a) intermolecular imine acylation followed by intramolecular Mannich reaction and/or (b) intermolecular Mannich reaction followed by intramolecular acylation or anhydride aminolysis. In the current scenario, thermally-assisted tautomerization of anhydride 6 affords enol 15 (Fig. 3), which undergoes Mannich-type addition with 7a1 to afford formal hydroalkylation product 16. In many instances in the past and present, we have isolated intermediates of type 16 at partial conversion, which lends credence to our working hypothesis. Subsequent intramolecular aminolysis of the anhydride by the pendant secondary amine resident in 16 gives rise to the desired lactam acid. We note however that the iminolysis pathway (see 17 and 18) is yet to be completely ruled out at this point.
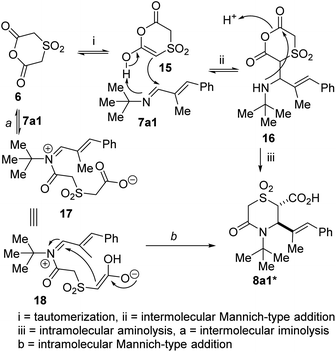 |
| Fig. 3 Proposed mechanism for the annulation of 6 with imines (formation of the acid that leads to 8a1 is chosen for simplicity). | |
Conclusions
In summary, the modular construction of densely populated allylic and benzylic N-heterocyclic sulfones has been accomplished using a readily affordable sulfone-embedded cyclic anhydride. The scalable nature of the reactions offers the opportunity for post-modification by incorporation of motifs with either known pharmaceutical value or that permit subsequent conversion (esters, alkenes, and alkynes) to medicinally relevant analogues. The criteria of efficiency, versatility, sustainability, and pot–atom–step economy are of paramount importance and these studies have met these benchmarks. The amenability of these functionalized intermediates to C–C bond forming transformations bodes well for future late-stage assembly of complex bioactive N-heterocyclic sulfones. We anticipate that the aforementioned merits will endear this methodology to both the synthesis and medicinal chemistry communities.
Experimental
All experiments involving air and moisture sensitive reagents were carried out under an inert atmosphere of nitrogen and using freshly distilled solvents. Column chromatography was performed on silica gel (230–400 mesh). Thin-layer chromatography (TLC) was performed using Silicycle Siliaplate™ glass backed plates (250 μm thickness, 60 Å porosity, F-254 indicator) and visualized using UV (254 nm) or KMnO4 stain. Unless otherwise indicated, 1H, 13C, and DEPT-135 NMR, and NOESY spectra were acquired using CDCl3 solvent at room temperature. Chemical shifts are quoted in parts per million (ppm). HRMS-EI+ data were obtained using either electronspray ionization (ESI) or electron impact (EI) techniques. High-resolution ESI was obtained on an LTQ-FT (ion trap; analyzed using Excalibur). High resolution EI was obtained on an Autospec (magnetic sector; analyzed using MassLynx). Brine solutions are saturated solutions of aqueous sodium chloride. Characterization data for all compounds are provided in the ESI.†
Author contributions
J. G. – investigation, data curation, methodology; J. E. – investigation, data curation, methodology; C. B. – investigation, data curation, methodology; T. K. B. – conceptualization, project administration, supervision, investigation, data curation, methodology, writing – original draft, funding acquisition.
Conflicts of interest
There are no conflicts of interest to declare.
Acknowledgements
We are grateful to Central Washington University (CWU) for financial support through the donation of startup funds to T. K. B. The School of Graduate Studies is thanked for a research fellowship to T. K. B. J. G., J. E., and C. B. are grateful to the Office of Undergraduate Research (OUR) and to Provost Denbeste for research fellowships. We thank Organic Syntheses, Inc for partial support of this project.
