DOI:
10.1039/D3RA01186K
(Paper)
RSC Adv., 2023,
13, 12065-12071
Highly enhanced electrocatalytic OER activity of water-coordinated copper complexes: effect of lattice water and bridging ligand†
Received
22nd February 2023
, Accepted 4th April 2023
First published on 18th April 2023
Abstract
The use of metal–organic compounds as electrocatalysts for water splitting reactions has gained increased attention; however, a fundamental understanding of the structural requirement for effective catalytic activity is still limited. Herein, we synthesized water-coordinated mono and bimetallic copper complexes (CuPz-H2O·H2O, CuPz-H2O, CuBipy-H2O·H2O, and CuMorph-H2O) with varied intermetallic spacing (pyrazine/4,4′-bipyridine) and explored the structure-dependent oxygen evolution reaction (OER) activity in alkaline medium. Single crystal structural studies revealed water-coordinated monometallic complexes (CuMorph-H2O) and bimetallic complexes (CuPz-H2O·H2O, CuPz-H2O, CuBipy-H2O·H2O). Further, CuPz-H2O·H2O and CuBipy-H2O·H2O contained lattice water along with coordinated water. Interestingly, the bimetallic copper complex with lattice water and shorter interspacing between the metal centres (CuPz-H2O·H2O) showed strong OER activity and required an overpotential of 228 mV to produce a benchmark current density of 10 mA cm−2. Bimetallic copper complex (CuPz-H2O) without lattice water but the same intermetallic spacing and bimetallic complex with increased interspacing but with lattice water (CuBipy-H2O·H2O) exhibited relatively lower OER activity. CuPz-H2O and CuBipy-H2O·H2O required an overpotential of 236 and 256 mA cm−2, respectively. Monometallic CuMorph-H2O showed the lowest OER activity (overpotential 271 mV) compared to bimetallic complexes. The low Tafel slope and charge transfer resistance of CuPz-H2O·H2O facilitated faster charge transfer kinetics at the electrode surface and supported the enhanced OER activity. The chronoamperometric studies indicated good stability of the catalyst. Overall, the present structure-electrocatalytic activity studies of copper complexes might provide structural insight for designing new efficient electrocatalysts based on metal coordination compounds.
Introduction
Electrochemical energy conversion and energy storage technologies, such as fuel cells, metal–air batteries, and electrochemical water splitting, are receiving great attention due to the increasing concern about environmental pollution and global climate change caused by the excess burning of fossil fuels.1,2 The electrochemical water splitting into hydrogen and oxygen offers a promising method to produce efficient, carbon-free, sustainable energy, and storage.3–5 In particular, the electrochemical oxygen evolution reaction (OER) is an important process in all these energy conversion and storage devices.6–8 Nevertheless, the multi-electron transfer process of OER is a thermodynamically uphill task that requires stable and efficient electrocatalysts for reducing the overpotential (η).9,10 The precious metal-based RuO2 and IrO2 show excellent activity; however, their scarcity and high cost restrict their extensive practical usage.11,12 Hence, great efforts are being made in recent years to develop earth-abundant, efficient, and low-cost OER electrocatalysts. Transition metals, especially earth-abundant 3d elements, have received great interest in developing efficient electrocatalysts due to their ability to exhibit multiple oxidation states.13–17 Transition metal-based nanostructured materials, including oxides, oxyhydroxides, sulphides, and phosphates, homogeneous and heterogeneous metal complexes, and metal–organic frameworks (MOFs) have been actively explored for developing cost-effective efficient electrocatalysts.18–28
Metal–organic frameworks (MOFs), which are generated by the coordination of metal ions and organic ligands, have emerged as an important class of porous materials in catalysis/electrocatalysis because of their tunable porosity, large surface area, and tailorable structures and functionality.29–38 However, the bulky organic linkers reduce the electric conductivity of MOFs as well as hinder the accessibility to the active metal sites that often contribute to the low electrocatalytic activity. To improve the conductivity, composites of MOFs are made by combining them with conducting graphene oxide or acetylene black.39 The use of π-conjugated dithiolene ligands showed improvement in coordination polymer conductivity and electrocatalytic activity.40–44 The coordination environment and functionality of ligands can strongly influence the activity of MOFs. For instance, two Co-MOFs with similar coordination modes showed drastically different electrocatalytic activity due to variations in water coordination.45 Water-coordinated Cu-peptoid complex exhibited enhanced water oxidation in the borate buffer medium.46 Recently, we have observed a strong enhancement of copper coordination in the electrocatalytic activity polymers by coordinated water molecules and hydrophilic functionalities.47–49 The interlinking of metal active centres in coordination polymer and increasing conjugation strongly enhanced the electrocatalytic activity compared to the complex with a similar coordination environment.47–51 However, still it is a challenge to design the structure of new coordination complexes/polymers/MOFs with enhanced electrocatalytic activity and required more systematic studies with a series of complexes/polymers.
