DOI:
10.1039/D3RA01214J
(Paper)
RSC Adv., 2023,
13, 12654-12662
A novel non-enzymatic electrochemical uric acid sensing method based on nanohydroxyapatite from eggshell biowaste immobilized on a zinc oxide nanoparticle modified activated carbon electrode (Hap-Esb/ZnONPs/ACE)
Received
23rd February 2023
, Accepted 11th April 2023
First published on 24th April 2023
Abstract
Hydroxyapatite-derived eggshell biowaste (Hap-Esb) has been fabricated and developed for the electrochemical detection of uric acid (UA). The physicochemical characteristics of the Hap-Esb and modified electrodes were evaluated using a scanning electron microscope and X-ray Diffraction analysis. Utilized as UA sensors, the electrochemical behavior of modified electrodes (Hap-Esb/ZnONPs/ACE) was assessed using cyclic voltammetry (CV). The superior peak current response observed for the oxidation of UA at Hap-Esb/ZnONPs/ACE, which was 13 times higher than that of the Hap-Esb/activated carbon electrode (Hap-Esb/ACE) is attributed to the simple immobilization of Hap-Esb on zinc oxide nanoparticle-modified ACE. The UA sensor exhibited a linear range at 0.01 to 1 μM, low detection limit (0.0086 μM), and excellent stability, which surpass the existing Hap-based electrodes reported in the literature. The facile UA sensor subsequently realized is also advantaged by its simplicity, repeatability, reproducibility, and low cost, applicable for real sample analysis (human urine sample).
Introduction
In the current field of disease diagnosis and treatment, there is a necessity to create a device that can identify, trace, and quantify the metabolites of several important biochemical processes in the human body for a fast, straight forward, and accurate diagnosis of a variety of disorders. Uric acid (UA) and its derivatives are required for biological activity in the human body. UA is a natural antioxidant and by product of purine metabolism.1,2 Excess UA concentration is associated with a number of diseases including gout, cardiovascular,3 hyperuricemia,4 and type II diabetes.5 Meanwhile, a lower amount of UA than its typical range may reflect an earlier stage of Parkinson's, Alzheimer's, or multiple sclerosis disease.6,7 Therefore, a device that can monitor UA has become important.
Several analysis methods, including high performance liquid chromatography,8,9 chemiluminescence analysis,10 spectrophotometry,11 and electrochemical analysis, have been used for UA analysis.12,13 Among them, electrochemical analysis has received more attention because of its fast, reliable, and accurate results.14–18 To date, most electrochemical UA sensing is enzymatic. This is because the UA molecules can react with a specific enzyme, uricase, resulting in good selectivity in the detection of UA.19,20 However, enzymatic sensing has several limitations, for example, the enzyme must be placed at a specific temperature (typically at 3–4 °C), have a short lifetime, and must be packed properly.21,22
Studies have proposed applying hydroxyapatite (Hap) as a non-enzymatic sensor for electrochemical detection of UA.23–25 Hap is a calcium phosphate compound with the chemical formula Ca10(PO4)6(OH)2. It can be synthesized by various techniques, including deposition, hydrolysis, hydrothermal synthesis, and/or extraction from natural resources, for example, eggshells, seashells, shrimp shells, bovine bones, and fish bones.26–28 Because of the high demand for eggs in food production, there is an abundance of eggshell biowaste (Esb). An eggshell is structurally composed of three layers: the cuticle, the spongy layer, and the lamellar layer.29,30 The spongeous and lamellar layers resemble a matrix of protein fibers tied to the calcium carbonate (calcite) crystal at a ratio of 1
:
50. The calcite content of an eggshell is approximately ∼94% wt, making it a good candidate for valorizing into Hap and using as UA sensing for electrochemical detection.23,31,32
The chemical structure of Hap and urate ions is presented in Fig. 1(a). As shown, the Hap has positive charges from Ca ions, while urate ions are negatively charged. Therefore, it is assumed that urate ions can be adsorbed onto the positively charged molecule of Hap, forming the Hap-UA complex (see Fig. 1(b)). However, as can be seen, in the Hap chemical structure, the negatively charged ions that are from the hydroxyl group (OH–) are also present. The (OH–) ions appear to play a significant role in electrochemistry thereby increasing the current response (reduction).32–37 Therefore, Hap could be used for the detection of UA with further modification of the electrode surface by applying a semiconductor material to improve the current response.
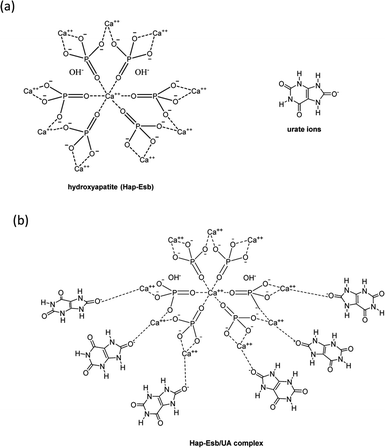 |
| Fig. 1 (a) Chemical structures of hydroxyapatite (Hap-Esb) and urate ions. (b) And proposed Hap-Esb/UA complexes. | |
Among various metal oxides, zinc oxide (ZnO) is a very promising semiconductor material.38 This is because ZnO has high electrocatalytic activity that facilitates better electron transport.16,39–44 The high isoelectric point of ZnO doubtless enables the improvement absorption of analytes, result in a greater current response.45–49 More importantly, ZnO is an environmentally friendly, cheap, highly stable material (both physically and chemically), biocompatible, and non toxic material.47,50,51 For the aforementioned reasons, we are interested in applying ZnO to modify our sensing.
In general, Hap can easily combine with the carbon family. Activated carbon, carbon nanotubes, and graphene are known for providing improved performance as supercapacitor electrodes.52–54 Activated carbon (AC) is frequently used as an electrode in the production of supercapacitors because AC has numerous advantages including inexpensive, easy to find, has a high porosity and highly scalable.55–57 As a result, in this present work, we further modify the Hap-Esb with ZnO nanoparticles and AC to create a non-enzymatic UA sensing system. The structure and surface morphology of the as synthesized Hap was characterized by scanning electron microscopy (SEM) and X-ray diffraction (XRD). While the performance of our proposed sensor in UA detection was evaluated by cyclic voltammetry (CV). In order to provide a feasibility study, we also observed the CV profiles of the urine sample as the real sample analysis.
