DOI:
10.1039/D3RA00856H
(Paper)
RSC Adv., 2023,
13, 11241-11248
Toxic effects and bioaccumulation of pinacolyl methylphosphonate acid in zebrafish following soman exposure to a water environment†
Received
8th February 2023
, Accepted 14th March 2023
First published on 11th April 2023
Abstract
Soman has been shown to be highly neurotoxic and can be easily degraded to produce pinacolyl methylphosphonate acid (PMPA). Thus, the perniciousness of PMPA deserved serious attention after soman was exposed to the environment. However, the toxicity of PMPA was not clearly elucidated to date. In this regard, the objective of this study was to determine if PMPA could pose an environmental risk after soman exposure to a water environment. In this study, the toxicity and bioaccumulation assessments of PMPA were carried out on zebrafish. Histological examination was used to assess the toxicity of PMPA in zebrafish and revealed that PMPA has chronic toxicity in view of tissue injury. The contents of PMPA in whole zebrafish and tissues were determined after soman exposure. The result showed that PMPA bioaccumulated in the whole zebrafish and tissue, especially the liver and intestinal tissues. This is the first report showing that the hydrolyzate of a G-series chemical nerve agent could accumulate in organisms. This study offers novel insights into the environmental risk assessments associated with soman exposure to a water environment.
1 Introduction
Chemical nerve agents are still retained and stored in some non-party states of the Organization for the Prohibition of Chemical Weapons (OPCW) and have even not been completely destroyed in all the party states of the OPCW.1 In addition, a large number of abandoned chemical nerve agents exist in many countries, such as China and Japan.2,3 Hundreds of thousands of tons of chemical nerve agents were dumped into the ocean following World War II in many places, such as European, Russian, Japanese, and United States coasts, and the Baltic and North Seas.4 Pinacolyl methylphosphonofluoridate (soman) is a kind of G-series chemical nerve agent.5 Soman could be exposed to the environment in various ways, during its destruction, use, storage, and transportation.
Soman has fatal neurotoxicity; its main mechanism of action is by irreversibly inhibiting acetylcholinesterase.6 The half lethal dose (LD50) values of soman in intravenous administration were reported to be 4, 10, 15, and 35 μg kg−1 in rats, dogs, cats, and mice, respectively.7–10 In 6 dpf zebrafish larvae, the calculated LC50-24 h values of soman were reported to be 21.8, 4.0, 2.3, and 1.3 μM following exposure times of 30, 60, 90, and 120 min, respectively.11 To the best of our knowledge, the toxicity of PMPA is little reported, especially in animals. Aquatic toxicity studies showed that the hydrolyzate of soman is toxic and unsuitable for disposal to sanitary sewers.12 On cultured cortical neurons, PMPA inhibited network mean burst and spike rates with an EC50 of approximately 2 mM and could interfere with excitatory synaptic transmission.13 PMPA was estimated to have a relatively mild chronic toxicity in the BIOWIN model.14 Among the PMPA analogues, isopropyl methylphosphonate acid (IMPA) is the main degradation product of sarin, and the acute LD50 value of IMPA was determined to be 5620 mg kg−1 in a male mouse (oral).15 On the basis of calculated values of the octanol–water coefficient (Kow) by the EPI Suite™ v4.00 program, theoretical acute LD50 values of IMPA were calculated as 3563, 1555, and 348 mg L−1 for fish, daphnes, and green algae, respectively, whereas, the LD50 values of PMPA were lower, at 294, 156, and 63 mg L−1 against fish, daphnes, and green algae, respectively.16 To the best of our knowledge, so far there are no reports on the bioaccumulation of PMPA or its analogues in organisms.