In continuation of our previous works on copper coordination complex/polymer designing and fabricating electrocatalysts,42–44 herein, we have prepared a series of water-coordinated copper complexes (Scheme 1) with different spacers and investigated the electrocatalytic OER activity in an alkaline medium. Single crystal structural studies confirmed water-coordinated mono and bimetallic copper complexes, the inclusion of lattice water with varied interspacing. Interestingly, water-coordinated copper complexes along with lattice water (CuPz-H2O·H2O) showed stronger OER activity (required 228 mV to produce 10 mA cm−2 current density) compared to only water-coordinated complex (CuPz-H2O·H2O required 236 mV to produce 10 mA cm−2 current density). Further, the bimetallic complex connected with a shorter spacer showed higher activity compared to the longer spacer (228 vs. 256 mV to produce 10 mA cm−2 current density). The bimetallic copper complexes showed stronger activity compared to the mononuclear complex with water coordination.
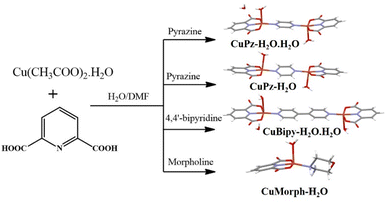 |
| Scheme 1 Synthesis of water-coordinated mono and bimetallic copper complexes. | |
Experimental
Copper nitrate trihydrate (Cu(NO3)2·3H2O), pyridine dicarboxylic acid, pyrazine, 4,4′-bipyridine, and morpholine were purchased from Sigma-Aldrich India and used as received. Nafion (product number: 274704), 5 wt% in lower aliphatic alcohols and water containing 15–20% water was obtained from Sigma-Aldrich. Carbon cloth (CC) was purchased from the electrode store in Tamil Nadu, India.
Synthesis of CuPz-H2O·H2O, CuPz-H2O, CuBipy-H2O·H2O, and CuMorph-H2O49,52
CuPz-H2O·H2O, CuBipy-H2O·H2O and CuMorph-H2O. Cu(NO3)2·3H2O (0.3 mM, 0.074 g), PDC (0.4 mM, 0.068 g) and pyrazine (0.45 mM, 0.038 g) were dissolved in 15 ml water and sonicated for 20 min to form a clear solution. The final mixture was taken in a Teflon-lined stainless-steel autoclave and was heated in an oven at 120 °C for 36 hours, then cooled to room temperature. The blue crystals of CuPz-H2O·H2O (yield = 70%, 0.077 g) were collected (Fig. S1†), washed with DMF, and dried under a vacuum. In the same synthetic methods, 4,4′-bipyridine (0.067 g) and morpholine (37 μl) were used instead of pyrazine that produced CuBipy-H2O·H2O (yield = 72%, 0.086 g) and CuMorph-H2O (yield = 76%, 0.091 g), respectively.
CuPz-H2O53. In a similar experiment to CuPz-H2O·H2O, DMF was used in place of water as a solvent to form CuPz-H2O crystals (yield = 72%, 0.072 g, Fig. S1†).
Characterization
FT-IR was measured on a PerkinElmer Spectrum 3 FT-IR spectrometer (L1280127). The phase and the crystallographic structure were identified using X-ray diffraction (XRD, Brucker, Cu-Kα: λ = 0.1540598 nm) at a scanning rate of 0.07° s−1 with 2θ ranging from 10° to 80°. Single crystals were coated with paratone-N oil and the diffraction data were measured using the synchrotron radiation (λ = 0.62998 Å) on an ADSC Quantum-210 detector at 2D SMC with a silicon (111) double crystal monochromator (DCM) at the Pohang Accelerator Laboratory, Korea. The crystallographic data from this paper is with the Cambridge crystallographic data centre (CCDC) no. 2240777. Thermogravimetric analysis (TGA) was performed using PerkinElmer TGA-8000 at N2 atmosphere. X-ray photoelectron spectroscopic (XPS) analysis was performed using a K-Alpha instrument (XPS KAlpha surface analysis, Thermo Fisher Scientific, UK).