Experimental section
Materials and instruments
Eggshell biowastes (Esb) were collected from the food waste located in Bandung City, Indonesia. Activated carbon, ZnONPs used were from the previous work. KH2PO4 (≥99.995%), and K2HPO4 (≥99.99%) are from Merck (Germany) with a pro analytical grade. Ultrapure water was prepared by a Module E-pure D4642-33 instrument (Barnstead) with a resistivity ≥18 MΩ.
The surface morphology of Hap-Esb, as well as electrode surface modification were observed using scanning electron microscopy (SEM; JEOL JSM IT300, Japan). The formation of Hap-Esb and the size of its crystallites were assessed using X-ray diffraction (XRD) patterns obtained with the D-8 Advance (Bruker) instrument. The Autolab PGSTAT101 Metrohm was used to record the electrochemical measurement.
CaO extraction and synthesis of Hap-Esb
In this investigation, the Esb were collected from a local market located (Bandung City, West Java, Indonesia) and rinsed repeatedly with tap water to remove impurities and dried under the sun. After drying, the clean Esb was crushed into coarse powder and then sieved with a 100-mesh size. The Esb powder was then transferred into a crucible to be calcinated at 1000 °C for 5 h in a furnace. Later, for the synthesis of Hap-Esb, about 21.23 g of CaO from Esb was dispersed in 500 mL of ultrapure water. Following that, 0.6 M H3PO4 was added to the solution at a flow rate of 6 mL min−1 until the ratio reached 1
:
1. The suspension was heated for 1 h, at 60 °C. The pH of the solution was adjusted to pH 10 by adding 25% v/v ammonia. Then the suspension was sonicated for 1 h (40 kHz) and kept at room temperature (RT) for overnight. Subsequently, the filtrate was separated by centrifugation (3000 rpm, 15 min). The precipitates were then collected and dried overnight in an oven at 105 °C. The dried precipitates were transferred to a crucible and calcined at 1000 °C for 5 hours. The as-synthesized Hap-Esb powders were then collected and characterized by SEM and XRD.
Preparation of bare and modified electrodes
Bare activated carbon electrode (ACE) was prepared by grinding 0.5 g of AC powder in a mortar with 100 μL paraffin oil for 30 minutes until a homogeneous paste was formed. Then the paste was packed into a capillary tube. A cooper wire was inserted into the capillary tube for electrical contact. The Hap-Esb/ZnO/ACE and Hap-Esb/ACE were prepared by a simple immobilization of the as-synthesized ZnONPs58 and Hap on the surface of ACE. The schematic preparation of Hap-Esb/ZnO NPs/ACE is presented in Fig. 2.
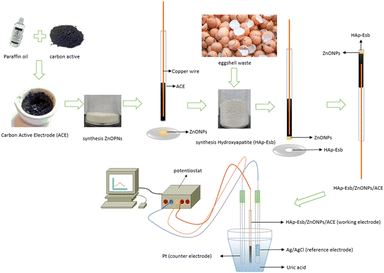 |
| Fig. 2 Schematic preparation of the Hap-Esb/ZnONPs/ACE. | |
Electrochemical measurements
A computerized potentiostat instrument (PGSTAT101 Metrohm, AutoLab) was linked to a computer equipped with software, NOVA 1.11 with a three-electrode cell (30 mL capacity) was employed for electrochemical measurement. A Pt-wire electrode (CE) (6.0301.100 Metrohm, AutoLab) and an Ag/AgCl reference electrode (RE) (6.0729.100 Metrohm, AutoLab) and the working electrode (WE) Hap-Esb/ZnONPs/ACE were used.
The cyclic voltammetry technique was used to study the electrochemical behavior as well as to evaluated the performance of the developed electrode using UA standard solutions and a healthy human urine sample for real sample analysis. The potentials window of −1.5 V to 1.8 V and a scan rate of 100 mV s−1 was employed. The UA standard solutions were prepared in 0.1 M phosphate buffer solution (PBS) pH 8, with various concentrations (0.01–1 μM). The sensor performance was also evaluated in the presence of interfering compounds that are ascorbic acid, glucose and tyrosine to confirm the selectivity in UA detection.
Results and discussion
Characterization of Hap-Esb
CaO was extracted from Esb by calcining the Esb for 5 hours at 1000 °C. High temperatures will decompose and eliminate the spongeous and lamellar matrix that are not thermally stable and are linked with the calcite from the eggshell.59,60 And the calcite from the Esb will be transformed into CaO in accordance with the reaction eqn (1).
The Hap-Esb was synthesized by mixing the CaO from Esb with a 0.6 M H3PO4 solution, followed by calcination at 1000 °C for 5 h. The heating stage is very important for the formation of Hap-Esb. The use of higher temperature, the more Hap produced.61 To confirm the formation of Hap from Esb (Hap-Esb), the XRD analysis was employed. The X-ray diffraction (XRD) pattern of the as-synthesized Hap-Esb was displayed in Fig. 3. The as-synthesized Hap-Esb clearly showed intense peaks at 2θ values of 10.84, 16.99, 21.85, 22.84, 25.84, 27.82, 28.91, 31.75, 32.16, 32.88, 34.03, 35.59, 38.53, 40.41, 41.96, 46.80, 49.44, 49.58, and 52.05 are assigned to (100), (101), (200), (111), (002), (102), (210), (211), (112), (300), (202), (301), (220), (310), (131), (113), (222), (213), and (322) planes respectively, matching with Hap JCPDS pattern of 9-432 (ref. 62) (see Fig. 3(a)). Next, the crystallite sizes of Hap-Esb were calculated using the Debye Scherer formula (see eqn (2)),
|
 | (2) |
where ‘
D’ is denotes for the crystallite size,
λ is the X-ray wavelength (1.54178 Å), ‘
β’ is the full width half maximum (FWHM) of the peak, and ‘2
θ’ is the Bragg angle.