Gas chromatography (GC) and liquid chromatography (LC) combined with mass spectrometry (MS) are established techniques for the determination of PMPA. In consideration of the considerable hydrophilic nature of PMPA, the GC method was developed for the directed determination of PMPA or its derivatizes employing trimethyloxonium tetrafluoroborate.16,17 However, the limit of quantification (LOQ) was considered dissatisfactory in terms of the sensitivity and the derivatization was cumbersome. On account of their high sensitivity, LC-MS methods are the most commonly used for PMPA determination in biological and environmental samples. For the verification of nerve agent exposure, hair, nail, blood, and urine samples were assessed for the detection of PMPA and other nerve agent hydrolysis products by LC-MS/MS, and the limits of detection (LODs) for PMPA were found to be 0.15 μg kg−1, 0.3 μg kg−1, 1.0 ng mL−1, and 0.03 ng mL−1, respectively.18–21 For monitoring their degree and site of contamination in the environment, tap water and soil samples were assessed for the detection of PMPA and other nerve agent hydrolysis products, and the limits of detection (LODs) for PMPA were found to be 0.01 ng mL−1 and 1.0 ng g−1.22,23
Zebrafish (Danio rerio) is a small tropical freshwater bony fish, in which the genes of zebrafish are highly similar to human genes.24–26 Besides, zebrafish has the advantages of a short generation cycle, easy feeding, low feeding cost, and low space requirements.27,28 Therefore, zebrafish is a widely used model organism applied in studies of biological processes with environmental and medical relevance and as recommended by the Organization for Economic Co-operation and Development (OECD).29,30
In the present study, the behavior variations of zebrafish were first researched following soman exposure for a week. Then, the zebrafish were exposed to PMPA with three different concentrations for a week and histological sections of the zebrafish tissues from the liver, gonad, intestine, and brain were examined to investigate the toxicity of PMPA. An LC-MS/MS method was established and validated. After soman exposure, the contents of PMPA in whole zebrafish and the tissues were finally determined by the LC-MS/MS method.
2 Materials and methods
2.1 Chemicals, reagents, and zebrafish culture
Soman (98% purity) was obtained from the Chinese Laboratory of Analytical Chemistry (Beijing, China). Pinacolyl methylphosphonate acid (PMPA, >97% purity) and isopropyl methylphosphonate acid (IMPA, internal standard, >97% purity) were purchased from Aldrich (Milwaukee, WI, USA). The structures of those standards are shown in Fig. S1.† 2-[(Dimethylamino)methyl]phenol (2-DMAMP, containing phenol, >70% purity), ammonium formate (98% purity), and methyl tert-butyl ether (MTBE, 99.9% purity) were obtained from Sigma-Aldrich (St. Louis, MO, USA). Chromatographic-grade acetonitrile and butyl alcohol were obtained from Merck (Darmstadt, Germany). Ultrapure water (18.2 MΩ cm resistivity) was generated in-house using a Milli-Q System from Millipore (Bedford, MA, USA). The other reagents were obtained from commercial sources and were analytical grade.
Zebrafish (Danio rerio) were purchased from a local aquarium (Huamao Longchang Aquarium Business Department) in Beijing, P. R. China, with a body weight from 0.25 to 0.55 g. They were allowed to acclimate to the laboratory conditions in glass aquaria filled with dechlorinated water for 2 weeks prior to the toxicity or exposure experiments. All the zebrafish feeding and experiments were conducted following the OECD Test Guideline 305 (OECD TG305).31
2.2 Acute LC50 experiments with soman
During the acute toxicity experiments with soman, 10 zebrafish were placed into glass aquaria filled with 4 L oxygenated water with air-bubbling. Each aquarium was exposed to a range of concentrations of soman (0, 1.20, 1.40, 1.82, 2.20, 2.37, 3.08 μg L−1) diluted in water. Zebrafish were not fed and the mortality of the fish due to exposure was recorded for up to 24 h at 6 h intervals to obtain the LC50 values of soman on the zebrafish. For assessing the influence of the solvent, the fish were exposed to acetonitrile (2 μL L−1) alone as the solvent control group under similar conditions to the treatment group.
2.3 Swimming behavior tests
After a week of exposure, 5 zebrafish were transferred from the exposure room as the exposure group. Besides, 5 untreated zebrafish were considered as the control group. The zebrafish were acclimated to the testing room for 1.5 h prior to swimming. Each zebrafish of those zebrafish groups was individually placed in a glass beaker containing 500 mL of natural water. The detection method and data analysis were similar to previously described methods.32 Swimming behavior tests of the zebrafish were investigated in the behavioral observation system EthoVision XT 15 (Noldus, Wageningen, Netherlands). The swimming behavior was recorded using a video camera (Basler acA1300-60 gm NIR). Then the tracking videos were analyzed using EthoVision XT software to assess several indicators of mobility, including distance moved, velocity, body fill of mobility, mobility state, and movement.
2.4 Histopathologic study
For the toxicity experiments to PMPA, 10 zebrafish were placed into glass aquaria and exposed to a range of concentrations of PMPA (0, 20, 50, 100 μg L−1) diluted in water. After a 1 week period, the zebrafish were collected and dissected for obtaining the tissue samples. Those tissues (intestine, liver, muscle, gonad, brain) were fixed using 4% paraformaldehyde solution in phosphate buffer solution (PBS) overnight at 4 °C, washed in PBS, and equilibrated in 30% sucrose/PBS overnight at 4 °C. Sections of 7 μm thickness were prepared from paraffin blocks using a rotary microtome and then stained with hematoxylin and eosin (H&E). Histopathological changes were examined under a Nikon Eclipse Bio microscope.