Electrochemical measurements
The electrochemical measurements were performed using a three-electrode configuration (electrochemical work station - CHI660E, Austin, Texas, USA) with modified carbon cloth (CC) as the working electrode, graphite rod as a counter electrode, and Hg/HgO as a reference electrode in 1.0 M KOH electrolyte solution. Carbon cloth was pre-treated with HNO3 and ethanol for 30 minutes to increase the hydrophilic nature. 4 mg of the catalyst was dispersed in 125 μl water, 125 μl ethanol, and 50 μl Nafion, and sonicated for 20 minutes to form a homogenous dispersion. 4 μl dispersed solution was drop cast onto the carbon cloth. Using the same electrodes, linear sweep voltammetry (LSV) was performed at a scan rate of 1 mV s−1. For the stability studies, 1000 cycles of CV cycling were performed at 100 mV s−1. All the potentials were converted to the reversible hydrogen electrode (RHE) according to the following equation:
. Electrochemical impedance spectroscopy (EIS) was performed (270 mV) with frequencies of 0.1 to 1
000
000 Hz and 10 mV amplitude in 1.0 M KOH solution. The non-faradaic region was investigated using cyclic voltammetry at various scan rates (10–100 mV s−1) to quantify the electrochemical surface area.
Results and discussion
Cu2+ was chosen to synthesise metal coordination compounds because of its versatile coordination geometry and the possibility of obtaining the water-coordinated complex/polymer.44–46 Similarly, pyridine dicarboxylic acid (PDA) ligands can exhibit varied coordination modes depending on the experimental condition.44 The mixing of Cu2+, PDA, and pyrazine (Pz)/bipyridine (Bipy)/morpholine (Morph) at a 1
:
1
:
1 ratio in water/DMF produced bimetallic copper complexes with different spacers (Pz/Bipy) and a mononuclear copper complex. The solid-state structural analysis confirmed the water coordination with the Cu metal centre in all four complexes (Fig. 1). Apart from water coordination, an additional water molecule was also present in the lattice of CuPz-H2O·H2O and CuBipy-H2O·H2O. Only coordinated water molecules are present in CuPz-H2O and CuMorph-H2O. It is noted that the single crystal structure CuPz-H2O·H2O, CuPz-H2O, and CuBipy-H2O·H2O are perfectly matched with the reported copper complex.49,52,53 The copper ions displayed distorted square-pyramidal geometry in all complexes. In CuPz-H2O·H2O, pyrazine and PDA aromatic rings displayed coplanar conformation while pyrazine adopted slightly twisted conformation in CuPz-H2O (Fig. 1). Similarly, the bipyridine and PDA rings displayed a coplanar conformation in CuBipy-H2O·H2O (Fig. 1). Thus, the inclusion of lattice water forced a coplanar conformation between the aromatic amine and PDA ligand.
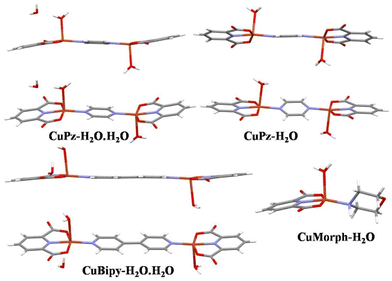 |
| Fig. 1 Molecular structure and conformation of copper complexes in the crystal lattice of CuPz-H2O·H2O, CuPz-H2O, CuBipy-H2O·H2O, and CuMorph-H2O. C (grey), H (white), N (blue), O (red), and Cu (brown). | |
The lattice water solvent involved in intermolecular H-bonding with coordinated water and the carbonyl oxygen of another molecule that connected the molecules in the crystal lattice of CuPz-H2O·H2O (Fig. 2). The intermolecular H-bonding was between the coordinated water and coordinated carbonyl oxygen interconnected the molecules in CuPz-H2O and CuMorph-H2O (Fig. 2). The molecules in the crystal lattice of CuBipy-H2O·H2O are interconnected by H-bonding of lattice water as well as coordinated water with another molecule (Fig. 2). The intermolecular H-bonding of interactions of lattice water and coordinated water in the crystal lattice of CuPz-H2O·H2O and CuBipy-H2O·H2O produced a 3D network structure (Fig. S2 and S4†). However, the coordinated water molecule H-bonding interaction in the crystal lattice of CuPz-H2O and CuMorph-H2O produced a 2D network structure (Fig. 2, S3, and S5†). PXRD studies were performed to confirm the phase purity of the samples. The perfect matching between the simulated and experimental PXRD pattern confirmed the phase purity of the samples (Fig. 3). The presence of the coordinated and non-coordinated water molecules in the complexes was further confirmed by thermogravimetric analysis (TGA, Fig. 4). CuPz-H2O·H2O and CuBipy-H2O·H2O showed weight loss from 50 °C onwards due to the removal of lattice water molecules. However, the coordinated water molecule in CuPz-H2O and CuMorph-H2O was removed only after 120 °C. All four complexes decomposed around 300 °C. FTIR also further confirmed the water coordination by exhibiting a broad peak around 3500 cm−1 (Fig. S6†).