63 The calculation of crystallite sizes from Hap-Esb was tabulated in
Table 1. As presented, the calculated crystallite size of Hap-Esb was found in the range of ∼48 to ∼107 nm. And the average of Hap-Esb crystallite sizes was ∼78 nm. The XRD analysis suggests that the as-synthesized Hap-Esb are nanoparticles.
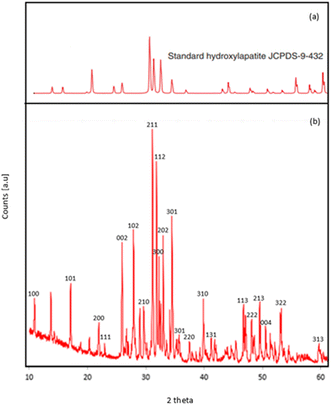 |
| Fig. 3 XRD pattern of (a) Hap (JCPDS card no. 9-432); (b) products Hap from eggshell obtained by precipitation method. | |
Table 1 Calculation of crystallite size of Hap from eggshell
2 theta position |
Crystallite size (nm) |
10.84 |
48.89 |
16.99 |
65.65 |
21.85 |
66.12 |
22.84 |
99.31 |
25.84 |
66.61 |
27.82 |
100.29 |
28.91 |
67.05 |
31.75 |
67.50 |
32.16 |
101.31 |
32.88 |
101.50 |
34.03 |
101.80 |
35.59 |
51.12 |
38.53 |
103.12 |
40.41 |
103.73 |
41.96 |
52.13 |
46.80 |
70.74 |
49.44 |
107.17 |
49.58 |
53.61 |
52.05 |
72.75 |
Average |
78.97 |
Fig. 4(a) shows an SEM image of as-synthesised Hap-Esb. As shown at 5000× magnification, the as-synthesized Hap-Esb were found in a mixture of coral shapes constructed with hexagonal rods and square tile-like shapes.64,65 Additionally, the Hap-Esb particles were found to agglomerate. The surface morphology of the modified ACE with ZnONPs is presented in Fig. 4(b). The ZnO NPs were indicated with white dots. From Fig. 4(b), it can be seen that the ZnO NPs are successfully attached to the surface of ACE and well dispersed. The surface morphology of Hap-Esb/ZnONPs/ACE is depicted in Fig. 4(c). As shown, white and cloudy particles are submicron in size and connected one to another, indicating an agglomeration of Hap-Esb particles.33,66–68 There are several factors that can cause agglomeration are (1) shear forces between particles, (2) initial density, (3) moisture, (4) sintering temperature, and (5) particle size.69–72 In this work, the synthesis procedure of Hap-Esb and the preparation of the developed electrode include grinding, wetting, and drying processes, possibly causing the agglomeration. The influence of particle size in agglomeration is emphasized by the calculation of Hap-Esb crystallite size by the Debye–Scherer equation, which suggests that the Hap-Esb are nanoparticles. Undoubtedly, they tend to agglomerate.63,71 Fig. 4(d) shows the surface morphology of Hap-Esb/ZnONPs/ACE after measurement. As shown, the Hap-Esb particles are detached from the ZnONPs-ACE surface, suggesting an instability issue after measurement.
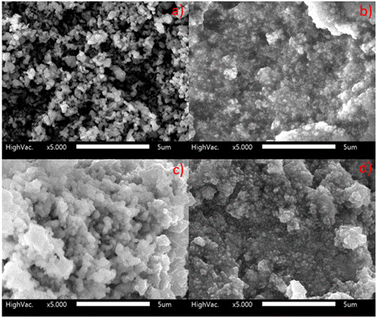 |
| Fig. 4 SEM images of (a) Hap synthesis from eggshell (Hap-Esb); (b) ZnONPs/ACE; (c) Hap-Esb/ZnONPs/ACE before used; (d) Hap-Esb/ZnONPs/ACE after used at 5000× magnification. | |
Cyclic voltammetry (CV) behavior of the modified electrode toward UA response
CV technique was applied to study the electrochemical behavior of the modified electrode toward 0.1 μM UA response in 0.1 M PBS solution (pH 8, potential range of −1.5 to 1.8 V, and a scan rate of 100 mV s−1). The cyclic voltammograms (CVs) for 0.1 μM UA at the bare ACE, Hap-Esb/ACE, ZnONPs/Hap-Esb/ACE, and Hap-Esb/ZnONPs/ACE are presented in Fig. 5(a). There were a good response in the peak at −0.53 V corresponding to UA electro-oxidation. The data showed that the greatest peak oxidation current response was observed at the Hap-Esb/ZnONPs/ACE (111.48 μA) surface in comparison with the bare ACE (30.6 μA), Hap-Esb/ACE (8.10 μA), and ZnONPs/Hap-Esb/ACE (24.02 μA) (see Fig. 5(b)), indicating the improved sensitivity of the developed electrode towards UA detection. It is very imperative to note that the layer arrangement on surface modification is significantly affects the currents response.73 When the ZnONPs layer was brought forward on the outermost surface of the developed sensor (ZnONPs/Hap-Esb/ACE), the ZnONPs blocked the Hap-Esb pore, reducing the current response. In reverse, when the ZnONPs were sent backward between Hap-Esb and ACE (Hap-Esb/ZnONPs/ACE), the current response was significantly higher than the bare ACE as well as the ZnONPs/Hap-Esb/ACE. Factors that affect the electrochemical response are (1) analyte diffusion, (2) catalysts, and (3) electron transfer.74 By placing the Hap at the outermost surface, this improves the diffusion of UA and promotes the complexation of urate ions with Ca2+ from the Esb-Hap.37,52 And the immobilization of ZnO NPs in the middle layer improves electron transfer, resulting in the highest current response.