2.5 Bioaccumulation experiment
In the bioaccumulation experiments, doses of soman selected for use in this investigation were based on the results from the 24 h LC50 study. Ten zebrafish were placed into glass aquaria filled with 4 L oxygenated water with air-bubbling. Each aquarium was randomly exposed to a range of concentrations of soman (0, 0.1, 0.5 μg L−1) diluted in water. Each test concentrations was replicated five times. The exposure solution was totally changed every 24 h in the exposure period. To investigate the soman degradation rate in the exposure solution, aquaria were exposed to soman (0.5 μg L−1) and none of the zebrafish was placed into glass aquaria. The exposure solutions were collected up to 24 h from each aquarium at 2 h intervals and the exposure solution concentrations of soman were detected using UHPLC-MS/MS. The solution was centrifuged at 12
000 rpm for 7 min and the supernatant was stored at 20 °C before analysis.
Following a 1 week period, the zebrafish were cleaned three times with purified water and placed on ice for a 1 week period. Then, eight zebrafish were dissected for obtaining the tissue samples. The other two zebrafish had their tissue samples collected and accurately weighed. These samples were transferred to 1.5 mL tubes and homogenized with acetonitrile (1
:
2, w/v) in a bullet blender (Bullet Blender Storm BBY24M, Next Advance, USA). The homogenized tissues were vortexed for 5 min. After, the homogenized tissues were centrifuged at 12
000 rpm for 7 min, and then the supernatant was transferred to another tube and stored at −20 °C before analysis. The internal standard (IMPA) was added to the supernatant at an IMPA concentration of 20 ng g−1.
2.6 Sample preparations of the standards and quality control
All the standards (soman, PMPA, IMPA) were accurately weighed, dissolved in acetonitrile and made up as stock solutions with concentrations all at 1.0 mg mL−1. Standard solutions of PMPA were obtained as 400, 160, 64, 25.6, and 10.24 ng mL−1 by diluting the stock solution with acetonitrile. Similarly, standard solutions of IMPA were obtained at 100 ng mL−1 by diluting the stock solution with acetonitrile. The standard solutions of PMPA and IMPA were respectively spiked with 5 μL of the supernatant (90 μL) of the blank whole zebrafish and tissues to prepare the calibration solutions. The supernatants of the blank whole zebrafish and tissues were acquired as describe in Section 2.4 regarding the bioaccumulation experiments. Therefore, the concentrations of the calibration solutions were 40.0, 16.0, 6.4, 2.6, and 1.0 ng g−1 for PMPA and all the calibration solutions contained IMPA with a concentration of 20 ng g−1. At the same time, quality control (QC) samples of low, medium, and high levels were prepared at 2.0, 8.0, and 32.0 ng g−1 to investigate the specificity, the recovery, accuracy, precision, and stability. Meanwhile, the QC samples contained IMPA with a concentration of 20 ng g−1. All the samples, including stock solutions, standards solutions, calibration solutions, and QC samples, were stored at −20 °C.
2.7 UHPLC-MS/MS analysis
The concentrations of PMPA and soman in the exposure solutions and zebrafish tissues were analyzed by a Agilent 6470 UHPLC-MS/MS. Analyte separations were carried out using an Agilent 1290 UHPLC system (Santa Clara, CA, USA), equipped with an Agilent Poroshell 120 HILIC-Z column (2.1 × 100 mm, 2.7 μm). The injection volume was 2 μL. Gradient elution was achieved using a mobile phase consisting of water (A) with 2 mM ammonium formate and acetonitrile (B) with 0.05% ammonia in each, and performed with the following schedule: 95–90% of B at 0–7.0 min, 90–86% of B at 7.0–7.5 min, 86–50% of B at 7.5–8.5 min, 50–50% of B at 8.5–14.0 min, and 50–95% B at 14–16 min. The column oven was held at 28 °C, and the flow rate was 0.35 mL min−1.
Quantitative analysis was performed on an Agilent 6470 QQQ mass spectrometer (Santa Clara, CA, USA). For the ion-source parameters, the sheath gas temperature and flow were set at 320 °C and 11 L min−1, respectively; the capillary voltage was 4500 V, and the nozzle voltage was 300 V; the nitrogen drying gas temperature and flow rate were 250 °C and 7 L min−1; and the nebulizer pressure was 30 psi. Data were acquired in MRM mode via negative electrospray ionization, and the MRM parameters, such as the precursor, transitions, and retention times of the standards, are shown in Table S1.†
2.8 Method validation and data analysis
To investigate the specificity and selectivity, samples of the blank matrix, blank matrix with the PMPA standard, and actual zebrafish were prepared and comparatively analyzed for the presence of interfering MRM transitions. The blank matrix from zebrafish considered 6 different zebrafish. The samples of the blank matrix with PMPA standard were obtained from a PMPA standard spiked blank matrix within 3 different zebrafish. The samples of actual zebrafish were actual samples after soman exposure within 3 different zebrafish.