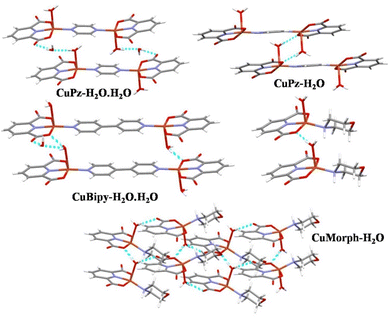 |
| Fig. 2 Intramolecular H-bonding interactions in the crystal lattice CuPz-H2O·H2O, CuPz-H2O, CuBipy-H2O·H2O, and CuMorph-H2O. C (grey), H (white), N (blue), O (red), and Cu (brown). The dotted lines indicate the hydrogen bonding interactions. H-bond distances ranged between 2.733 and 2.980 Å. | |
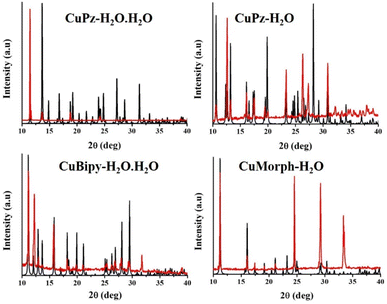 |
| Fig. 3 Comparison of simulated (black line) and experimental (red line) PXRD pattern of copper complexes. | |
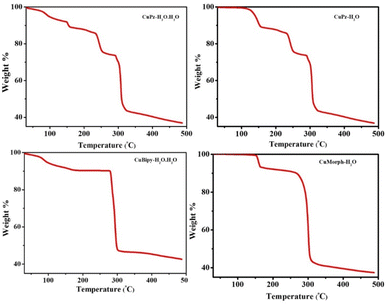 |
| Fig. 4 TG analysis of the copper complexes. | |
The electrocatalytic OER studies for copper complex catalysts coated on carbon cloth (CC) were performed using linear sweep voltammetry (LSV) in a standard three-electrode cell in 1.0 M alkaline medium (pH = 14.0) at a scan rate of 1 mV s−1. In the same condition, electrochemical measurements were also performed for the bare CC electrode and commercial RuO2 for comparison. As expected, the bare CC electrode did not show any significant OER activity (Fig. 5a). However, the copper complexes modified CC electrode showed highly enhanced but varied OER activity depending upon the complex. CuPz-H2O·H2O exhibited a comparatively higher electrocatalytic activity among the copper complexes (Fig. 5a and S7†). CuPz-H2O·H2O required an overpotential of 228 mV to produce a benchmark current density of 10 mA cm−2. CuPz-H2O, CuBipy-H2O·H2O, and CuMorph-H2O required the overpotential of 236, 256, and 271 mV for achieving 10 mA cm−2 current density. The commercial RuO2 fabricated CC electrode required 364 mV for producing the current density of 10 mA cm−2. CuPz-H2O·H2O also exhibited the highest current density (175.2 mA cm−2) compared to other copper complexes at the applied potential. Tafel slope analysis showed the lowest value for CuPz-H2O·H2O (55.8 mV dec−1) compared to bimetallic CuPz-H2O (57.5 mV dec−1) and CuBipy-H2O·H2O (63.2 mV dec−1) as well as monometallic CuMorph-H2O (71.8 mV dec−1, Fig. 5b). RuO2 showed a Tafel slope of 67 mV dec−1. The low Tafel value of CuPz-H2O·H2O suggested faster kinetics compared to other copper complexes. The electrochemical impedance (EIS) measurement also showed relatively low charge transfer resistance for the CuPz-H2O·H2O complex compared to other complexes as well as commercial RuO2 (Fig. 5c). CuPz-H2O·H2O showed charge transfer resistance of 5.28 Ω whereas CuPz-H2O, CuBipy-H2O·H2O, and CuMorph-H2O exhibited charge transfer resistances of 6.03, 17.41, and 19.87 Ω, respectively. The low charge transfer resistance of CuPz-H2O·H2O further supported the stronger OER catalytic activity compared to other complexes. The comparison