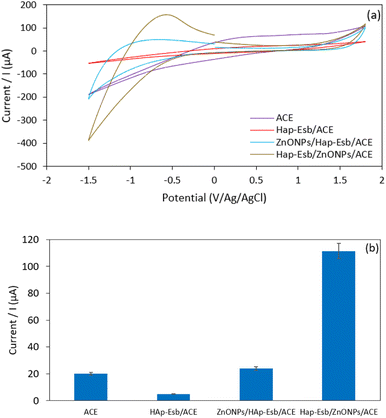 |
| Fig. 5 (a). The CV voltammogram and (b) histogram of peak current response of the bare, Hap-Esb, ZnONPs/Hap-Esb/ACE and Hap-Esb/ZnONPs/ACE. | |
Optimization of HAp-Esb/ZnONPs/ACE
The influence of pH. In electro-analytical evaluation, the pH of the solution significantly influences the current response since the protons are involved in the reaction.75 Over a pH range of 3 to 9, the effect of pH on the peak current response of UA at the Hap-Esb/ZnONPs/ACE in 0.1 M PBS was observed. Fig. 6 depicts CVs recorded in the presence of 0.1 μM UA at a scan rate of 100 mV s−1. The results show that the peak current response of UA increases gradually from pH 3 to 8, which can be attributed to the fact that Hap slowly dissolves in acidic solutions. It is also known that the Ca2+ ions of Hap-Esb form a complex with urate ions (see Fig. 1(b)).76,77 As a result, when Hap dissolves slowly in an acidic environment, it loses its ability to bond the urate ions, resulting in a lower current response. The maximum oxidation UA current was obtained at pH 8. Therefore, PBS at pH 8 was used as the electrolyte in subsequent experiments.
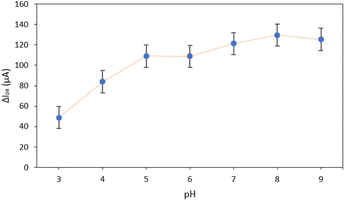 |
| Fig. 6 The anodic peak current response of 0.1 μM UA at the Hap-Esb/ZnONPs/ACE in PBS at pH ranging from 3 to 9. | |
Calibration plot and limit of detection. A calibration plot was constructed to assist the quantitative calculation of UA in real samples using the most proficient electrodes, Hap-Esb/ZnONPs/ACE. Fig. 7(a), presents the CV voltammograms recorded using HAp/ZnONPs/ACE at various concentrations of UA, from 0.01–1 μM (Fig. 7(b)), in a 0.1 M phosphate buffer at pH 8.0. It can be concluded from Fig. 7(c), that the current response (Ip) increases linearly with the concentration of UA in that range, with the linear regression equation of Ip (μA) = 1160.5x + 78.238 and R2 = 0.9946.
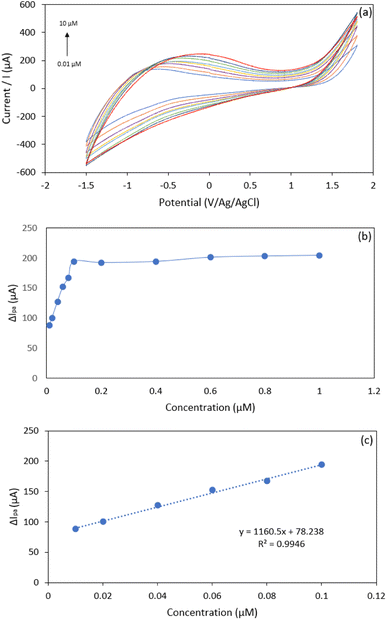 |
| Fig. 7 (a) CV voltammogram of UA at 0.01–10 μM; (b) plot of UA concentration (0.01–1 μM) vs. anodic peak current; (c) plot of UA concentration (0.01–0.1 μM) vs. anodic peak current. | |
We noticed that at concentrations of 0.01 to 1.0 μM, the peak current response tends to be flat (Fig. 7(b)); this is probably due to the saturation of the recognition site on the electrode surface.37,78 The limit of detection (LOD) was determined as “3da/b”, where ‘da’ is the standard error of the intercept, and ‘b’ is the slope of the calibration curve, while the LOQ was calculated by “10da/b”. The limit of detection (LOD) and limit of quantification (LOQ) were estimated to be 0.0086 μM and 0.028 μM, respectively. The linear current response and the LOD value obtained in this study are compared with the literature and presented in Table 2. As tabulated in Table 2, the proposed Hap-Esb based sensor is superior to those available in the literature, as suggested by the lowest LOD value. Therefore, our work creates a proof that Hap-Esb has the potential to be used as a component of a sensor.
Table 2 Comparison of the proposed uric acid sensor with literature reports
Electrode |
pH |
Linear range |
Detection limit |
Ref. |
Hap-Esb/ZnONPs/ACE |
8.0 |
0.01 to 10 μM |
0.0086 μM |
This work |
HA/GCE |
7.0 |
0.1 to 30.0 μM |
0.142 μM |
79 |
Graphene-modified carbon fiber |
7.0 |
0.194 to 49.68 μM |
0.132 μM |
22 |
Pretreated-CPE |
7.0 |
5 to 53.0 μM |
5.0 μM |
80 |
Carbon ionic liquid |
6.8 |
2 to 220 μM |
1.0 μM |
81 |
Ce-HA/GCE |
7.0 |
0.5 to 200 μM |
0.39 μM |
25 |
Poly(DPA)/SiO2@Fe3O4-CPE |
7.0 |
1.2 to 8.2 μM |
0.4 μM |
18 |
Graphite-like pyrolytic carbon film |
7.0 |
0.1 to 9.8 μM |
0.03 μM |
82 |
RGO-ZnO/GCE |
6.0 |
1.0 to 70.0 μM |
0.33 μM |
83 |
Poly(bromocresol purple)/GCE |
6.5 |
0.5 to 120 μM |
0.20 μM |
17 |
Nitrogen doped graphene |
6.0 |
0.1 to 20.0 μM |
0.045 μM |
84 |
LaPO4/CPE |
7.0 |
2.7 to 24.0 μM |
0.09 μM |
85 |
Graphene/size-selected Pt nanocomposites/GCE |
7.0 |
0.05 to 11 μM |
0.05 μM |
86 |
N-rGO |
7.0 |
1.0 to 30.0 μM |
0.20 μM |
15 |
PCN/MWCN |
4.8 |
0.2 to 4.0 μM |
0.139 μM |
87 |
n-HA/CPE |
7.0 |
0.068 to 50 μM |
0.05 μM |
23 |
Reproducibility, repeatability, and stability of the developed electrode
Reproducibility, repeatability, and stability all played important roles in sensor performance. In this investigation, four equally prepared electrodes were tested to detect UA by using 0.1 μM UA solution by CV for reproducibility evaluation. The relative standard deviation (RSD) of the peak current response of UA was found to be 0.24%. Next, we tested the developed sensor for 20 repetitive measurements to confirm the instability issue suggested by the SEM image. The CV and histogram of repetitive testing were presented in Fig. 8. Before the repeating test, the developed sensor was washed with ultrapure water using the CV for 3 cycles to remove the analyte. The CV curve of the first measurement and the 15th measurement were compared. As shown in Fig. 8(a), the recorded voltammograms at n = 1 and n = 15 were almost similar. And the calculation of RSD value for 15 repetitive measurement result in 1.5% RSD, indicating excellent repeatability performance. We recorded that at the 20th measurement, the peak current decreased (see Fig. 8(b)). This was probably due to the detachment of Hap from the electrode surface. For the aforementioned reason we suggest the prepared electrode is stable for 15 repetitive measurement.