In the range of 1.0–40.0 ng g−1, a calibration curve was established by analysis of a series of calibration solutions in duplicate over 5 runs. LOD and LOQ were investigated within 3 sources in duplicate over 3 runs. The LOD was defined by the peak shape (S/N > 3), and ion ratio (deviate within ±20%) at the LOD. The LOQ was defined by the peak shape (S/N > 10), and relative standard deviation (RSD ≤ 20%).
The accuracy and precision were assessed by intra- and inter-day variations. Within the same day and three consecutive days, 6 replicates of QCs at three different levels (low, medium, high) in duplicate over 5 runs were tested for intra-day variations and inter-day variations, respectively. Matrix effects and recovery were estimated by QCs at three different levels (low, medium, high) within 6 replicates using three sets of samples: (A) standard solution directly spiked into the mobile phase; (B) standard solution spiked into post-extraction; (C) standard solution spiked into pre-extraction. Matrix effects (%) were calculated as the peak area ratio of sets B to sets A and recovery (%) was calculated as the peak area ratio of sets C to sets B.
Stability was assessed by QCs at three different levels (low, medium, high) under different storage conditions, including short-term storage at 23 °C for 24 h, long-term storage at −40 °C for 30 days, and three freeze–thaw cycles. The concentrations of those sample were measured and combined with the initial concentrations to calculate the accuracy and precision.
UHPLC-MS/MS data were collected through an Agilent MassHunter Workstation Data Acquisition and processed by QQQ Quantitative Analysis software (Santa Clara, CA, USA). The MRM parameters of the soman, PMPA, and IMPA were optimized by using Agilent MassHunter Optimizer software. Additionally, the ion-source parameters were optimized by Agilent MassHunter Source Optimizer software.
2.9 Live subject statement
The authors state that all experiments were performed in compliance with the relevant laws and institutional guidelines of the People's Republic of China regarding animal experiments. The research ethics committee of the State Key Laboratory of NBC Protection for Civilians approved the experiments on live subjects (Code of Ethics: LAE-2023-03-001).
3 Results
3.1 Chronic toxicity test of PMPA
Histological analysis was used to investigate chronic toxicity of PMPA on tissue samples of the liver, gonad, intestine, and brain (Fig. 1). During this part of the experiment, no zebrafish died. The control fish swam normally without any abnormal signs. In the intestinal sections, the experimental groups were examined at three different levels. The epithelial cells of normal intestinal tissue were closely arranged (a1), and the epithelial cell boundaries were blurred after exposure to all exposure concentrations (a2 and a3), while the epithelial cell boundaries were blurred after exposure to 100 μg L−1 (a4), and the villi were noted to be irregular and the intestinal lumen was dilated (b). In the hepatic section, vacuolization began at 50 μg L−1 (c1 and c2) and the sinusoids spaces were also observed from 100 μg L−1 (d). In the gonad sections of female, an increase in the number of atretic oocytes was observed from 20 μg L−1 for female specimens (e1–e3). Lastly, histopathological alterations were not found in the brain section. However, in this part, histopathologic lesions were found in the liver, gonad, and intestine.
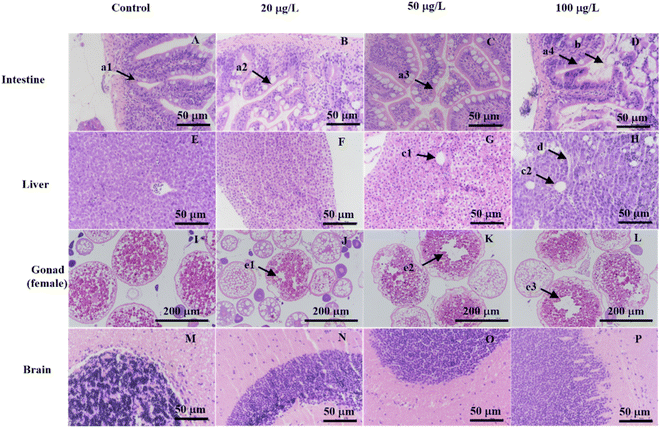 |
| Fig. 1 Histopathologic lesions from zebrafish liver, gonad, intestine, and brain samples following exposure to a range of concentrations of PMPA (0, 20, 50, 100 μg L−1). | |
3.2 Acute LC50 of soman on zebrafish
The mortality was recorded at up to 24 h in the experimental group and no mortality was found in the control group. Statistical analysis was performed using GraphPad Program 8 (GraphPad Software, San Diego, USA) and the results gave the median lethal concentration (LC50) values and 95% confidence interval using the profile likelihood for soman on zebrafish. The 24 h LC50 value of soman on zebrafish was found to be 1.93 μg L−1 with a 95% confidence interval of 1.76–2.11 μg L−1 (Fig. 2). These results revealed that soman was an extremely toxic substance to zebrafish.