of LSV curves of CuPz-H2O·H2O electrode between the 1st and 1000th cycle showed negligible variation and indicated the good stability of the catalysts (Fig. 5d). The current–time amperometric test was performed for CuPz-H2O·H2O at the overpotential of 228 mV (1.0 M KOH, a current density of 10 mA cm−2 and room temperature) showed only slight decrease of current density in 36 h and suggested good stability of the catalyst (Fig. 5e). The current–time amperometric studies at higher potential and current density (380 mV and 30 mA cm−2) also displayed good stability over 30 h (Fig. S8†). The current–time amperometric test was also performed by step-wise increasing the potential and observing the current density using the CuPz-H2O·H2O catalyst (Fig. 5f). At the low applied potential, the CuPz-H2O·H2O catalyst showed constant current density; however, it showed a decrease of current density at a higher applied potential. Further, XPS analysis of CuPz-H2O·H2O suggested that copper is present in a +2 oxidation state with a similar electronic environment as that in the as-prepared formed, after pasting onto the carbon cloth and after electrocatalysis (Fig. S9†). High-resolution XPS spectra of Cu (as-prepared and after pasting onto carbon cloth) showed peaks at 934.65 and 954.48 eV, corresponding to Cu 2p3/2 and Cu 2p1/2, respectively. After electrochemical studies, Cu 2p3/2 and Cu 2p1/2 peaks were observed at 933.74 and 953.67 eV, respectively.
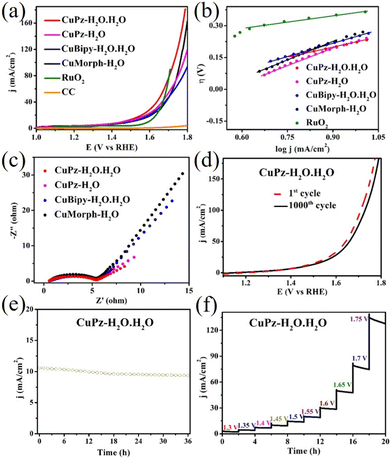 |
| Fig. 5 (a) OER polarization curves (scan rate 1 mV s−1), (b) Tafel plots of copper complexes, (c) electrochemical impedance spectra of copper complexes, and (d–f) stability studies of CuPz-H2O·H2O. (d) OER curve of CuPz-H2O·H2O at the 1st cycle (before OER) and after 1000th cycles showed the same activity, (e) current–time amperometric test and (f) multi-potential step studies of CuPz-H2O·H2O. | |
The electrochemical double layer capacitance (Cdl) of the catalyst provided insights into the OER activity of the catalysts. The electrochemical double layer capacitance for CuPz-H2O·H2O, CuPz-H2O, CuBipy-H2O·H2O, and CuMorph-H2O was calculated based on linear proportionality. The non-faradaic region of CuPz-H2O·H2O, CuPz-H2O, CuBipy-H2O·H2O, and CuMorph-H2O are shown in Fig. S10.† The slopes obtained from the linear relationship of the current density differences (Janode − Jcathode) vs. the scan rate indicated high active sites in CuPz-H2O·H2O compared to those in the other complexes (Fig. 6). CuPz-H2O·H2O exhibited slightly higher Cdl (3.3 mF cm−2) compared to CuPz-H2O (Cdl = 3.1 mF cm−2), CuBipy-H2O·H2O (Cdl = 2.8 mF cm−2), and CuMorph-H2O (Cdl = 2.4 mF cm−2).
