DOI:
10.1039/D3RA01031G
(Paper)
RSC Adv., 2023,
13, 10657-10666
Study on the photocatalytic properties differences between the 1-D and 3-D W18O49 particles†
Received
15th February 2023
, Accepted 27th March 2023
First published on 4th April 2023
Abstract
The morphology of W18O49 catalysts has a significant effect on their photocatalytic performance. Herein, we successfully prepared two commonly used W18O49 photocatalysts just by changing the reaction temperature in the hydrothermal system, namely 1-D W18O49 nanowires (1-D W18O49) and 3-D urchin-like W18O49 particles (3-D W18O49), and evaluated the difference of their photocatalytic performances by taking the degradation of methylene blue (MB) as an example. Remarkably, 3-D W18O49 exhibited an impressive photocatalytic degradation performance towards MB with photocatalytic reaction rates of 0.00932 min−1, which was about 3 times higher than that of 1-D W18O49. The comprehensive characterization and control experiments could further reveal that the hierarchical structure of 3-D W18O49 brought higher BET surface areas, stronger light harvesting, faster separation of photogenerated charges and so on, which was the main reason for its better photocatalytic performance. ESR results confirmed that the main active substances were superoxide radicals (˙O2−) and hydroxyl radicals (˙OH). This work aims to explore the intrinsic relationship between the morphology and photocatalytic properties of W18O49 catalysts, so as to provide a theoretical basis in the morphology selection of W18O49 or its composite materials in the field of photocatalysis.
1 Introduction
With the advent of environmental matters and the energy crisis, the oxide semiconductor photo-catalysts have received extensive attention and research given that their energy source is renewable solar energy.1,2 Among various transition metal oxides, earth-abundant tungsten oxides have been proved to be ideal candidates for photocatalytic systems, attributed to their chemical stability, wide bandgap and strong adsorption of the solar spectrum.3,4 Tungsten mainly has three stoichiometric oxides: WO2, W2O5 and WO3, of which WO3 is the most commonly used due to its high sensitivity. In the actual preparation process of WO3, part of the tungsten is reduced to form non-stoichiometric tungsten oxide (WO3−x, 0 ≤ x ≤ 1) with mixed valence states of +6 and +5, such as WO2.90 (W20O58), WO2.83 (W24O68), WO2.80 (W5O14), WO2.72 (W18O49), etc.5,6 In particular, the non-stoichiometric W18O49 structure is rich in more oxygen vacancies and W5+ defects as reaction sites to stimulate adsorption and activation of oxygen molecules, hence W18O49 has incomparable catalytic properties and novel properties for photocatalytic degradation of organic dyes, especially monoclinic phase W18O49.7 To promote the application of W18O49 with excellent properties in more fields, more and more preparation methods have been developed to date, mainly including chemical vapor deposition, sputtering technique, micro-emulsion method, sol–gel method and hydrothermal method.8–12 Among them, hydrothermal route exhibits the most feasibility and research value because of its easy operation, mild conditions, and more importantly, better control on the morphology of prepared particles. It is concluded by reviewing many literature that the photocatalytic efficiency of the materials mainly depends on the surface area, bandgap, crystallinity and so on, and most of these factors are closely related to the morphology of particles.13 Therefore, it is very important to control the W18O49 particle morphology by exploring different reaction conditions in hydrothermal method. And it is more important to study the internal relationship between particle morphology and performance, so as to provide theoretical guidance for selecting suitable particle morphology in different application fields.
At present, water pollution has become an urgent global problem in current environmental matters and energy crisis, especially, the pollution from textile industries would endanger peoples life and affect aquatic life as well from remote antiquity.14–18 Furthermore, methylene blue (MB) is one of the most commonly used organic dyes in the textile industry and is said to be composed of various aromatic amine groups, which can cause serious skin damage, cancerization and respiratory disease.13,19,20 Therefore, finding the effective way to remove organic dyes from wastewater has become the top priority in environmental protection and has attracted wide attention. To better solve the above problems, more and more approaches have been carried out to degrade organic dyes, such as physical absorption, solvent extraction, biodegradation, and photocatalytic oxidation.21–26 Among these methods, photocatalytic degradation, which utilizes semiconductor and light to solve organic dyes, is undoubtedly the most promising one by virtue of its environmental protection, high efficiency and advanced oxidation in sewage disposal.27–29
Having said all of the foregoing, it is very necessary to use tungsten oxide material with many superior photocatalytic performance advantages to photodegrade organic dyes. In addition, given that the morphology of W18O49 particles has a big influence on its photocatalytic properties, various morphologies of W18O49 have been synthesized and tested for their photocatalytic activities. Among the available W18O49 materials, one dimensional (1-D) nanowires and three dimensional (3-D) urchin-like particles are the most desirable and excellent in the field of photocatalysis because of their uniform form and numerous performance advantages.30–34 However, most researchers concentrate solely on investigating the properties of individual materials and their composites, and few deeply study their difference in photocatalytic properties and the fundamental reasons for such differences. The absence of this part of research content is not conducive to our better understanding of the relationship between the structure and properties for W18O49 particle and better application of them in more fields.
With the aim of understanding the effect of morphology on the photocatalytic properties of W18O49 particles, 1-D W18O49 nanowires and 3-D urchin-like W18O49 were first prepared by simply adjusting the reaction temperature in a hydrothermal system, and further taken the degradation of methylene blue as a typical example to explore the catalytic degradation efficiency of two kinds of particles. As a result, within the specified degradation time of 120 minutes, the degradation rate of 3-D urchin-like W18O49 was approximately 3 times than that of 1-D nanowires. Through the analysis of the physical and chemical properties of the two materials, it was concluded that the better catalytic performance of 3-D particles was mainly due to the existence of three-dimensional hierarchical structure with larger specific surface area, which could facilitate the visible light absorption, improve the transfer and separation of electron–hole pairs and enriche the surface electrons at the rod top owing to the multiple light scattering and directional electron transfer induced by the nanotip effect.
2 Experimental section
2.1 Materials
Tungsten(VI) chloride (WCl6, 99.9%) and ethanol were obtained from Tianjin Kemiou Chemical Reagent Co., Ltd. Sodium sulfate (Na2SO4) was purchased from Tianjin Institute of Chemical Agents. All the reagents were of analytical grade and were used without any further purification.