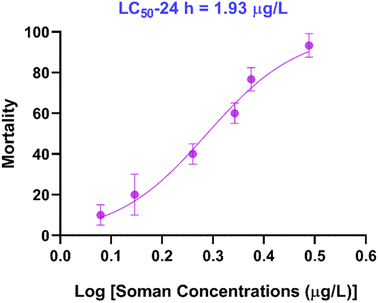 |
| Fig. 2 LC50-24 h plot for soman effect on zebrafish. | |
3.3 Swimming behavior response
EthoVision XT 15 recorded the changes of the zebrafish body contour during swimming behavior tests and was used to calculate the mobility parameters to evaluate excitability. The distance moved and velocity were significantly lower compared with the control group after exposure to soman at 0.1 μg L−1 for 1 week (Fig. 3A and B). Moreover, the body signs for mobility, mobility state, and movement were significantly lower compared with the control group after exposure to soman at 0.1 μg L−1 for 1 week (Fig. 3C–E). These findings suggested that zebrafish were depressed after exposure to soman at 0.1 μg L−1 for 1 week.
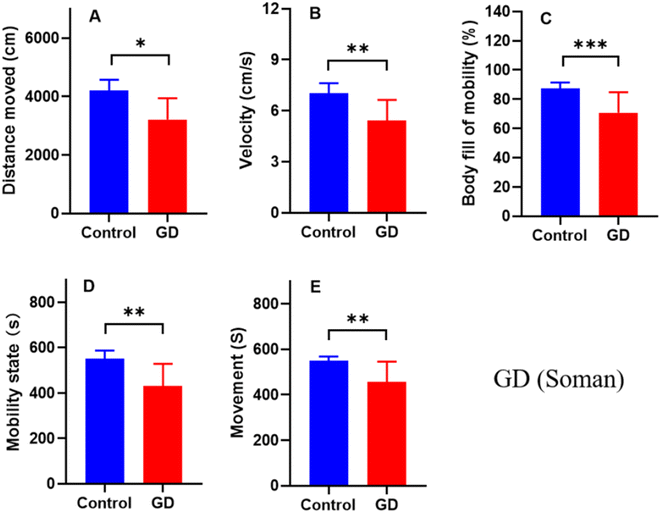 |
| Fig. 3 The swimming behavioral response of zebrafish after a week of soman exposure at 0.1 mg L−1 (n = 5) compared with the control group (n = 5), including distance moved (A), velocity (B), body bill of mobility (C), mobility state (D), movement (E), (*p < 0.05, **p < 0.01, ***p < 0.001). | |
3.4 Development and validation of the quantitative method
To reduce the complexity of sample pretreatment and the loss of analytes, PMPA was directly extracted from the zebrafish and tissue. Parameters for the LC were first optimized and developed to obtain a satisfactory method and to meet quantitative requirements, including the use of an HILIC column. Considering its similar chemical structure, IMPA was selected as the internal standard. The TICs of the QC samples were shown at three different levels (Fig. S2†).
Validation of the quantitative method was performed in accordance with USA Food and Drug Administration (US-FDA) bioanalytical method validation guidance.33 Based on the established quantitative method, there was no interference peak observed in the blank matrix of the zebrafish and tissues and the peak shapes of PMPA could be specifically identified from the actual zebrafish (Fig. S3 and S4†). The correlation coefficient of the calibration curve exceeded 0.999 in the range of 1.0–40.0 ng g−1 (Fig. S5†). The LOD and LOQ of the PMPA were 0.1 ng g−1 and 1.0 ng g−1 in zebrafish and tissue samples and the sensitivity was considered sufficient for quantification in biological samples (Fig. S6†). The intra- and inter-day precision were assessed at three levels of QCs, and finally the RSD and RE were obtained (Table S2†), respectively. The maximum RSDs of the intra- and inter-day precision were 11.5% and 10.6%, respectively. Meanwhile, the intra- and inter-day accuracy was within ±12.6% for all analytes. Matrix effects of the QCs at three different levels (low, medium, high) ranged from 77.3% to 89.2%, respectively. Additionally, recovery of the QCs at three different levels (low, medium, high) ranged from 87.3% to 115.5%, respectively (Table S3†). PMPA was found to be stable under each storage condition assessed: short-term storage at 23 °C for 24 h, long-term storage at −40 °C for 30 days, and three freeze–thaw cycles (Table S4†); whereby the concentration of PMPA deviated less than ±15%. Above all, all the results were within the criteria for acceptability with satisfactory specificity, selectivity, linearity, precision, accuracy, recovery, and stability.