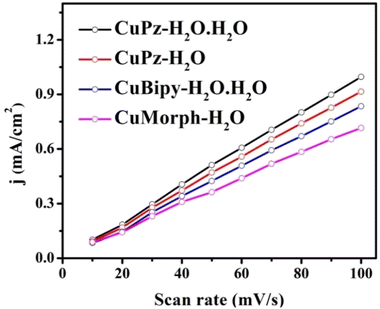 |
| Fig. 6 Double layer capacitance and capacitive currents as a function of the scan rate. | |
The comparison of the copper complexes structure with OER activity showed a very interesting trend in the OER activity. Monometallic complex, CuMorph-H2O·H2O exhibited the lowest activity compared to bimetallic copper complexes and indicated that interconnected metal active centres could be beneficial for enhancing activity.44–46 Among the bimetallic copper complexes, CuPz-H2O·H2O and CuPz-H2O displayed similar coordination except for the presence of lattice water molecules in the former. But the higher activity of CuPz-H2O·H2O suggested that the presence of lattice water along with coordinated water might help improve the activity. Both CuPz-H2O·H2O and CuBipy-H2O·H2O showed similar coordination geometry, water coordination, and lattice water. However, the metal centres in CuPz-H2O·H2O are connected using a shorter linker, pyrazine whereas metal centres are connected by a longer spacer, 4,4′-bipyridine in CuBipy-H2O·H2O. Interestingly, CuPz-H2O·H2O showed stronger OER activity compared to CuBipy-H2O·H2O. Thus, the interconnected active metal centres separated by shorter distances might have better electronic coupling and improved electrocatalytic activity.
Conclusion
In summary, water-coordinated mono and bimetallic copper coordination complexes with similar coordination environments were synthesized and compared for the structure-dependent OER activity in an alkaline medium. CuMorph-H2O formed as monometallic water coordinated complex whereas CuPz-H2O·H2O, CuPz-H2O, and CuBipy-H2O·H2O formed bimetallic water coordinated complex with different interspacing between the metal centre. OER studies of CuMorph-H2O showed the lowest activity that required 271 mV to produce 10 mA cm−2 current density. Interestingly, the bimetallic complexes showed enhanced OER activity. The presence of lattice water with short interspacing between the metal centre in CuPz-H2O·H2O produced strong OER activity. It required an overpotential of 226 mV to achieve the 10 mA cm−2 current density. The complex without the lattice water (CuPz-H2O) and with lattice water but increased interspacing (CuBipy-H2O·H2O) exhibited relatively low OER activity. CuPz-H2O and CuBipy-H2O·H2O required an overpotential of 236 and 256 mV to produce 10 mA cm−2 current density, respectively. Tafel slope analysis and impedance studies suggested faster reaction kinetics with low charge transfer resistance in CuPz-H2O·H2O and supported the enhanced OER activity. CuPz-H2O·H2O also exhibited good stability over 36 h. Thus, the present studies indicated that interconnected metal active centres with short intermetallic distances in metal coordination compounds might facilitate better electronic coupling and promote the electrocatalytic activity.
Conflicts of interest
There are no conflicts to declare.
Acknowledgements
Financial support from the Science and Engineering Research Board (SERB), Core Research grant (CRG) (CRG/2020/003978) New Delhi, India is acknowledged with gratitude. The Deanship of scientific research at King Khalid University is greatly appreciated for funding (R.G.P.1/274/43). The X-ray crystallography was supported by Basic Science Research Program through the National Research Foundation of Korea (NRF) funded by the Ministry of Education, Science, and Technology (NRF-2021R1A2C1003080).