Notes and references
- For reviews, see:
(a) A. Karawajczyk, F. Giordanetto, J. Benningshof, D. Hamza, T. Kalliokoski, K. Pouwer, R. Morgentin, A. Nelson, G. Müller, A. Piechot and D. Tzalis, Drug Discovery Today, 2015, 20, 1310–1316 CrossRef CAS PubMed
;
(b) F. Lovering, J. Bikker and C. Humblet, J. Med. Chem., 2009, 52, 6752–6756 CrossRef CAS PubMed
;
(c) T. J. Ritchie and S. J. F. Macdonald, Drug Discovery Today, 2009, 14, 1011–1020 CrossRef CAS PubMed
. - J. Meyers, M. Carter, N. Y. Mok and N. Brown, Future Med. Chem., 2016, 8, 1753–1767 CrossRef PubMed
. -
(a) B. L. Donnelly, L. D. Elliott, C. L. Willis and K. I. Booker-Milburn, Angew. Chem., Int. Ed., 2019, 58, 9095–9098 CrossRef CAS PubMed
;
(b) A. Sveiczer, A. J. P. North, N. Mateu, S. L. Kidd, H. F. Sore and D. R. Spring, Org. Lett., 2019, 21, 4600–4604 CrossRef CAS PubMed
;
(c) N. J. Flodén, A. Trowbridge, D. Willcox, S. M. Walton, Y. Kim and M. J. Gaunt, J. Am. Chem. Soc., 2019, 141, 8426–8430 CrossRef PubMed
;
(d) T. G. Chen, L. M. Barton, Y. Lin, J. Tsien, D. Kossler, I. Bastida, S. Asai, C. Bi, J. S. Chen, M. Shan, H. Fang, F. G. Fang, H.-W. Choi, L. Hawkins, T. Qin and P. S. Baran, Nature, 2018, 560, 350–354 CrossRef CAS PubMed
;
(e) D. J. Foley, P. G. E. Craven, P. M. Collins, R. G. Doveston, A. Aimon, R. Talon, I. Churcher, F. von Delft, S. P. Marsden and A. Nelson, Chem.–Eur. J., 2017, 23, 15227–15232 CrossRef CAS PubMed
;
(f) R. Gianatassio, J. M. Lopchuk, J. Wang, C.-M. Pan, L. R. Malins, L. Prieto, T. A. Brandt, M. R. Collins, G. M. Gallego, N. W. Sach, J. E. Spangler, H. Zhu, J. Zhu and P. S. Baran, Science, 2016, 351, 241–246 CrossRef CAS PubMed
;
(g) B. A. Chalmers, H. Xing, S. Houston, C. Clark, S. Ghassabian, A. Kuo, B. Cao, A. Reitsma, C.-E. P. Murray, J. E. Stok, G. M. Boyle, C. J. Pierce, S. W. Littler, D. A. Winkler, P. V. Bernhardt, C. Pasay, J. J. De Voss, J. McCarthy, P. G. Parsons, G. H. Walter, M. T. Smith, H. M. Cooper, S. K. Nilsson, J. Tsanaktsidis, G. P. Savage and C. M. Williams, Angew. Chem., Int. Ed., 2016, 55, 3580–3585 CrossRef CAS PubMed
;
(h) M. U. Luescher and J. W. Bode, Org. Lett., 2016, 18, 2652–2655 CrossRef CAS PubMed
. - H. W. V. Hamersvelt, H. J. Kloke, D. J. D. Jong, R. A. Koene and F. T. Huysmans, J. Hypertens., 1996, 14, 1041–1045 Search PubMed
. - U. Wollina, U. Hipler, A. Seeling and H. Oelschlager, Skin Pharmacol. Physiol., 2005, 18, 132–135 CrossRef CAS PubMed
. - Y. Liu, W. Qin and H. Yan, Synlett, 2016, 27, 2756 CrossRef CAS
. - J. Pepin, F. Milord, F. Meurice, L. Ethier, L. Loko and B. Mpia, Trans. R. Soc. Trop. Med. Hyg., 1992, 86, 254–256 CrossRef CAS PubMed
. -