2.2 Characterization of the as-prepared samples
The field-emission scanning electron microscope (SEM, Nano 450, FEI, USA, operated at 10 kV) and transmission electron microscopy (TEM, Talos F200S, FEI, USA, operated at 80 kV) were used to analyze the particle morphology. Energy-disperse X-ray (EDS) was carried out on a field emission scanning electron microscope (Talos F200S) to analyze elemental features of the samples. N2 adsorption–desorption isotherm measurements were used to study the particle specific surface areas using the BET method. The crystallographic properties of the catalysts were analyzed by the X-ray powder diffractometer (Shimadzu XRD-7000). X-ray photoelectron spectroscopy was obtained at PHI-5400 (America PE) 250 xi system. The UV-Vis diffuse reflectance spectra (UV-Vis-DRS) were measured on UV-2550 UV-Vis Spectrophotometer. Photoluminescence (PL) spectroscopy was taken down by an Agilent Cary Eclipse (F-7000) at room temperature. Time-resolved photoluminescence (TRPL) spectra were investigated via a FLS920 fluorescence spectrometer (Edinburgh Analytical Instruments, UK). The X-ray photoelectron spectroscopy (XPS, Kratos Axis Ultra) was used to analyze the chemical composition and element valence. The electron spin resonance (EPR) spectra were recorded via a Bruker ELEXSYS-II E500 CW-EPR. All electrochemical and photoelectrochemical characterizations were carried out through an electrochemical workstation (CHI760E), a three-electrode system (pH 7.0) was adopted, Pt was the counter electrode, Ag/AgCl as the reference electrode, and ITO as the working electrode under visible light. Transient photocurrent response measurements were researched on an electrochemical workstation, the whole process was in the saturated solution of Na2SO4 (0.5 M) under visible light by controlling the light on and off. The electrochemical workstation in Nafion solution was used to test the Electrochemical impedance spectroscopy (EIS).
2.3 Preparation of 1-D W18O49 nanowires
Similar to the synthetic route previously reported.35 In a typical recipe, 0.5 g WCl6 was dissolved in 100 mL absolute ethanol, a clear yellow solution appeared. Then, above yellow solution was poured to a Teflon-lined autoclave and heated at 180 °C for 24 h. A blue flocculent precipitate was formed, purified several times with absolute distilled water and anhydrous ethanol, and vacuum dried at 50 °C.
2.4 Preparation of 3-D urchin-like W18O49
Refer to the synthesis route reported earlier.36 In this process, 0.5 g W6+ WCl6 was dissolved in 100 mL absolute ethanol, a clear yellow solution was formed. Then, above yellow solution was poured to a Teflon-lined autoclave and heated at 200 °C for 24 h. A blue flocculent precipitate was formed, purified several times with absolute distilled water and anhydrous ethanol, and vacuum dried at 50 °C.
2.5 Photocatalyst activity evaluation
20 mg of as-prepared photocatalysts were suspended in 50 mL of aqueous solution of MB (10 mg L−1). Under visible light (400–800 nm) with 300 W Xenon lamp, the photocatalytic degradation of MB experiments was conducted after adsorption/desorption equilibrium (1 h). Photodegradation of MB experiments was investigated by UV-Vis spectrophotometer at 664 nm.
3 Results and discussions
3.1 Morphology and composition characterizations
At first, the morphology, microstructure and elemental composition of as-prepared W18O49 materials under different reaction temperature were systematically characterized through SEM, TEM and the corresponding EDS element-mapping images. As shown in Fig. 1a–c, the obtained particles at a reaction temperature 180 °C were uniform 1-D nanowires with a diameter of about 10 nm. While, Fig. 1d and e showed the typical SEM images of the W18O49 particles with a diameter of about 496 nm obtained at 200 °C, which indicated that these particles had a layered structure consisting of central microsphere and a large number of radial nanowires. Interestingly, through the information of above SEM and TEM images in Fig. 1f, it could be seen that some nanowires on the surface of different urchin-like particles were linked to each other, resulting in the formation of 3D network nanowires. The results of high-resolution TEM images for both particles (the inserts of Fig. 1c and f) verified that the ordered lattice fringes with a spacing of 3.8 Å, which could be attributed to the (010) plane of monoclinic W18O49. These information indicated that the 1-D W18O49 nanowires and 3-D W18O49 both grew along the [010] direction. For 3-D W18O49 particles, the EDS element-mapping images of O and W were shown in Fig. 1h and i, respectively, and we could directly observe the uniform distribution of the two elements. In addition, the tungsten content in the 3-D W18O49 was 86 wt%, as shown in Fig. S1.† These results confirmed that 1-D W18O49 nanowires and 3-D W18O49 could be successfully prepared by simply adjusting the reaction temperature in a hydrothermal system, the corresponding schematic diagram was as shown in Fig. S2.† In the hydrothermal system, the W18O49 molecules growed mainly along the (010) direction, and further formed (WOn)− particles. The particle with negative charges generated faster at 200 °C than at 180 °C, the surface tension and surface energy of the system would further increase, and the particles generated at 200 °C were more likely to gather to three-dimensional W18O49 particles under the principle that the system energy could tend to be kept to a minimum.37 In addition, the direct result of morphological difference was that these two kinds of W18O49 materials display different BET surface areas based on the adsorption and desorption of N2, and the corresponding results were shown in Fig. 2. The 3-D W18O49 particles had a larger specific surface area (120 m2 g−1) than 1-D W18O49 nanowires (75 m2 g−1) due to the existence of hierarchical structure.