References
- S. Chu, Y. Cui and N. Liu, Nat. Mater., 2017, 16, 16–22 CrossRef PubMed
. - E. Hu, X.-Y. Yu, F. Chen, Y. Wu and X. W. Lou, Adv. Energy Mater., 2018, 9, 1702476 CrossRef
. - Z. Zhou, X. Li, Q. Li, Y. Zhao and H. Pang, Mater. Today Chem., 2019, 11, 169–196 CrossRef CAS
. - H. Sun, Z. Yan, F. Liu, W. Xu, F. Cheng and J. Chen, Adv. Mater., 2020, 32, 1806326 CrossRef CAS PubMed
. - B. Zhang, Y. Zheng, T. Ma, C. Yang, Y. Peng, Z. Zhou, M. Zhou, S. Li, Y. Wang and C. Cheng, Adv. Mater., 2021, 33, 2006042 CrossRef CAS PubMed
. - Y. Jiao, Y. Zheng, M. Jaroniec and S. Z. Qiao, Chem. Soc. Rev., 2015, 44, 2060 RSC
. - R. P. Putra, H. Horino and I. I. Rzeznicka, Catalysts, 2020, 10, 233 CrossRef CAS
. - P. P. Wang, J. An, Z. Ye, W. Cai and X. Zheng, Front. Chem., 2022, 10, 913874 CrossRef CAS PubMed
. - C. Tang, N. Cheng, Z. Pu, W. Xing and X. Sun, Angew. Chem., Int. Ed., 2015, 54, 9351–9487 CrossRef CAS PubMed
. - C. C. L. McCrory, S. Jung, I. M. Ferrer, S. Chatman, J. C. Peters and T. F. Jaramillo, J. Am. Chem. Soc., 2015, 137, 4347–4357 CrossRef CAS PubMed
. - H. Guo, Z. Fang, H. Li, D. Fernandez, G. Henkelman, S. M. Humphrey and G. Yu, ACS Nano, 2019, 13, 13225–13234 CrossRef CAS PubMed
. - Y. Xing, J. Ku, W. Fu, L. Wang and H. Chen, Chem. Eng. J., 2020, 395, 125149 CrossRef CAS
. - F. Song, L. Bai, A. Moysiadou, S. Lee, C. Hu, L. Liardet and X. Hu, J. Am. Chem. Soc., 2018, 140, 7748–7759 CrossRef CAS PubMed
. - K. Wang, X. Wang, Z. Li, B. Yang, M. Ling, X. Gao, J. Lu, Q. Shi, L. Lei, G. Wu and Y. Hou, Nano Energy, 2020, 77, 105162 CrossRef CAS
. - K. Zhang and R. Zou, Small, 2021, 17, 2100129 CrossRef CAS PubMed
. - W. Shao, M. Xiao, C. Yang, M. Cheng, S. Cao, C. He, M. Zhou, T. Ma, C. Cheng and S. Li, Small, 2022, 18, 2105763 CrossRef CAS PubMed
. - S. Sanati, A. Morsali and H. García, Energy Environ. Sci., 2022, 15, 3119–3151 RSC
. - T. N. Huan, G. Rousse, S. Zanna, I. T. Lucas, X. Xu, N. Menguy, V. Mougel and M. Fontecave, Angew. Chem., Int. Ed., 2017, 56, 4792–4796 CrossRef CAS PubMed
. - Z. Wang, Y. Wang, N. Zhang, L. Ma, J. Sun, C. Yu, S. Liu and R. Jiang, J. Mater. Chem. A, 2022, 10, 10342–10349 RSC
. - J. Kundu, S. Khilari, K. Bhuniaa and D. Pradhan, Catal. Sci. Technol., 2019, 9, 406–417 RSC
. - S. M. Pawar, B. S. Pawar, P. T. Babar, A. T. A. Ahmed, H. S. Chavan, Y. Jo, S. Cho, J. Kim, A. I. Inamdar, J. H. Kim, H. Kim and H. Im, Mater. Lett., 2019, 241, 243–247 CrossRef CAS
. - W. Ahn, M. G. Park, D. U. Lee, M. H. Seo, G. Jiang, Z. P. Cano, F. M. Hassan and Z. Chen, Adv. Funct. Mater., 2018, 28, 1802129 CrossRef
. - X. Wang, W. Zhou, Y.-P. Wu, J.-W. Tian, X.-K. Wang, D.-D. Huang, J. Zhao and D.-S. Li, J. Alloys Compd., 2018, 753, 228–233 CrossRef CAS
. - J. He, F. Liu, Y. Chen, X. Liu, X. Zhang, L. Zhao, B. Chang, J. Wang, H. Liu and W. Zhou, Chem. Eng. J., 2022, 432, 134331 CrossRef CAS
. - H. Yuan, L. Zhao, B. Chang, Y. Chen, T. Dong, J. He, D. Jiang, W. Yu, H. Liu and W. Zhou, Appl. Catal., B, 2022, 314, 121455 CrossRef CAS
. - B. Singh, A. Yadava and A. Indra, J. Mater. Chem. A, 2022, 10, 3843–3868 RSC
. - N. Seal, K. Karthick, M. Singh, S. Kundu and S. Neogi, Chem. Eng. J., 2022, 429, 132301 CrossRef CAS