(a) R. K. Haynes, B. Fugmann, J. Stetter, K. Rieckmann, H.-D. Heilmann, H.-W. Chan, S. L. Croft, L. Vivas, L. Rattray, L. Stewart, W. Peters, B. L. Robinson, M. D. Edstein, B. Kotecka, D. E. Kyle, B. Beckermann, M. Gerisch, M. Radtke, G. Schmuck, W. Steinke, U. Wollborn, K. Schmeer and A. Römer, Angew. Chem., Int. Ed., 2006, 45, 2082 CrossRef CAS PubMed
;
(b) J. Nagelschmitz, B. Voith, G. Wensing, A. Römer, B. Fugmann, R. K. Haynes, B. M. Kotecka, K. H. Rieckmann and M. D. Edstein, Antimicrob. Agents Chemother., 2008, 52, 3085 CrossRef CAS PubMed
. - X. Huang, D. Chen, N. Wu, A. Zhang, Z. Jia and X. Li, Bioorg. Med. Chem. Lett., 2009, 19, 4130 CrossRef CAS PubMed
. -
(a) T. Sasaki, T. Takahashi, T. Nagase, T. Mizutani, S. Ito, Y. Mitobe, Y. Miyamoto, M. Kanesaka, R. Yoshimoto, T. Tanaka, N. Takenaga, S. Tokita and N. Sato, Bioorg. Med. Chem. Lett., 2009, 19, 4232 CrossRef CAS PubMed
;
(b) S. Menichetti, M. C. Aversa, F. Cimino, A. Contini, C. Viglianisi and A. Tomaino, Org. Biomol. Chem., 2005, 3, 3066 RSC
;
(c) A. Regueiro-Ren, Z. Liu, Y. Chen, N. Sin, S. Y. Sit, J. J. Swidorski, J. Chen, B. L. Venables, J. Zhu, B. Nowicka-Sans, T. Protack, Z. Lin, B. Terry, H. Samanta, S. Zhang, Z. Li, B. R. Beno, X. S. Huang, S. Rahematpura, D. D. Parker, R. Haskell, S. Jenkins, K. S. Santone, M. I. Cockett, M. Krystal, N. A. Meanwell, U. Hanumegowda and I. B. Dicker, ACS Med. Chem. Lett., 2016, 7, 568 CrossRef CAS PubMed
. - C. W. Meyers, A. M. Malte and W. S Matthews, J. Am. Chem. Soc., 1969, 91, 7510 CrossRef CAS
. - M. Julia and J.-M. Paris, Tetrahedron Lett., 1973, 14, 4833 CrossRef
. - A. R. Surrey, W. G. Webb and R. M. Gesler, J. Am. Chem. Soc., 1958, 13, 3469 CrossRef
. -
(a) W. Su, Tetrahedron Lett., 1994, 35, 4955 CrossRef CAS
;
(b) N. K. Jana and J. G. Verkade, Org. Lett., 2003, 5, 3787 CrossRef CAS PubMed
. - H. Braunstein, S. Langevin, M. Khim, J. Adamson, K. Hovenkotter, L. Kotlarz, B. Mansker and T. K. Beng, Org. Biomol. Chem., 2016, 14, 8864–8872 RSC
. - C. Bosset, G. Lefebvre, P. Angibaud, I. Stansfield, L. Meerpoel, D. Berthelot, A. Guérinot and J. Cossy, Beilstein J. Org. Chem., 2017, 82, 4020–4036 CrossRef CAS PubMed
. - N. A. Sorto, M. J. Di Maso, M. A. Munoz, R. A. Dougherty, J. C. Fettinger and J. T. Shaw, J. Org. Chem., 2014, 79, 2601–2610 CrossRef CAS PubMed
. -
(a) J. N. Tawara, A. Blokhin, T. A. Foderaro, F. R. Stermitz and H. Hope, J. Org. Chem., 1993, 58, 4813–4818 CrossRef CAS
;
(b) T. J. Wilkinson, N. W. Stehle and P. Beak, Org. Lett., 2000, 2, 155–158 CrossRef CAS PubMed
. - K. Brak and J. A. Ellman, Org. Lett., 2010, 12, 2004–2007 CrossRef CAS PubMed