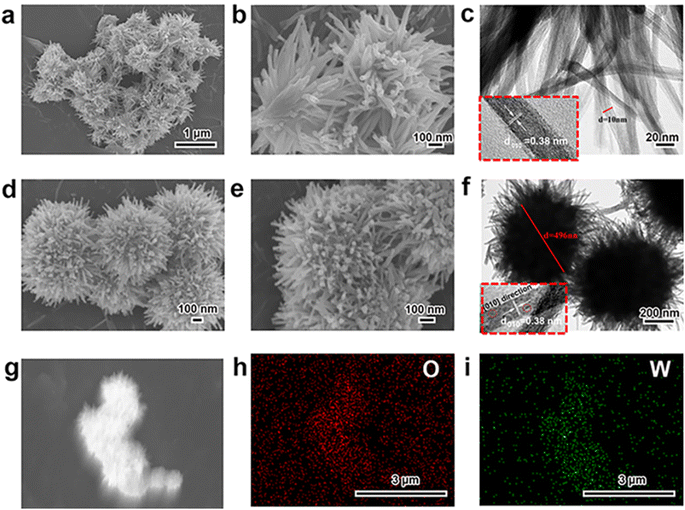 |
| Fig. 1 SEM and TEM images of (a, b, c) 1-D W18O49 nanowires, (d, e, f) 3-D urchin-like W18O49, and (g, h, i) the EDS mapping of the elements O, W in 3-D urchin-like W18O49. | |
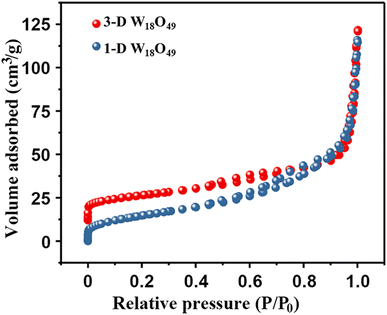 |
| Fig. 2 Nitrogen adsorption–desorption isotherms of 1-D W18O49 nanowires and 3-D urchin-like W18O49. | |
Then, the crystal structure and phase composition of 1-D W18O49 and 3-D W18O49 were exhibited in Fig. 3 by XRD patterns with scanning range from 10° to 80°. And the result (Fig. 3a) showed that the position and relative intensity of the characteristic peaks of two as-prepared W18O49 samples were highly consistent with the monoclinic crystals according to the JCPDS card (no. 71-2450).38 Specifically, the narrow and pointed peak at 23.2° matched with the (010) plane, and the weak reflection at 47.5° was in accordance with the (020) plane. Moreover, the diffraction intensities of other peaks were so weak as to be negligible. In addition, the peaks in XRD were slightly out of position with standard cards, which was due to a large number of oxygen vacancies in two sample.39,40 Thus, the crystals of two W18O49 samples both preferentially tended to grow in (010) direction based on XRD results, which cohered with the TEM results in Fig. 1.
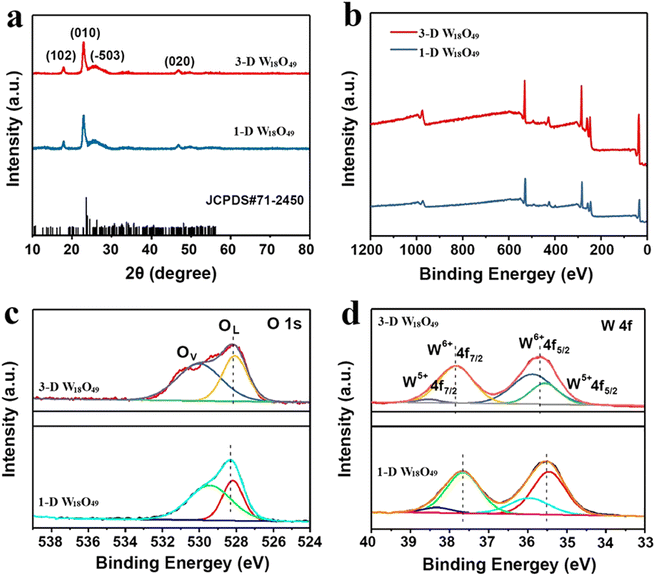 |
| Fig. 3 (a) XRD patterns and (b) XPS spectra of 1-D W18O49 nanowires and 3-D urchin-like W18O49, and the corresponding high-resolution XPS spectra of (c) O 1s and (d) W 4f. | |
The detailed chemical states and chemical components of obtaining W18O49 samples were further analyzed by XPS spectra, the results were displayed in Fig. 3b–d. For accuracy, the binding energy for all the results had been corrected by C1s peak at 284.8 eV. First, the elements W, O in the 1-D W18O49 and 3-D W18O49 could be confirmed by the scanning survey spectra in Fig. 3b, and this result was in accordance with EDS mapping results (Fig. 1h and i). Moreover, the oxygen vacancies of 1-D and 3-D W18O49 were further detected by high-resolution XPS spectra of O1s (Fig. 3c), and O1s spectra of two samples were both divided into two main peaks. One peak located at 530.2 eV corresponded to the lattice oxygen of W–O, while the other one located at 531.2 eV was assigned to the oxygen vacancy. This result was a direct proof of the existence of oxygen vacancies in 1-D and 3-D W18O49, and the oxygen vacancy ratio of 3-D W18O49 was significantly higher than that of 1-D W18O49. Additionally, since the EPR spectroscopy is also a highly effective characterization for the detection surface oxygen vacancies of prepared two photo-catalysts. And we could conclude that the oxygen vacancy in 3-D W18O49 was more abundant than 1-D W18O49 from the EPR signals in Fig. S3,† which was consistent with above XPS results in Fig. 3c. Moreover, two different valence states of W element (namely W6+, W5+) corresponding to W4f5/2 and W4f7/2 could be observed in the W4f high-resolution XPS spectrum (Fig. 3d). For 3-D W18O49, the peaks at 35.70 and 38.50 eV were rated to W4f7/2 and W4f5/2 characteristic peaks of W6+, respectively. The second double peak with binding energies of 34.91 and 37.10 eV were attributed to the W4f7/2 and W4f5/2 characteristic peaks of W5+. The emergence of the low valence state W5+ was attributed to the short W–W distances (0.26 nm) and the removal of oxygen occurs at the shear planes, which could further verify the existence of a large number of oxygen vacancies.41,42 In addition, the binding energy of W 4f for 1-D W18O49 shifts by 0.2 eV toward lower value than that of the 3-D W18O49, which was attributed to the existence of larger oxygen vacancies in 3-D W18O49. The oxygen vacancies and W5+ defects could provide more active sites and would have important influence on the photocatalytic properties of as-prepared W18O49. To better study the photocatalytic properties and compare their differences of two forms of W18O49, the optical properties and separation efficiency of both photo-catalysts were explored by a sequence of photoelectrochemical characterizations.