3.5 Soman concentration after exposure
Concentrations of soman in the exposure solutions were determined, as described in ref. 34. The concentration of soman decayed from 0.46 μg L−1 to 0.07 μg L−1 in the 24 h exposure solution. The data were entered and the curves fitted after selecting XY analysis, linear regression, the exponential equations pane, and one phase decay in GraphPad Prism 8 software (Fig. 4). The R squared of the curves was 0.994 and the t1/2 of soman degradation was found to be 6.9 h in the exposure solution.
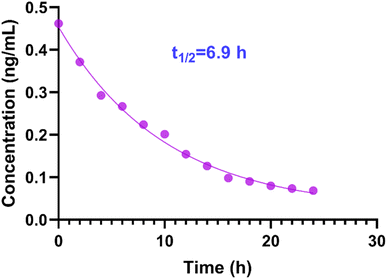 |
| Fig. 4 Concentration of soman in 24 h exposure solution at 0.5 μg L−1. | |
3.6 Concentration of PMPA in zebrafish after exposure
The established method was applied for the determination of PMPA in whole zebrafish and the tissue samples after 1 week of soman exposure (n = 5). Fig. S7† shows the chromatograms corresponding to PMPA and IMPA detected in the whole zebrafish. The whole zebrafish samples were determined and the concentration values were in the linear range 1.0–40.0 ng g−1. The relative standard deviation of the QC samples was less than 15% in the running samples. The concentration of whole zebrafish sample was 12.52 ng g−1 after exposure to soman at 0.5 μg L−1 and 1.21 ng g−1 after exposure to soman at 0.1 μg L−1. This indicated that bioaccumulation occurred in the whole body of the zebrafish. Similarly, the concentrations in the tissue samples (muscle, liver, gonad, intestine, and brain) were found to be 1.03% ± 8.5%, 3.98% ± 4.9%, 2.50% ± 7.6%, 5.69% ± 7.4%, and 2.41% ± 6.9% ng g−1 respectively after exposure to soman at 0.1 μg L−1 (Fig. 5).
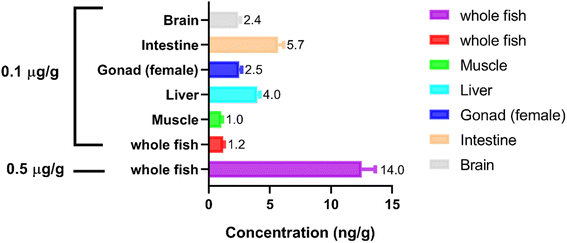 |
| Fig. 5 Concentration of PMPA in whole zebrafish and tissue samples after 1 week of soman exposure at 0.1 and 0.5 μg L−1 (n = 5). | |
4 Discussion
Dyskinesia was first found on zebrafish after exposure to soman at 0.1 μg L−1 for a 1 week period. Soman is susceptible to degradation in the environment, and the main degradation product is pinacolyl methylphosphonic acid (PMPA). Meanwhile, soman can also be easily metabolized into PMPA in vivo.15 This implies that PMPA is likely to have toxic effects in zebrafish. Thus, histological sections of zebrafish tissues were examined after zebrafish were exposed to PMPA with three different concentration at 20, 50, and 100 μg L−1 for 1 week. Histopathologic lesions were found in the liver, gonad, and intestine at 50 and 100 μg L−1, confirming that PMPA has toxic effects in zebrafish; notwithstanding that IMPA (PMPA analogues) displayed a relatively low acute toxicity.35 In view of the fact that PMPA is more liposoluble than IMPA in vivo and as soman exposure lasts a long time, the difference between IMPA and PMPA was probably a consequence of PMPA bioaccumulating in organisms. After exposure to soman at 0.1 μg L−1 for 1 week, the concentration of PMPA in the whole zebrafish samples, muscle, liver, gonad, intestine, and brain tissues samples were lastly determined as 1.21, 1.03, 3.98, 2.50, 5.69, and 2.41 ng g−1 respectively. Moreover, the concentration of PMPA in the whole zebrafish samples was 12.52 ng g−1 after exposure to soman at 0.5 μg L−1 for 1 week. PMPA could more easily accumulate in the intestine and liver. This may result from lesions in the liver and intestine, which hampered PMPA metabolism and excretion. The results proved for the first time that PMPA bioaccumulates in organisms. Although PMPA may be less toxic, it can more easily accumulate in aquatic organisms under long-term soman exposure to cause PMPA poisoning. In summary, we put forward that PMPA could pose an environmental risk after soman exposure to a water environment.