. - S. Sanati, A. Morsali and H. García, Energy Environ. Sci., 2022, 15, 3119–3151 RSC
. - I. Hod, P. Deria, W. Bury, J. E. Mondloch, C.-W. Kung, M. So, M. D. Sampson, A. W. Peters, C. P. Kubiak, O. K. Farha and J. T. Hupp, Nat. Commun., 2015, 6, 8304 CrossRef CAS PubMed
. - E. M. Miner, T. Fukushima, D. Sheberla, L. Sun, Y. Surendranath and M. Dincă, Nat. Commun., 2016, 7, 10942 CrossRef CAS PubMed
. - Y.-B. Huang, J. Liang, X.-S. Wang and R. Cao, Chem. Soc. Rev., 2017, 46, 126–157 RSC
. - Q. Yang, Q. Xu and H.-L. Jiang, Chem. Soc. Rev., 2017, 46, 4774–4808 RSC
. - T. Drake, P. Ji and W. Lin, Acc. Chem. Res., 2018, 51, 2129–2138 CrossRef CAS PubMed
. - X. Su, Y. Wang, J. Zhou, S. Gu, J. Li and S. Zhang, J. Am. Chem. Soc., 2018, 140, 11286–11292 CrossRef CAS PubMed
. - C.-D. Wu and M. Zhao, Adv. Mater., 2017, 29, 1605446 CrossRef PubMed
. - D.-H. He, J. J. Liu, Y. Wang, F. Li, B. Li and J.-B. He, Electrochim. Acta, 2019, 308, 285–294 CrossRef CAS
. - S. Ji, Y. Chen, S. Zhao, W. Chen, L. Shi, Y. Wang, J. Dong, Z. Li, F. Li, C. Chen, Q. Peng, J. Li, D. Wang and Y. Li, Angew. Chem., Int. Ed., 2019, 58, 4271–4275 CrossRef CAS PubMed
. - A. Goswami, D. Ghosh, V. V. Chernyshev, A. Dey, D. Pradhan and K. Biradha, ACS Appl. Mater. Interfaces, 2020, 12, 33679–33689 CrossRef CAS PubMed
. - M. Jahan, Z. Liu and K. P. Loh, Adv. Funct. Mater., 2013, 23, 5363–5372 CrossRef CAS
. - C. A. Downes and S. C. Marinescu, Dalton Trans., 2016, 45, 19311–19321 RSC
. - R. Dong, Z. Zheng, D. C. Tranca, J. Zhang, N. Chandrasekhar, S. Liu, X. Zhuang, G. Seifert and X. Feng, Chem.–Eur. J., 2017, 23, 2255–2260 CrossRef CAS PubMed
. - Q. Deng, J. Han, J. Zhao, G. Chen, T. Vegge and H. A. Hansen, J. Catal., 2021, 393, 140–148 CrossRef CAS
. - K. Chen, C. A. Downes, E. Schneider, J. D. Goodpaster and S. C. Marinescu, ACS Appl. Mater. Interfaces, 2021, 13, 16384–16395 CrossRef CAS PubMed
. - K. Chen, D. Ray, M. E. Ziebel, C. A. Gaggioli, L. Gagliardi and S. C. Marinescu, ACS Appl. Mater. Interfaces, 2021, 13, 34419–34427 CrossRef CAS PubMed
. - Y.-P. Wu, W. Zhou, J. Zhao, W.-W. Dong, Y.-Q. Lan, D.-S. Li, C. Sun and X. Bu, Angew. Chem., Int. Ed., 2017, 56, 13001–13005 CrossRef CAS PubMed
. - G. Ruan, P. Ghosh, N. Fridman and G. Maayan, J. Am. Chem. Soc., 2021, 143, 10614–10623 CrossRef CAS PubMed
. - P. Muthukumar, D. Moon and S. P. Anthony, CrystEngComm, 2019, 21, 6552–6557 RSC
. - P. Muthukumar, M. Pannipara, A. G. Al-Sehemi, D. Moon and S. P. Anthony, CrystEngComm, 2020, 22, 425–429 RSC
. - P. Muthukumar, D. Moon and S. P. Anthony, Catal. Sci. Technol., 2019, 9, 4347–4354 RSC
. - Q.-F. Chen, Z.-Y. Cheng, R.-Z. Liao and M.-T. Zhang, J. Am. Chem. Soc., 2021, 143, 19761–19768 CrossRef CAS PubMed
. - D. Fa, J. Yuan, G. Feng, S. Lei and W. Hu, Angew. Chem., Int. Ed., 2023 DOI:10.1002/anie.202300532
. - E. Altin, R. Kirchmaier, A. Lentz and Z. Kristallogr, New Cryst. Struct., 2004, 219, 137 CAS
. - M. Felloni, A. J. Blake, P. Hubberstey, C. Wilson and M. Schröder, Cryst. Growth Des., 2009, 9, 4685–4699 CrossRef CAS
.
Footnote |
† Electronic supplementary information (ESI) available: Crystal packing and electrochemical data. CCDC 2240777. For ESI and crystallographic data in CIF or other electronic format see DOI: https://doi.org/10.1039/d3ra01186k |
|
This journal is © The Royal Society of Chemistry 2023 |