3.2 Optical properties of prepared catalysts
It is well known that the optical absorption efficiency plays a decisive role in determining of photocatalytic performance.43 Herein, the light trapping properties of 1-D W18O49 nanowires and 3-D urchin-like W18O49 were analyzed by UV-Vis-NIR absorption spectrum, and the related information was shown in Fig. 4. Two different dimensional sizes of W18O49 particles both presented a large absorption throughout the visible and near-infrared regions, which could be attributed to the plasmon resonance absorption driven by the existence of lots of oxygen vacancies in W18O49. Among them, 3-D W18O49 had stronger responsiveness in the UV-Vis-NIR region than 1-D W18O49, and the relevant proof information was shown in Fig. 4a. We could ascribe this phenomenon to the existence of 3-D urchin-like hierarchical structure, which not only could provide large surface area (Fig. 2) but also facilitate the multiple light scattering between the radial nanorods.44,45 Besides, the bandgap information of the as-prepared samples was further studied by the relevant Tauc plots of UV-Vis spectra, as shown in Fig. 4b. The band gap was calculated using the Kubelka–Munk eqn (1).46where α, h, v and k represent the diffuse absorption coefficient, plank constant, light frequency and a constant, respectively. It was estimated that the band gap of 3-D W18O49 (3.35 eV) was lower than that of 1-D W18O49 nanowires (3.70 eV), which could be inferred from Fig. 4b. Therefore, the light absorption range of 3-D urchin-like W18O49 was extended and then the light utilization efficiency was improved, which was crucial to obtain a better photocatalytic performance.23,47 In addition, the potential of the valence band (VB) of two W18O49 semiconductors was determined through the Mott–Schottky measurements.48,49 And the relevant results were shown in the Fig. S4,† the VB value of 1-D W18O49 and 3-D W18O49 were evaluated to be about 3.16 eV and 3.01 eV, respectively. Mott–Schottky (MS) plot was employed to investigate the CB of 3-D W18O49.50,51 As shown in Fig. S5,† an obvious positive slope for MS plot was observed, indicating 3-D W18O49 belonged to n-type semiconductors. In fact, the position of the CB is close to the flat potential of the n-type semiconductors. The flat potential of 3-D W18O49 was further determined to be −0.54 eV (vs. Ag/AgCl at pH 7). The corresponding CB of 3-D W18O49 was converted to be about −0.34 eV (vs. NHE) according to the eqn (2). Based on the eqn (3), the VB of 3-D W18O49 was calculated to be +3.01 eV. |
ENHE = EAg/AgCl + 0.197
| (2) |
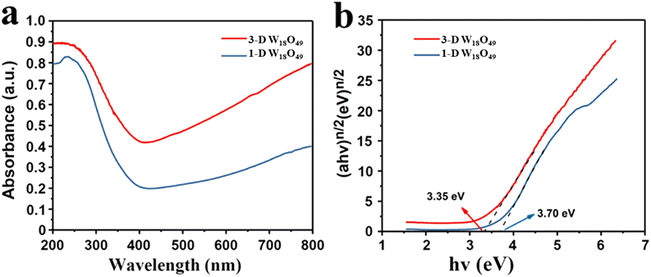 |
| Fig. 4 (a) The UV-Vis diffuse absorbance spectra and (b) the corresponding plots of (αhν)n/2 vs. photoenergy (hv) of 1-D W18O49 nanowires and 3-D urchin-like W18O49. | |
3.3 The separation efficiency of photogenerated charge carriers
To comprehensively investigate the separation and transfer efficiency of photo-excited charges over the photo-catalysts, the PL, time-resolved PL (TRPL) and EIS of the samples were carried out, and the detailed characterization results were presented in Fig. 5. Firstly, as shown in Fig. 5a, the fluorescence signal of 3-D urchin-like W18O49 was lower than that of W18O49 nanowires, which indicated that the 3-D W18O49 had more efficient charge separation efficiency. Furthermore, the TRPL was been demonstrated to be an effective tool for studying the dynamics of specific charge carrier, and the relevant TRPL results of 1-D W18O49 and 3-D W18O49 were shown in the Fig. 5b. It was obvious that the average lifetime value of 3-D W18O49 (20.23 ns) was longer than that of the 1-D W18O49 (5.45 ns), indicating that the 3-D W18O49 particle could appear larger defects, which had also been verified by the EPR technique (Fig. S1†). Generally, the higher photocurrent value also stands for more photogenerated electrons and higher separation efficiency. When the light source was switched on and off at 20 second intervals, the photocurrent signals could show vigorous spikes and decreases. Notably, the 3-D W18O49 had a higher signal (Fig. 5c) under the light illumination, which corresponded to higher light trapping and lower photogenerated charge recombination efficiency. To better explain above research results, there were differences in electron transfer efficiency between the two as-prepared catalysts. And the EIS was used to explore the real reason. As shown in Fig. 5d, the 3-D W18O49 photocatalyst possessed smaller radius, which further implied 3-D W18O49 had lower charge transfer impedance and higher charge separation efficiency in comparison with 1-D W18O49.
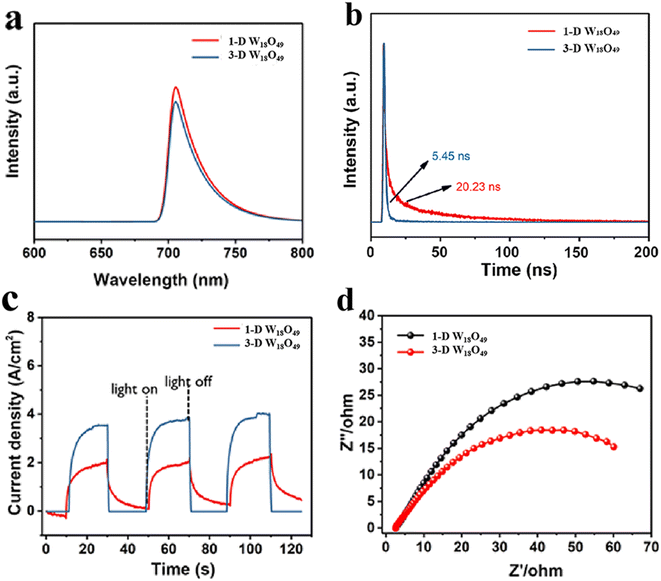 |
| Fig. 5 (a) Photoluminescence spectra, (b) time-resolved transient PL decay (c) photocurrent transient responses and (d) electrochemical impedance spectra of 1-D W18O49 nanowires and 3-D urchin-like W18O49. | |
3.4 Photocatalytic activity of the prepared photocatalysts
As shown above, the morphological difference of W18O49 particles brought about the difference in the surface area (Fig. 2), light absorption (Fig. 4) and separation efficiency of photogenerated charge (Fig. 5). These properties were important factors that could determine the photocatalytic performance of the photocatalysts.52,53
Herein, the degradation behavior of MB was taken as a typical example to investigate the specific photocatalytic activity of 1-D W18O49 nanowires and 3-D urchin-like W18O49, the corresponding degradation activities and mechanism were shown in the Fig. 6. The dye concentration remained same under all experimental conditions. To exclude the effect of adsorption on the reduction of MB, the MB solution was exposed to dark conditions for 20 minutes. As shown in Fig. 6a, the MB content was not reduced in the dark, which indicated that the reduction of MB content in the system was only related to the photodegradation of the photocatalyst. In addition, the MB solution containing the two catalysts was irradiated for 120 minutes to compare the photocatalytic degradation rate of MB by the two catalysts. When W18O49 nanowires was used in the photocatalytic system, the concentration of MB decreased by 45% within 120 minutes, and 70% MB had been degraded in 120 min when 3D urchin-like W18O49 was employed. According to following eqn (4),54 the values of photocatalytic reaction rates of 1-D W18O49 nanowires and 3-D urchin-like W18O49 were measured to be 0.00312 min−1 and 0.00932 min−1 respectively, as shown in Fig. 5b.