The LOQ of PMPA in the quantitative method was 1.0 ng g−1, which means it was not sensitive enough to determine PMPA concentration after soman exposure at 0.5 μg L−1; thereby after soman exposure, the PMPA concentration was not measured in the soman exposure solution. Besides, the concentration of soman exposure was approximately defined at 0.25 LC50 and 0.05 LC50, i.e., 0.5 and 0.1 μg L−1, in the bioaccumulation experiments, so that the PMPA concentration could be determined in zebrafish. The hepatic microsomal esterase activity was enhanced following soman exposure, which could impact the metabolization of PMPA.36 Therefore, in order to acquire reliable results in the bioaccumulation of PMPA after soman exposure, zebrafish were exposed to soman instead of PMPA.
In the bioaccumulation part, we aimed to preliminary determine if PMPA could pose a bioaccumulation risk in zebrafish after soman exposure to a water environment. Therefore, the bioaccumulation research did not strictly follow the guidance from OECD TG305.31 For example, the uptake phase is usually run for 28 days instead of 1 week in the aqueous exposure tests. In the future, more work should be done in the bioaccumulation study to closely follow the guidance for bioaccumulation in fish, such as a study on the depuration phase, BCF.
5 Conclusion
In this study, PMPA was proved to be a toxicant to zebrafish. PMPA bioaccumulation was preliminary found in whole zebrafish and tissues for the first time following soman exposure to a water environment and through us establishing a method to determine PMPA in zebrafish and tissues. Consequently, there is not only a need to pay attention to the harm of soman to the ecological environment, but also the harm of its degradation product PMPA should not be ignored when soman is exposed to the environment.
Conflicts of interest
The author(s) declared no potential conflicts of interest with respect to the research, authorship, and/or publication of this article.
Acknowledgements
The author(s) received financial support by State Key Laboratory of NBC Protection for Civilian Found (File No. SKLNBC2021-12).
References
- J. Hart, Looking Back: The Continuing Legacy of Old and Abandoned Chemical Weapons, Arms Control Today, 2008, pp. 21–25 Search PubMed.
- A. T. Tu, Toxin Rev., 2011, 30, 1–5 CrossRef.
- G. Glasby, Sci. Total Environ., 1997, 206, 267–273 CrossRef CAS PubMed.
- M. Greenberg, K. Sexton and D. Vearrier, Clin. Toxicol., 2016, 54, 79–91 CrossRef CAS PubMed.
- V. Aroniadou-Anderjaska, J. P. Apland, T. H. Figueiredo, M. D. A. Furtado and M. F. Braga, Neuropharmacology, 2020, 181, 108298 CrossRef CAS PubMed.
- M. Moshiri, E. Darchini-Maragheh and M. Balali-Mood, DARU J. Pharm. Sci., 2012, 20, 1–24 CrossRef PubMed.
- K. S. Bakshi, S. N. Pang and R. Snyder, J. Toxicol. Environ. Health, 2000, 59, 417–438 CrossRef.
- H. E. Brezenoff, J. McGee and V. Knight, Acta Pharmacol. Toxicol., 1984, 55, 270–277 CrossRef CAS PubMed.
- D. Rickett, J. Glenn and E. Beers, Neurotoxicology, 1986, 7, 225–236 CAS.
- T. Pazdernik, R. Cross, S. Nelson, F. Samson and J. McDonough Jr, Neurotoxicology, 1983, 4, 27–34 CAS.
- J. A. Koenig, T. L. Dao, R. K. Kan and T. M. Shih, Ann. N. Y. Acad. Sci., 2016, 1374, 68–77 CrossRef CAS PubMed.
- M. Haley, C. Kurnas and J. Ware, Toxicity of Hydrolyzed Chemical Agents to Aquatic Organisms, United States Army Edgewood Research, Development and Engineering Center, Aberdeen Proving Ground, 1997 Search PubMed.
- J. J. Pancrazio, E. W. Keefer, W. Ma, D. A. Stenger and G. W. Gross, Neurotoxicology, 2001, 22, 393–400 CrossRef CAS PubMed.
- H. Sanderson, P. Fauser, M. Thomsen and P. B. Sørensen, J. Hazard. Mater., 2007, 148, 210–215 CrossRef CAS PubMed.