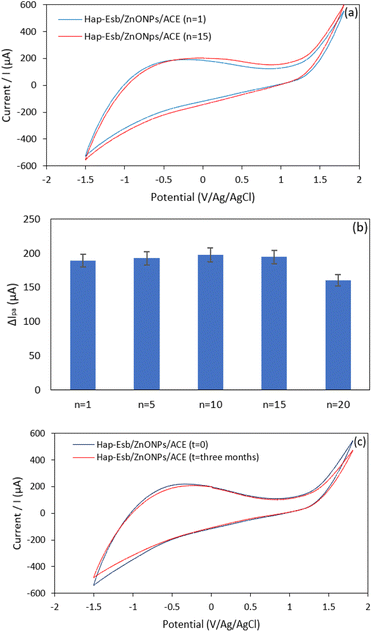 |
| Fig. 8 (a) CV voltammograms of 0.1 μM UA at n = 1 and n = 15 and (b) the histogram of oxidation peak current of 0.1 μM UA at various repetitive measurement at Hap-Esb/ZnONPs/ACE; and (c) CV of UA measured using Hap-Esb/ZnONPs/ACE at different electrode stored for 0 and 3 months. | |
Next, we also observed the stability of the developed electrode by comparing the UA peak current response measured using freshly prepared and stored electrodes. For this analysis, the electrode was stored for 3 months in a desiccator. The results of comparative measurement can be seen in Fig. 8(c). As presented, the UA peak current responses of freshly prepared (t = 0) and stored (t = 3 months) are similar, as suggested by the oxidation peak current values of 206.8 μA and 217.3 μA for t = 3 months and t = 0, respectively. Those data show that the developed sensor can be stored for 3 months.
Interference study. Possible interferences for the detection of UA at Hap-Esb/ZnONPs/ACE were evaluated by spiking UA with various interfering species. In this work, the selectivity performance of Hap-Esb/ZnONps/ACE towards UA was evaluated in the presence of glucose, ascorbic acid, and tyrosine. Those interferings are coexisting molecules present in the human biological fluids. The interfering species were first tested individually to detect any possibility of overlapping signals. As shown in Fig. 9, the CV of UA, AA, glucose, tyrosine, UA + AA, UA + glucose, UA + tyrosine, and UA + AA + glucose + tyrosine depicted the peak current response at potentials of −0.32 V, −0.49 V, −0.46 V, −0.53 V, −0.55 V, −0.44 V, −0.83 V and −0.72 V, respectively (see Fig. 9(a)). The peaks of the current responses of UA were found to be 217.3 μA, 180.9 μA, 173.8 μA, 216 μA, 193.4 μA, 204.6 μA, 208.6 μA and 248.7 μA when measured under unspiking and spiking conditions (see Fig. 9(b)). Notably, the presence of glucose and AA decreased the peak current response. In the pH optimization analysis, the pH value obviously influences the peak response of UA at Hap-Esb/ZnO/ACE (see Fig. 6). The presence of AA appears to increase the acidity and decreasing current response.87,88 Whereas the decrease of UA peak current response in the presence of glucose might be due to the competition between glucose and UA to fill the cavity of Hap-Esb.16,23,25,87,89 We also noticed that the presence of tyrosine increased the peak current response.25 Given that the difference of peak current responses are less than 10% (between 1–9.15%), our proposed sensor shows a remarkably selectivity.
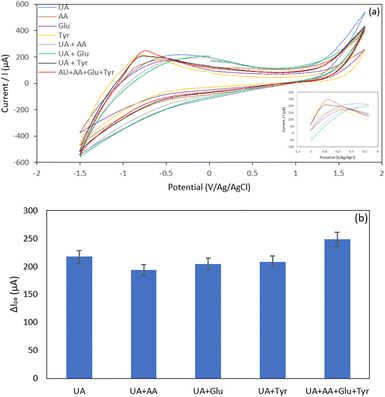 |
| Fig. 9 (a) Voltammogram of 0.2 μM interferent molecules on Hap-Esb/ZnONPs/ACE; (b) histogram of oxidation peak current of 0.2 μM interferent molecules on Hap-Esb/ZnONPs/ACE. | |
Finally, the developed sensor is applied to test UA in a real sample. In this work, we apply human urine samples. The urine samples were diluted 100 times with 0.1 M PBS pH 8. The dilution process aims to reduce as well as to eliminate the effect of metabolites present in the urine. As presented in Fig. 10, the CV voltammogram of the urine sample is similar with the UA standard solutions. The peak potential of the UA response from the urine sample was found at −0.13 V, with a peak current response of 291 μA. From the calculation results, the concentration of UA in urine is equivalent to 10.08 mg dL−1 with an RSD value of 4.4%. This results is within the range of UA concentration for healthy human urine. The results obtained were compared using the high-performance liquid chromatography (HPLC) method, with a result of 10.12 mg dL−1. The Hap-Esb/ZnONPs/ACE electrode was able to detect UA in urine samples (10.08 mg dL−1) with results that were not significantly different when using the HPLC method (10.12 mg dL−1). So we suggest that the proposed sensor promising for monitoring UA in real samples.