where
k is the apparent rate constant,
t is the reaction time,
Ct is the concentration at irradiation time
t,
C0 is the initial concentration. Furthermore, the time dependent absorption spectra of MB dye in the presence of 1-D W
18O
49 and 3-D W
18O
49 photocatalysts were shown separately in the
Fig. 6c and d. The MB dye showed strong characteristic absorption peak at 670 nm and shoulder peak at 616 nm.
55 The results showed that the absorption maximum had a steady decline trend within 120 minutes no matter what kind of nanomaterials existed, however, the reduction rate of MB in 3-D urchin-like W
18O
49 catalysis was much faster than that in 1-D W
18O
49 nanowires. As discussed above, the 3-D W
18O
49 has been found to exhibit better catalytic performance and catalytic rate for the decomposition of MB than that of W
18O
49 nanowires. Furthermore, it was found that the morphology of the 3-D W
18O
49 particles did not change and still had a three-dimensional structure after the photocatalytic degradation of MB (Fig. S6
†), which indicated that the particle was expected to achieve reuse in the field of photocatalytic degradation.
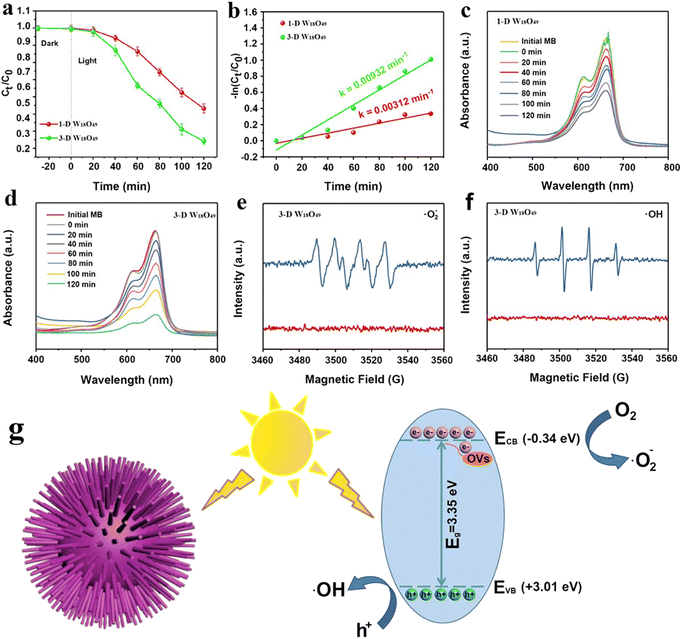 |
| Fig. 6 (a) The photocatalytic degradation of methylene blue and (b) plot of ln Ct/C0 vs. time under simulated solar irradiation over 1-D W18O49 nanowires and 3-D urchin-like W18O49, the UV-Vis spectral changes of methylene blue as a function of irradiation time in the presence of (c) 1-D W18O49 nanowires and (d) 3-D urchin-like W18O49, the EPR spectra of (e) ˙O2− and (f) ˙OH for 3-D urchin-like W18O49, and (g) the schematic illustration of the reaction mechanism involved in the photocatalytic activity of 3-D urchin-like W18O49. | |
It is well known that the produced ˙OH and ˙O2− radicals had a major impact on the degradation efficiency. To better study the mechanism of 3-D urchin-like W18O49, the low-temperature ESR technology was carried to study the active radicals. It was obvious that the signal of ˙O2− (Fig. 6e) could be observed in the 3-D urchin-like W18O49. Meanwhile, the ˙OH signal (Fig. 6f) was also detected under the same conditions. Based on the above experimental results and discussions, we proposed a possible mechanism for the better photocatalytic performance of 3-D urchin-like W18O49 particle, as shown in Fig. 6g. The conduction band (CB) and valence band (VB) of 3-D urchin-like W18O49 were further determined. And the corresponding CB and VB of 3-D urchin-like W18O49 were estimated to be about −0.34 and +3.01 eV, respectively. The CB potential (−0.34 eV) of 3-D urchin-like W18O49 was more negative than that of Eθ (˙O2−/O2) (−0.33 eV), while the active species (˙O2−) could be generated. Besides, and VB potential (+3.01 eV) of 3-D urchin-like W18O49 was more positive that of Eθ (˙OH/H2O) (+1.99 eV), the active species (˙OH) might be formed, which was consistent with the ESR results. Under visible light excitation, a large number of photogenerated electrons and holes were produced on 3-D urchin-like W18O49. Photogenerated electrons on 3-D urchin-like W18O49 could capture O2 and generate ˙O2− due to more negative potential, while, holes could directly oxidize H2O into ˙OH. Compared to the 1-D W18O49 nanowires, the unique three-dimensional hierarchical structure in 3-D W18O49 would be more effective in harvesting light through multiple scattering between the radial nanorods, which could generate more electrons and holes. Meanwhile, lots of electrons concentrated on the tip of the nanorods because of the tip effect, and therefore, 3-D W18O49 was more conducive to adsorption, activation and degradation of pollutants like MB. Then, the photo-induced electrons could interact with oxygen and water adsorbed on the surface to generate active radicals ˙O2− and ˙OH, which could contribute to the degradation of MB.56 Therefore, the larger surface area, more light absorption and higher effective charge separation brought by the unique 3-D urchin-like structure work synergistically, resulting in the excellent photocatalytic performance.