- N. B. Munro, S. S. Talmage, G. D. Griffin, L. C. Waters, A. P. Watson, J. F. King and V. Hauschild, Environ. Health Perspect., 1999, 107, 933–974 CrossRef CAS PubMed.
- I. Połeć, A. Kiełczewska, L. Konopski, G. Oleksa, H. N. Krukowska and J. Legocki, Cent. Eur. J. Chem., 2010, 8, 1251–1265 Search PubMed.
- C. A. Valdez, R. N. Leif and A. Alcaraz, Anal. Chim. Acta, 2016, 933, 134–143 CrossRef CAS PubMed.
- A. S. Appel, J. H. McDonough, J. D. McMonagle and B. A. Logue, Anal. Chem., 2016, 88, 6523–6530 CrossRef CAS PubMed.
- L. Y. Aviram, M. Magen, S. Chapman, A. N. Cohen, S. Lazar and S. Dagan, J. Chromatogr. B: Anal. Technol. Biomed. Life Sci., 2018, 1093–1094, 60–65 CrossRef PubMed.
- A. S. Appel and B. A. Logue, J. Chromatogr. B: Anal. Technol. Biomed. Life Sci., 2016, 1031, 116–122 CrossRef CAS PubMed.
- D. B. Mawhinney, E. I. Hamelin, R. Fraser, S. S. Silva, A. J. Pavlopoulos and R. J. Kobelski, J. Chromatogr. B: Anal. Technol. Biomed. Life Sci., 2007, 852, 235–243 CrossRef CAS PubMed.
- B. T. Røen, S. R. Sellevåg and E. Lundanes, Anal. Chim. Acta, 2013, 761, 109–116 CrossRef PubMed.
- S. Dowling, E. M. McBride, J. McKenna, T. Glaros and N. E. Manicke, Forensic Chem., 2020, 17, 100206 CrossRef CAS.
- K. Howe, M. D. Clark, C. F. Torroja, J. Torrance, C. Berthelot, M. Muffato, J. E. Collins, S. Humphray, K. McLaren and L. Matthews, Nature, 2013, 496, 498–503 CrossRef CAS PubMed.
- J.-P. Levraud, L. Jouneau, V. Briolat, V. Laghi and P. Boudinot, J. Immunol., 2019, 203, 3361–3373 CrossRef CAS PubMed.
- W. B. Barbazuk, I. Korf, C. Kadavi, J. Heyen, S. Tate, E. Wun, J. A. Bedell, J. D. McPherson and S. L. Johnson, Genome Res., 2000, 10, 1351–1358 CrossRef CAS PubMed.
- B. Tsang, H. Zahid, R. Ansari, R. C. Lee, A. Partap and R. Gerlai, Zebrafish, 2017, 14, 561–573 CrossRef PubMed.
- A. Nasiadka and M. D. Clark, ILAR J., 2012, 53, 161–168 CrossRef CAS PubMed.
- M. Faria, N. Garcia-Reyero, F. Padrós, P. J. Babin, D. Sebastián, J. Cachot, E. Prats, M. Arick, E. Rial and A. Knoll-Gellida, Sci. Rep., 2015, 5, 1–16 Search PubMed.
- G. Bowley, E. Kugler, R. Wilkinson, A. Lawrie, F. van Eeden, T. J. Chico, P. C. Evans, E. S. Noël and J. Serbanovic-Canic, Br. J. Pharmacol., 2022, 179, 900–917 CrossRef CAS PubMed.
- OECD, Test No. 305: Bioaccumulation in Fish: Aqueous and Dietary Exposure, 2012 Search PubMed.
- L. R. Hansen and P. Roslev, Aquat. Toxicol., 2016, 179, 36–43 CrossRef CAS PubMed.
- U. S. Food and Drug Administration, Department of Health and Human Services and Center for Drug Evaluation and Research (CDER), Guidance for Industry Bioanalytical Method Validation, 2018 Search PubMed.
- A. Weissberg, M. Madmon, M. Elgarisi and S. Dagan, J. Chromatogr. A, 2018, 1577, 24–30 CrossRef CAS PubMed.
- C. C. Green, S. E. Lochmann and D. L. Straus, J. Toxicol. Environ. Health, Part A, 2005, 68, 141–149 CrossRef CAS PubMed.
- W. E. Luttrell and M. C. Castle, Biochem. Pharmacol., 1993, 46, 2083–2092 CrossRef CAS PubMed.
|
This journal is © The Royal Society of Chemistry 2023 |
Click here to see how this site uses Cookies. View our privacy policy here.