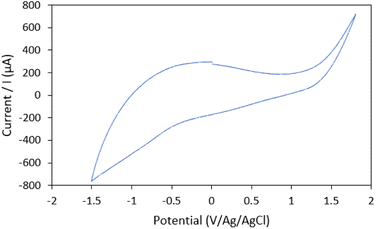 |
| Fig. 10 CV voltammogram of UA from human urine for real sample analysis at Hap/ZnONPs/ACE. | |
Conclusions
In summary, an electrochemical sensing-based Hap-Esb/ZnONPs/ACE is developed for detection of UA. A simple immobilization of Hap-Esb on ZnONPs/ACE is used to modify the electrode. Electrochemical studies show that the developed electrode exhibits excellent response for UA detection in PBS at pH 8 in the presence of interferings. The linear dynamic range of UA is 0.01–1 μM with a LOD value of 0.0086 μM at the developed electrode. It is observed that the repeatability performance of the developed electrode is verified, as suggested by a similar response after 15 repetitive CV tests. Most interestingly, the current response of UA is improved up to 13 times higher by combining the Hap-Esb with ZnONPs, suggesting a better electrocatalytic activity. Considering similar CV response of UA in real sample evaluation, the proposed Hap-Esb/ZnONPs/ACE has a promising application for monitoring UA.
Ethical statement
The authors state that for investigations involving human subjects were in compliance with relevant laws or guidelines and informed consent was obtained from all human subjects.
Conflicts of interest
There are no conflicts to declare.
Acknowledgements
The authors thank Research Center for Applied Microbiology and Advanced Characterization Laboratories BASICS at KST Samaun Samadikun, National Research and Innovation Agency Republic of Indonesia through e-Layanan Sains and Bhayangkara Jakarta Raya University. This research is partly supported by Project of Riset and Inovasi untuk Indonesia Maju (RIIM) with contract No. 82/II.7/HK/2022 under VS and PostDoctoral Program of Direktorat Manajemen Talenta BRIN with Contract No. 64/II/HK/2022 under RW.
References
- L. L. Jiang, X. Gong, M. Y. Ji, C. C. Wang, J. H. Wang and M. H. Li, Foods, 2020, 9(8), 1–24 CrossRef PubMed.
- Y. Wu, P. Deng, Y. Tian, J. Feng, J. Xiao, J. Li, J. Liu, G. Li and Q. He, J. Nanobiotechnol., 2020, 18, 112 CrossRef CAS PubMed.
- M. Kuwabara, Pulse, 2015, 3, 242–252 CrossRef PubMed.
- W. L. Nyhan, Tzu Chi Med. J., 2007, 19, 105–108 CrossRef CAS.
- A. Dehghan, M. Van Hoek, E. J. G. Sijbrands, A. Hofman and J. C. M. Witteman, Diabetes Care, 2008, 31, 361–362 CrossRef CAS PubMed.
- I. I. Hidayat, L. Hamijoyo and M. A. Moeliono, Indones. J. Rheumatol., 2013, 04, 14–19 Search PubMed.
- X. L. Jin Jun Luo, Brain Disord. Ther., 2013, 02, 109–111 Search PubMed.
- K. Inoue, T. Namiki, Y. Iwasaki, Y. Yoshimura and H. Nakazawa, J. Chromatogr. B: Anal. Technol. Biomed. Life Sci., 2003, 785, 57–63 CrossRef CAS PubMed.
- X. Dai, X. Fang, C. Zhang, R. Xu and B. Xu, J. Chromatogr. B: Anal. Technol. Biomed. Life Sci., 2007, 857, 287–295 CrossRef CAS PubMed.
- C. Yang and Z. Zhang, Talanta, 2010, 81, 477–481 CrossRef CAS PubMed.
- D. R. Norton, M. A. Plunkett, F. A. Richards and M. A. Plunkett, Anal. Chem., 1954, 26, 454–457 CrossRef CAS.
- F. Mazzara, B. Patella, G. Aiello, A. O'Riordan, C. Torino, A. Vilasi and R. Inguanta, Electrochim. Acta, 2021, 388, 138652 CrossRef CAS.
- J. Lv, C. Li, S. Feng, S.-M. Chen, Y. Ding, C. Chen, Q. Hao, T.-H. Yang and W. Lei, Ionics, 2019, 25, 4437–4445 CrossRef CAS.
- D. R. Norton, M. A. Plunkett and F. A. Richards, Anal. Chem., 1954, 26, 454–457 CrossRef CAS.
- H. Zhang and S. Liu, J. Alloys Compd., 2020, 155873 CrossRef CAS.
- D. Lakshmi, M. J. Whitcombe, F. Davis, S. Sharma and B. Prasad, Electroanalysis, 2011, 305–320 CrossRef CAS.
- Y. Wang and L. Tong, Sens. Actuators, B, 2010, 150, 43–49 CrossRef CAS.
- Y. Veera Manohara Reddy, B. Sravani, S. Agarwal, V. K. Gupta and G. Madhavi, J. Electroanal. Chem., 2018, 820, 168–175 CrossRef CAS.
- B. Govindan, R. Madhu, S.-M. Chen, V. Veeramani, A. Balamurugan, D. Mangalaraj, C. Viswanathan and P. Nagamony, J. Mater. Chem. B, 2015, 3(7), 1360–1370 RSC.
- R. Jirakunakorn, S. Khumngern, J. Choosang, P. Thavarungkul, P. Kanatharana and A. Numnuam, Microchem. J., 2020, 154, 104624 CrossRef CAS.
- H. Zhu, L. Li, W. Zhou, Z. Shao and X. Chen, J. Mater. Chem. B, 2016, 4, 7333–7349 RSC.