4 Conclusions
1-D W18O49 nanowire and 3-D urchin-like W18O49 particle were successfully synthesized under different reaction temperatures through the hydrothermal method. In addition, we systematically evaluated the differences in photocatalytic performance between these two particles. The comprehensive characterizations and control experiments indicted that the hierarchical urchin-like structure given 3-D W18O49 particle a larger BET surface areas (120 m2 g−1) than 1-D W18O49 nanowires (75 m2 g−1), which led to more excellent optical properties of 3-D urchin-like W18O49 particle: the larger light absorption capacity and light utilization efficiency; lower charge transfer impedance and complexation efficiency of photogenerated charges, and so on. Furthermore, the actual photocatalytic performance was measured by taking MB degradation as an example, the result was that the photocatalytic reaction rates of 3-D urchin-like W18O49 particles was about three times than that of 1-D W18O49 nanowires. This study is helpful for us to better understand the influence of morphology on the photocatalytic performance of W18O49 material, which is conducive to the better application of W18O49 or their composites in the field of photocatalysis.
Data availability
Juan Wang: investigation, methodology, writing-original draft, funding acquisition. Jin Ye: methodology, experimental characterization, writing-editing.
Conflicts of interest
The authors declare that they have no known competing financial interests or personal relationships that could have appeared to influence the work reported in this paper.
Acknowledgements
Financial support from the Talent Introduction Project (40621051).
References
- X. Wang, X. Zhang, Y. Zhang, Y. Wang, S.-P. Sun, W. D. Wu and Z. Wu, Nanostructured Semiconductor Supported Iron Catalysts for Heterogeneous Photo-Fenton Oxidation: A Review, J. Mater. Chem. A, 2020, 8(31), 15513–15546 RSC
. - J. Ye, J. Xu, C. Li, D. Ti, X. Zhao, Q. Wang, W. Lv, J. Wang, H. Xie, Y. Li, Z. Liu and Y. Fu, Novel N-Black In2O3-x/InVO4 Heterojunction for Efficient Photocatalytic Fixation: Synergistic Effect of Exposed (321) Facet and Oxygen Vacancy, J. Mater. Chem. A, 2021, 9(43), 24600–24612 RSC
. - D. Yun, E. Ayla, D. Bregante and D. Flaherty, Reactive Species and Reaction Pathways for the Oxidative Cleavage of 4-Octene and Oleic Acid with H2O2 over Tungsten Oxide Catalysts, ACS Catal., 2021, 11(593), 3137–3152 CrossRef CAS
. - Z. Jin, J. Li, D. Liu, Y. Sun, X. Li, Q. Cai, H. Ding and J. Gui, Effective Promotion of Spacial Charge Separation of Dual S-scheme (1D/2D/0D) WO3@ZnIn2S4/Bi2S3 Heterojunctions for Enhanced Photocatalytic Performance under Visible Light, Sep. Purif. Technol., 2022, 284(2), 120207 CrossRef CAS
. - X. Xiao, T. Ding, L. Yuan, Y. Shen, Q. Zhong, X. Zhang, Y. Cao, B. Hu, T. Zhai, L. Gong, J. Chen, Y. Tong, J. Zhou and Z. Wang, WO3-x/MoO3-x Core/Shell Nanowires on Carbon Fabric as an Anode for All-Solid-State Asymmetric Supercapacitors, Adv. Energy Mater., 2012, 2(11), 1328–1332 CrossRef CAS
. - C. Valentin, F. Wang and G. Pacchioni, Tungsten Oxide in Catalysis and Photocatalysis: Hints from DFT, Top. Catal., 2013, 56(15–17), 1404–1419 CrossRef
. - J. Liang, J. Yu, W. Xing, W. Tang, N. Tang and J. Guo, 3D interconnected Network Architectures Assembled from W18O49 and Ti3C2 MXene with Excellent Electrochemical Properties and CDI Performance, Chem. Eng. J., 2022, 435, 134922 CrossRef CAS
. - L. Han, J. Xia, X. Hai, Y. Shu, X. Chen and J. Wang, Protein-Stabilized Gadolinium Oxide-Gold Nanoclusters Hybrid for Multimodal Imaging and Drug Delivery, ACS Appl. Mater. Interfaces, 2017, 9(8), 6941–6949 CrossRef CAS PubMed
. - Z. Wang, M. Hu and Y. Qin, Solvothermal Synthesis of WO3 Nanocrystals with Nanosheet and Nanorod Morphologies and the Gas-Sensing Properties, Mater. Lett., 2016, 171, 146–149 CrossRef CAS
. - C. Balázsi, L. Wang, E. Zayim, I. Szilágyi, K. Sedlacková, J. Pfeifer, A. Tóth and P. Gouma, Nanosize Hexagonal Tungsten Oxide for Gas Sensing Applications, J. Eur. Ceram. Soc., 2008, 28(5), 913–917 CrossRef
. - A. Aral and J. Gardeniers, Synthesis and Atmospheric Pressure Field Emission Operation of W18O49 Nanorods, J. Phys. Chem. C, 2008, 112(39), 15183–15189 CrossRef
. - C. Guo, S. Yin, Y. Huang, Q. Dong and T. Sato, Synthesis of W18O49 Nanorod via Ammonium Tungsten Oxide and Its Interesting Optical Properties, Langmuir, 2011, 27(19), 12172–12178 CrossRef CAS PubMed
. - B. Bishal, B. Paul, S. S. Dhar and S. Vadivel, Facile Hydrothermal Synthesis of Ultrasmall W18O49 Nanoparticles and Studies of Their Photocatalytic Activity towards Degradation of Methylene Blue, Mater. Chem. Phys., 2017, 188, 1–7 CrossRef
. - N. Meng, B. Jin, C. Chow and C. Saint, Recent Developments in Photocatalytic Water Treatment Technology: A Review, Water Res., 2010, 44(10), 2997–3027 CrossRef PubMed