. - B. Ye and N. Cramer, Acc. Chem. Res., 2015, 48, 1308–1318 CrossRef CAS PubMed
. - P. K. Prasad, R. N. Reddi and A. Sudalai, Org. Lett., 2016, 18, 500–503 CrossRef CAS PubMed
. - A. Deb, S. Manna, A. Modak, T. Patra, S. Maity and D. Maiti, Angew. Chem., Int. Ed., 2013, 52, 9747–9750 CrossRef CAS PubMed
. - D. T. Smith and J. T. Njardarson, Angew. Chem., Int. Ed., 2014, 53, 4278–4280 CrossRef CAS PubMed
. - W. Su, T.-J. Gong, X. Lu, M.-Y. Xu, C.-G. Yu, Z.-Y. Xu, H.-Z. Yu, B. Xiao and Y. Fu, Angew. Chem., Int. Ed., 2015, 54, 12957–12961 CrossRef CAS PubMed
. - A. Faulkner, T. van Leeuwen, B. L. Feringa and S. J. Wezenberg, J. Am. Chem. Soc., 2016, 138, 13597–13603 CrossRef CAS PubMed
. - R. C. Cioc, E. Ruijter and R. V. A. Orru, Green Chem., 2014, 16, 2958 RSC
. - A. R. Alcantara and P. D. de Maria, Curr. Green Chem., 2018, 5, 88 Search PubMed
. - Aryl iodide 8a10 was prepared in 3 mmol scale and 8a16 was prepared in 5 mmol scale.
- T. K. Beng and A. Moreno, New J. Chem., 2020, 44, 4257–4261 RSC
. -
(a) A. Sarkic and I. Stappen, Cosmetics, 2018, 5, 11 CrossRef
;
(b) H. Satoh and K. Takeuchi, Curr. Med. Chem., 2012, 19, 82–89 CrossRef CAS PubMed
;
(c) M. H. Keylor, B. S. Matsuura and C. R. Stephenson, Chem. Rev., 2015, 115, 8976–9027 CrossRef CAS PubMed
. -
(a) S. Diez-Gonzalez, Catal. Sci. Technol., 2011, 1, 166 RSC
;
(b) A. Palisse and S. F. Kirsch, Org. Biomol. Chem., 2012, 10, 8041 RSC
;
(c) I. V. Alabugin and B. Gold, J. Org. Chem., 2013, 78, 7777 CrossRef CAS PubMed
;
(d) R. Hu, J. W. Y. Lam and B. Z. Tang, Macromol. Chem. Phys., 2013, 214, 175 CrossRef CAS
;
(e) R. Chinchilla and R. C. Nájera, Chem. Rev., 2014, 114, 1783 CrossRef CAS PubMed
. - R. Chinchilla and C. Nájera, Chem. Soc. Rev., 2011, 40, 5084 RSC
. - B. M. Rosen, K. W. Quasdorf, D. A. Wilson, N. Zhang, A. M. Resmerita, N. K. Garg and V. Percec, Chem. Rev., 2011, 111, 1346 CrossRef CAS PubMed
. - A. O. Farah, R. Archibald, M. J. Rodriguez, A. Moreno, B. Dondji and T. K. Beng, Tetrahedron Lett., 2018, 59, 3495–3498 CrossRef CAS
. -
(a) M. Krasavin and D. Darin, Tetrahedron Lett., 2016, 57, 1635–1640 CrossRef CAS
;
(b) M. Gonzalez-Lopez and J. T. Shaw, Chem.
Rev., 2009, 109, 164–189 CrossRef CAS PubMed
;
(c) J. Garcia, J. Eichwald, J. Zesiger and T. K. Beng, RSC Adv., 2022, 12, 309–318 RSC
;
(d) S. W. Laws, S. Y. Howard, R. Mato, S. Meng, J. C. Fettinger and J. T. Shaw, Org. Lett., 2019, 13, 5073–5077 CrossRef PubMed
;
(e) T. K. Beng, S. Langevin, A. O. Farah, J. Goodsell and K. Wyatt, New J. Chem., 2019, 43, 5282–5286 RSC
.
|
This journal is © The Royal Society of Chemistry 2023 |
Click here to see how this site uses Cookies. View our privacy policy here.