- J. Du, R. Yue, Z. Yao, F. Jiang, Y. Du, P. Yang and C. Wang, Colloids Surf., A, 2013, 419, 94–99 CrossRef CAS.
- E. Iyyappan, S. J. Samuel Justin, P. Wilson and A. Palaniappan, ACS Appl. Nano Mater., 2020, 3, 7761–7773 CrossRef CAS.
- P. Kanchana and C. Sekar, Mater. Sci. Eng., C, 2014, 42, 601–607 CrossRef CAS PubMed.
- P. Kanchana, M. Navaneethan and C. Sekar, Mater. Sci. Eng., B, 2017, 226, 132–140 CrossRef CAS.
- P. S. L. Pandharipande, Int. J. Adv. Inf. Sci. Technol., 2016, 52, 36–47 Search PubMed.
- E. M. Rivera, M. Araiza, W. Brostow, V. M. Castaño, J. R. Díaz-Estrada, R. Hernández and J. R. Rodríguez, Mater. Lett., 1999, 41, 128–134 CrossRef CAS.
- K. Lin and J. Chang, Structure and Properties of Hydroxyapatite for Biomedical Applications, Elsevier Ltd., 2015, vol. 4214 Search PubMed.
- A. S. M. Bashir and Y. Manusamy, J. Eng. Res. Technol., 2016, 2, 2015 Search PubMed.
- M. T. Hincke, Y. Nys, J. Gautron, K. Mann, A. B. Rodriguez-Navarro and M. D. McKee, Front. Biosci., 2012, 17, 1266–1280 CrossRef CAS PubMed.
- C. X. Wang, M. Wang and X. Zhou, Biomaterials, 2003, 24, 3069–3077 CrossRef CAS PubMed.
- Z. Zyman, J. Weng, X. Liu, X. Li and X. Zhang, Biomaterials, 1994, 15, 151–155 CrossRef CAS PubMed.
- G. Bharath, R. Madhu, S.-M. Chen, V. Veeramani, A. Balamurugan, D. Mangalaraj, C. Viswanathan and N. Ponpandian, J. Mater. Chem. B, 2015, 3, 1360–1370 RSC.
- J. J. K. Ngouoko, K. Y. Tajeu, R. C. T. Temgoua, G. Doungmo, I. Doench, A. K. Tamo, T. Kamgaing, A. Osorio-Madrazo and I. K. Tonle, Materials, 2022, 15(12), 1–17 CrossRef PubMed.
- A. Khalil, A. Almajid and M. Soliman, Mater. Sci. Appl., 2011, 02, 105–110 Search PubMed.
- G. Bharath, A. Naldoni, K. H. Ramsait, A. Abdel-Wahab, R. Madhu, E. Alsharaeh and N. Ponpandian, J. Mater. Chem. A, 2016, 4, 6385–6394 RSC.
- T. Tite, A. C. Popa, L. M. Balescu, I. M. Bogdan, I. Pasuk, J. M. F. Ferreira and G. E. Stan, Materials, 2018, 11, 1–62 CrossRef PubMed.
- R. Verma, S. Pathak, A. K. Srivastava, S. Prawer and S. Tomljenovic-Hanic, J. Alloys Compd., 2021, 876, 160175 CrossRef CAS.
- T. Sen, S. Mishra, S. Sonawane and N. Shimpi, Polym. Eng. Sci., 2018, 58(8), 1438–1445 CrossRef CAS.
- V. S. Bhati, M. Hojamberdiev and M. Kumar, Energy Rep., 2020, 6, 46–62 CrossRef.
- K. Shi and K.-K. Shiu, Electroanalysis, 2001, 13, 1319–1325 CrossRef CAS.
- B. Uslu, Open Chem. Biomed. Methods J., 2011, 3, 56–73 CrossRef.
- M. Ghaedi, S. Y. S. Jaberi, S. Hajati, M. Montazerozohori, M. Zarr, A. Asfaram, L. K. Kumawat and V. K. Gupta, Electroanalysis, 2015, 27, 1516–1522 CrossRef CAS.
- M. Ganjali, Int. J. Electrochem. Sci., 2017, 12, 3231–3240 CrossRef CAS PubMed.
- T. Kokab, A. Shah, M. A. Khan, M. Arshad, J. Nisar, M. N. Ashiq and M. A. Zia, ACS Appl. Nano Mater., 2021, 4, 4699–4712 CrossRef CAS.
- D. Wingett, P. Louka, C. B. Anders, J. Zhang and A. Punnoose, Nanotechnol., Sci. Appl., 2016, 9, 29–45 CrossRef CAS PubMed.
- M. L. M. Napi, S. M. Sultan, R. Ismail, K. W. How and M. K. Ahmad, Materials, 2019, 12(18), 1–34 CrossRef PubMed.
- Z. Wang, H. Li, F. Tang, J. Ma and X. Zhou, Nanoscale Res. Lett., 2018, 13, 202 CrossRef PubMed.
- M. S. Rahmanpour and M. A. Khalilzadeh, Sensors, 2016, 8, 922–930 CAS.
- Y. Zhao, Z. Wang, R. Yuan, Y. Lin, J. Yan, J. Zhang, Z. Lu, D. Luo, J. Pietrasik, M. R. Bockstaller and K. Matyjaszewski, Polymer, 2018, 137, 370–377 CrossRef CAS.
- S. Chaudhary, A. Umar, K. K. Bhasin and S. Baskoutas, Materials, 2018, 11, 1–38 CrossRef PubMed.
- P. R. Dev, C. P. Anand, D. S. Michael and P. Wilson, Mater. Adv., 2022, 7773–7809 RSC.
- T. Mutuk and M. Gürbüz, J. Compos. Mater., 2021, 55, 3087–3097 CrossRef CAS.
- M. Magni, D. Sironi, M. Ferri, S. Trasatti, S. Campisi, A. Gervasini, M. Papacchini and P. Cristiani, ChemElectroChem, 2023, 202201017, 1–11 Search PubMed.