. - S. Mei, M. Pan, J. Wang, X. Zhang, S. Song, C. Li and G. Liu, Self-assembly of Strawberry-like Organic-inorganic Hybrid Particle Clusters with Directionally Distributed Bimetal and Facile Transformation of the Core and Corona, Polym. Chem., 2020, 11(18), 3136–3151 RSC
. - J. Wang, M. Pan, J. Yuan, Q. Lin, X. Zhang, G. Liu and L. Zhu, Hollow Mesoporous Silica with Hierarchical Shell from in-situ Synergetic Soft-Hard Double Templates, Nanoscale, 2020, 12(19), 10863–10871 RSC
. - Y. Fu, K. Zhang, Y. Zhang, Y. Cong and Q. Wang, Fabrication of Visible-light-active MR/NH2-MIL-125(Ti) Homojunction with Boosted Photocatalytic Performance, Chem. Eng. J., 2021, 412, 128722 CrossRef CAS
. - J. Guo, D. Ma, F. Sun, G. Zhuang, Q. Wang, A. Al-Enizi, A. Nafady and S. Ma, Substituent Engineering in g-C3N4/COF Heterojunctions for Rapid Charge Separation and High Photo-redox Activity, Sci. China: Chem., 2022, 65(9), 1704–1709 CrossRef CAS
. - K. G. Bhattacharyya and A. Sharma, Kinetics and Thermodynamics of Methylene Blue Adsorption on Neem (Azadirachta Indica) Leaf Powder, Dyes Pigm., 2005, 65(1), 51–59 CrossRef CAS
. - J. Galvan, M. X. Borsoi, L. Julek, D. Bordin and F. B. T. Alves, Methylene Blue for the Treatment of Health Conditions: a Scoping Review, Braz. Arch. Biol. Technol., 2021, 64(4), e21200266 CrossRef CAS
. - W. Lü, Y. Wu, J. Chen and Y. Yang, Facile Preparation of Graphene-Fe3O4 Nanocomposites for Extraction of Dye from Aqueous Solution, CrystEngComm, 2014, 16, 609–615 RSC
. - E. Cserhati, E. Forgacs and G. Oros, Removal of Synthetic Dyes from Wastewater: A Review, Environ. Int., 2004, 30, 953–971 CrossRef PubMed
. - R. A. Pereira, A. F. Salvador, P. Dias and M. F. R. Pereira, Perspectives on Carbon Materials as Powerful Catalysts in Continuous Anaerobic Bioreactors, Water Res., 2016, 101(15), 441–447 CrossRef CAS PubMed
. - N. Abdullah, M. Mohamed, N. Shohaimi, A. Lazim, A. Halim, N. Shukri and M. Razab, Enhancing the Decolorization of Methylene Blue Using a Low-Cost Super-Absorbent Aided by Response Surface Methodology, Molecules, 2021, 26(15), 4430 CrossRef CAS PubMed
. - G. Zheng, Y. Cui, Y. Zhou, Z. Jiang, Q. Wang, M. Zhou, P. Wang and Y. Yu, Photoenzymatic Activity of Artificial-Natural Bienzyme Applied in Biodegradation of Methylene Blue and Accelerating Polymerization of Dopamine, ACS Appl. Mater. Interfaces, 2021, 13(47), 56191–56204 CrossRef CAS PubMed
. - M. Xu, S. Ma, J. Li and M. Yuan, Multifunctional 3D Polydimethylsiloxane Modified MoS2@biomass-Derived Carbon Composite for Oil/Water Separation and Organic Dye Adsorption/Photocatalysis, Colloids Surf., A, 2022, 637, 128281 CrossRef CAS
. - C. Muthukemar, S. Alam, E. lype and K. Prakash, Statistical Analysis of Photodegradation of Methylene Blue Dye under Natural Sunlight, Opt. Mater., 2021, 122(8), 111809 CrossRef
. - L. Bi, Z. Chen, L. Li, J. Kang and J. Shen, Selective Adsorption and Enhanced Photodegradation of Diclofenac in Water by Molecularly Imprinted TiO2, J. Hazard. Mater., 2021, 407(5), 124759 CrossRef CAS PubMed
. - W. Ma, Y. Li, M. Zhang, S. Gao and G. Fu, Biomimetic Durable Multifunctional Self-Cleaning Nanofibrous Membrane with Outstanding Oil/Water Separation, Photodegradation of Organic Contaminants, and Antibacterial Performances, ACS Appl. Mater. Interfaces, 2020, 12(31), 34999–35010 CrossRef CAS PubMed
. - C. Wang, Y. Lei, Q. Lv, W. Kong, F. Wan and W. Chen, Abundant Oxygen Vacancies Promote Bond Breaking of Hydrogen Peroxide on 3d Urchin-Like Pd/W18O49 Surface to Achieve High-Performance Catalysis of Hydroquinone Oxidation, Appl. Catal., B, 2022, 315, 121547 CrossRef CAS
. - H. Zhang, Y. Wang, S. Zuo, W. Zhou, J. Zhang and X. Lou, Isolated Cobalt Centers on W18O49 Nanowires Perform as a Reaction Switch for Efficient CO2 Photoreduction, J. Am. Chem. Soc., 2021, 143(5), 2173–2177 CrossRef CAS PubMed
. - Y. Li, W. Chen, Z. Liu, D. Cao, Y. Chen, K. Thummavichai, N. Wang and Y. Zhu, In situ Fabrication of Porous Biochar Reinforced W18O49 Nanocomposite for Methylene Blue Photodegradation, RSC Adv., 2022, 12, 14902–14911 RSC
. - M. Li, S. Zhang, M. Alwafi, J. Han and H. Wang, Multiple Light Scattering and Nanotip Effect of Hierarchical Sea Urchin-like W18O49 Boosting Photocatalytic Hydrolysis of Ammonia Borane, Int. J. Hydrogen Energy, 2021, 46(36), 18964–18976 CrossRef CAS
. - N. Zhang, Y. Zhao, Y. Lu and G. Zhu, Preparation of Aligned W18O49 Nanowire Clusters with High Photocatalytic Activity, Mater. Sci. Eng., B, 2017, 218, 51–58 CrossRef CAS
. - G. Xi, S. Ouyang, P. Li, J. Ye, Q. Ma, N. Su, H. Bai and C. Wang, Ultrathin W18O49 Nanowires with Diameters below 1 nm: Synthesis, Near-Infrared Absorption, Photoluminescence, and Photochemical Reduction of Carbon Dioxide, Angew. Chem., Int. Ed., 2012, 51(10), 2395–2399 CrossRef CAS PubMed
. - S. Park, H.-W. Shim, C. W. Lee, H. J. Song, J.-C. Kim and D.-W. Kim, High-power and Long-life Supercapacitive Performance of Hierarchical, 3-D Urchin-like W18O49 Nanostructure Electrodes, Nano Res., 2016, 9, 633–643 CrossRef CAS
. - J. Polleux, N. Pinna, M. Antonietti and M. Niederberger, Growth and Assembly of Crystalline Tungsten Oxide Nanostructures Assisted by Bioligation, J. Am. Chem. Soc., 2005, 127, 15595–15601 CrossRef CAS PubMed
. - Z. Chen, Q. Wang, H. Wang, L. Zhang and G. Song, Ultrathin PEGylated W18O49 Nanowires as a New 980nm-Laser-Driven Photothermal Agent for Efficient Ablation of Cancer Cells In Vivo, Adv. Mater., 2013, 25(14), 2095–2100 CrossRef CAS PubMed
. - C. Chen, T. Jiang, J. Hou, T. Zhang, G. Zhang, Y. Zhang and X. Wang, Oxygen Vacancies induced Narrow Band Gap of BiOCl for Efficient Visible-light Catalytic Performance from Double Radicals, J. Mater. Sci. Technol., 2022, 114, 240–248 CrossRef
. - T. Xiong, Y. Zhang, W. S. V. Lee and J. Xue, Defect Engineering in Manganese-Based Oxides for Aqueous Rechargeable Zinc-Ion Batteries: A Review, Adv. Energy Mater., 2020, 10, 2001769 CrossRef CAS
. - P. Chen, M. Qin, D. Zhang, Z. Chen, B. Jia, Q. Wan, H. Wu and X. Qu, Combustion Synthesis and Excellent Photocatalytic Degradation Properties of W18O49, CrystEngComm, 2015, 39(2), 1196–1201 CAS
. - S. Park, H.-W. Shim, C. W. Lee, H. J. Song, J.-C. Kim and D.-W. Kim, High-power and Long-life Supercapacitive Performance of Hierarchical, 3-D Urchin-Like W18O49 Nanostructure Electrodes, Nano Res., 2016, 9(3), 633–643 CrossRef CAS
. - H. Bai, N. Su, W. Li, X. Zhang, Y. Yan, P. Li, S. Quyang, J. Ye and G. Xi, W18O49 Nanowire Networks for Catalyzed Dehydration of Isopropyl Alcohol to Propylene under Visible Light, J. Mater. Chem. A, 2013, 1(20), 6125–6129 RSC
. - Y. Liu, Z. Zhang, Y. Fang, B. Liu, J. Huang, F. Miao, Y. Bao and B. Dong, IR-Driven Strong Plasmonic-Coupling on Ag Nanorices/W18O49 Nanowires
Heterostructures for Photo/Thermal Synergistic Enhancement of H2 Evolution from Ammonia Borane, Appl. Catal., B, 2019, 252, 164–173 CrossRef CAS
. - W. Ren, H. Zhang, D. Kong, B. Liu, Y. Yang and C. Cheng, A Three-dimensional Hierarchical TiO2 Urchin as a Photoelectrochemical Anode with Omnidirectional Anti-Reflectance Properties, Phys. Chem. Chem. Phys., 2014, 16, 22953–22957 RSC
. - G. Eshaq, S. Wang, H. Sun and M. Sillanpää, Core/shell FeVO4@BiOCl Heterojunction as a Durable Heterogeneous Fenton Catalyst for the Efficient Sonophotocatalytic Degradation of P-nitrophenol, Sep. Purif. Technol., 2020, 16(231), 115915 CrossRef
. - K. Mori, K. Miyawaki and H. Yamashita, Ru and Ru-Ni Nanoparticles on TiO2 Support as Extremely Active Catalysts for Hydrogen Production from Ammonia-Borane, ACS Catal., 2016, 6(5), 3128–3135 CrossRef CAS
. - H. Liang, A. Acharjya, D. Anito, S. Vogl and B. Han, Rhenium-Metalated Polypyridine-Based Porous Polycarbazoles for Visible-Light CO2 Photoreduction, ACS Catal., 2019, 9(5), 3959–3968 CrossRef CAS
. - H. Xu, J. Hu, D. Wang, Z. Li, Q. Zhang, Y. Luo, S.-H. Yu and H.-L. Jiang, Visible-Light Photoreduction of CO2 in a Metal-Organic Framework: Boosting Electron-Hole Separation via Electron Trap States, J. Am. Chem. Soc., 2015, 137(42), 151004174149004 CrossRef PubMed
. - Y. Fu, M. Tan, Z. Guo, D. Hao, Y. Xu, H. Du, C. Zhang, J. Guo, Q. Li and Q. Wang, Fabrication of Wide-spectra-responsive NA/NH2-MIL-125(Ti) with Boosted Activity for Cr(VI) Reduction and Antibacterial Effects, Chem. Eng. J., 2023, 452, 139417 CrossRef CAS
. - S. Zheng, H. Du, L. Yang, M. Tan, N. Li, Y. Fu, D. Hao and Q. Wang, PDINH bridged NH2-UiO-66(Zr) Z-scheme Heterojunction for Promoted Photocatalytic Cr(VI) Reduction and Antibacterial Activity, J. Hazard. Mater., 2023, 447, 130849 CrossRef CAS PubMed
. - S. Thirumalairajan, K. Girija, V. Mastelaro and N. Ponpandian, Photocatalytic Degradation of Organic Dyes under Visible Light Irradiation by Floral-like LaFeO3 Nanostructures Comprised of Nanosheet Petals, New J. Chem., 2014, 38(11), 5480–5490 RSC
. - J. Chen, J. Liu, S. Qiao, R. Xu and X. Lou, Formation of Large 2D Nanosheets via PVP-assisted Assembly of Anatase TiO2 Nanomosaics, Chem. Commun., 2011, 47(37), 10443–10445 RSC
. - J. Wang and T. Nonami, Photocatalytic Activity for Methylene Blue Decomposition of NaInO2 with a Layered Structure, J. Mater. Sci., 2004, 39(20), 6367–6370 CrossRef CAS
. - Y. Zhao, Y. Wang, E. Liu, J. Fan and X. Hu, Bi2WO6 Nanoflowers: An Efficient Visible Light Photocatalytic Activity for Ceftriaxone Sodium Degradation, Appl. Surf. Sci., 2018, 436, 854–864 CrossRef CAS
. - B. Bhuyan, B. Paul, S. S. Dhar and S. Vadivel, Facile Hydrothermal Synthesis of Ultrasmall W18O49 Nanoparticles and Studies of Their Photocatalytic Activity towards Degradation of Methylene Blue, Mater. Chem. Phys., 2017, 188, 1–7 CrossRef CAS
.
|
This journal is © The Royal Society of Chemistry 2023 |
Click here to see how this site uses Cookies. View our privacy policy here.