- S. Joseph, D. M. Kempaiah, M. R. Benzigar, H. Ilbeygi, G. Singh, S. N. Talapaneni, D. H. Park and A. Vinu, Microporous Mesoporous Mater., 2019, 280, 337–346 CrossRef CAS.
- I. Švancara and K. Kalcher, Adv. Electrochem. Sci. Eng., 2016, 16, 379–423 Search PubMed.
- E. Pérez-Mayoral, I. Matos, M. Bernardo and I. M. Fonseca, Catalysts, 2019, 9(2), 1–35 CrossRef.
- H. Setiyanto, P. Faradilla, R. Manurung and V. Saraswaty, RSC Adv., 2022, 12, 743–752 RSC.
- D. Cree and P. Pliya, J. Build. Eng., 2019, 26, 100852 CrossRef.
- F. Murakami, P. Rodrigues, C. Campos and M. Silva, Cienc. Tecnol. Aliment., 2007, 27(3), 658–662 CrossRef CAS.
- M. Marraha, J. C. Heughebaert and M. Heughebaert, Phosphorus, Sulfur Silicon Relat. Elem., 1993, 79, 281–291 CrossRef CAS.
- M. Tagaya, T. Ikoma, N. Hanagata, D. Chakarov, B. Kasemo and J. Tanaka, Sci. Technol. Adv. Mater., 2010, 11, 45002 CrossRef PubMed.
- S. Fatimah, R. Ragadhita, D. F. Al Husaeni and A. B. D. Nandiyanto, ASEAN J. Sci. Eng., 2021, 2, 65–76 CrossRef.
- F. Hilbrig and R. Freitag, in Biopharmaceutical Production Technology, Volume 1 & Volume 2, 2012, vol. 1, pp. 283–331 Search PubMed.
- A. Afshar, M. Ghorbani, N. Ehsani, M. R. Saeri and C. C. Sorrell, Mater. Des., 2003, 24, 197–202 CrossRef CAS.
- J. J. K. Ngouoko, K. Y. Tajeu, R. C. T. Temgoua, G. Doungmo, I. Doench, A. K. Tamo, T. Kamgaing, A. Osorio-Madrazo and I. K. Tonle, Materials, 2022, 15(12), 1–17 CrossRef PubMed.
- S. Das Lala, P. Deb, E. Barua, A. B. Deoghare and S. Chatterjee, Mater. Today: Proc., 2019, 15, 323–327 CAS.
- T. V. Gopal, T. M. Reddy, P. Shaikshavali and G. Venkataprasad, Surf. Interfaces, 2021, 24, 101145 CrossRef CAS.
- M. Manoj, R. Subbiah, D. Mangalaraj, N. Ponpandian, C. Viswanathan and K. Park, Nanobiomedicine, 2015, 2, 1–11 CrossRef PubMed.
- M. Tourbin, F. Brouillet, B. Galey, N. Rouquet, P. Gras, N. Abi Chebel, D. Grossin and C. Frances, Powder Technol., 2020, 360, 977–988 CrossRef CAS.
- F. Wu, D. D. W. Lin, J. H. Chang, C. Fischbach, L. A. Estroff and D. Gourdon, Cryst. Growth Des., 2015, 15, 2452–2460 CrossRef CAS PubMed.
- C. Wang and S. H. Chen, Acta Mech. Sin. Xuebao, 2012, 28, 711–719 CrossRef CAS.
- L. Khalafi, Cyclic Voltammetry, 2017 Search PubMed.
- N. Thakur, D. Mandal and T. C. Nagaiah, J. Mater. Chem. B, 2021, 9, 8399–8405 RSC.
- T. Kawasaki, J. Chromatogr. A, 1991, 544, 147–184 CrossRef CAS.
- G. Viscusi, G. Barra and G. Gorrasi, Cellulose, 2020, 27, 8653–8665 CrossRef CAS.
- G. Bharath, A. J. Kumar, K. Karthick, D. Mangalaraj and C. Viswanathan, RSC Adv., 2014, 4, 37446–37457 RSC.
- Q. Yan, N. Zhi, L. Yang, G. Xu, Q. Feng, Q. Zhang and S. Sun, Sci. Rep., 2020, 1–10 Search PubMed.
- P. Kanchana and C. Sekar, Mater. Sci. Eng., C, 2014, 42C, 601–607 CrossRef PubMed.
- X. Cai, K. Kalcher, C. Neuhold and B. Ogorevc, Talanta, 1994, 41, 407–413 CrossRef CAS PubMed.
- A. Safavi, N. Maleki, O. Moradlou and F. Tajabadi, Anal. Biochem., 2006, 359, 224–229 CrossRef CAS PubMed.
- M. Hadi and A. Rouhollahi, Anal. Chim. Acta, 2012, 721, 55–60 CrossRef CAS PubMed.
- X. Zhang, Y. Zhang and L. Ma, Sens. Actuators, B, 2016, 227, 488–496 CrossRef CAS.
- Z. Sheng, X. Zheng, J. Xu, W. Bao, F. Wang and X. Xia, Biosens. Bioelectron., 2012, 34, 125–131 CrossRef CAS PubMed.
- Y. Zhou, H. Zhang, H. Xie, B. Chen, L. Zhang, X. Zheng and P. Jia, Electrochim. Acta, 2012, 75, 360–365 CrossRef CAS.
- C. Sun, H. Lee, J. Yang and C. Wu, Biosens. Bioelectron., 2011, 26, 3450–3455 CrossRef CAS PubMed.
- J. Lv, C. Li, S. Feng, S. M. Chen, Y. Ding, C. Chen, Q. Hao, T. H. Yang and W. Lei, Ionics, 2019, 25, 4437–4445 CrossRef CAS.
- N. Tukimin, J. Abdullah and Y. Sulaiman, Sensors, 2017, 17(7), 1–12 CrossRef PubMed.
- M. Khasanah, H. Darmokoesoemo, N. Widayanti, Y. Kadmi, H. Elmsellem and H. S. Kusuma, Results Phys., 2017, 7, 1833–1844 CrossRef.
|
This journal is © The Royal Society of Chemistry 2023 |