DOI:
10.1039/D3RA00716B
(Paper)
RSC Adv., 2023,
13, 8535-8539
CaGdF5 based heterogeneous core@shell upconversion nanoparticles for sensitive temperature measurement†
Received
2nd February 2023
, Accepted 5th March 2023
First published on 14th March 2023
Abstract
Lanthanide-doped upconversion nanoparticles (UCNPs) have attracted great attention in temperature sensing because of their widespread thermal quenching effect (TQE), a phenomenon in which luminescence intensity decreases as the temperature increases. However, enhancing the TQE of activated ions without changing the dopants or the host is still challenging. Herein, Yb3+ and Er3+ codoped UCNPs in a cubic CaGdF5 host were synthesized by a coprecipitation method for optical temperature sensing. Compared with the homogeneous shell (CaGdF5), those heterogeneous (CaF2) shelled UCNPs exhibited stronger upconversion luminescence (UCL) due to the significantly reduced multiphonon nonradiative relaxation. Further, we investigated the effects of homogeneous and heterogeneous shells on TQE. The relationship between the intensity ratio of the green emission bands of Er3+ ions (2H11/2 → 4I15/2 and 4S3/2 → 4I15/2) and temperature are obtained for these two core@shell UCNPs. The results demonstrated that the UCNPs with CaF2 shells are more sensitive to temperature in the 200–300 K. The maximum thermal sensitivity of CaGdF5:Yb,Er@CaF2 could reach 2.2% K−1 at 200 K. These results indicate that the heterogeneous core@shell UCNPs are promising for use as optical temperature sensors.
1 Introduction
Optical temperature sensing based on the luminescence intensity ratio (LIR) has been used in the past decades since it allows for noninvasive thermal detection.1–3 This sensing technique monitors temperature-dependent changes at specific thermally coupled energy levels by measuring emission intensity ratios. Lanthanide-doped UCNPs can convert long-wavelength photons to short-wavelength photons, and are considered promising probes in optical thermometry with a fast response, and great resolution and sensitivity.4–7 It has been shown that the LIR of the thermally coupled two green bands of Er3+ ions varies as a function of temperature. Consequently, Er3+-doped luminescent materials have been widely used as probes for thermal sensing applications.8–13
Besides, it is crucial to choose an appropriate host material with low crystal field symmetry14 and low phonon energy15 that provide an efficient luminescence signal for practical applications. On the one hand, the forbiddance of the 4f–4f electronic-dipole transitions of rare-earth ions can be broken by the crystal field symmetry of the host matrix. On the other hand, the host materials also require low phonon energy, which prevents luminescence quenching due to the nonirradiation relaxation. Lanthanide fluorides (LaF3, YF3 and GdF3) have been taken as promising materials for upconversion luminescence because of low phonon energy. Unfortunately, these materials suffered from the high crystal field symmetry of orthorhombic phase, resulting in limited upconversion efficiencies.16–18 Therefore, research interest turns to alkaline or alkaline-earth rare-earth fluoride (MReF) host lattices showing more efficient upconversion luminescence, such as hexagonal NaLnF4, and cubic BaYF5 NPs.19–26 Recently, SrLuF host lattices have provided more efficient upconversion than β-NaGdF4 with similar nanoparticle size.27 Lanthanide-doped MReF UCNPs should be the promising host material for optical temperature sensing applications. In addition to composition optimization, the core@shell structure can also improve temperature detection sensitivity. Liu et al. reported core@multishell UCNPs with an enhanced thermal sensitivity three times higher than core-only NPs.28 For preparing core@shell UCNPs, epitaxial growth is preferred for constructing the same or similar crystal structure for core and shell counterparts, such as NaYF4@NaGdF4. Nevertheless, the influences of shell composition on the temperature sensitivity of UCNPs have not been thoroughly investigated until now.
Here, we developed a thermal decomposition method to synthesize ultra-small CaGdF nanoparticles (NPs) with cubic phase. Sub-5 nm Yb3+ and Er3+ codoped CaGdF UCNPs were synthesized with an optimized Ca2+/Gd3+ molar ratio of 1
:
1. Our alkaline-earth-based UCNPs doped with Yb3+ and Er3+ show mostly green and red upconversion emissions under 980 nm laser illumination. The dominant upconversion mechanism is based on Förster resonant energy transfer (FRET), which depends on the inverse distance between the dopants. What's more, we studied the influence of CaGdF and CaF2 shells on optical properties. Due to less non-irradiation relaxation, the core@shell CaGdF5@CaF2 NPs (CGF@CF) performed higher efficient luminescence than CaGdF5@CaGdF5 NPs (CGF@CGF). Furthermore, we evaluated the thermal sensing performance of CGF@CF and CGF@CGF in the temperature range from 200 K to 300 K. The LIR of thermally coupled two green bands of Er3+ ions in CGF@CF is more sensitive to temperature variation, indicating the potential for these NPs as thermometers.
2 Experimental
2.1 Reagents
Ln(CH3COO)3·xH2O (>99%), Ln2O3 (Ln: Gd, Yb, Er, > 99%), Ca(CH3COO)2, CaO, NH4F (>98%), oleic acid (OA, 90%), 1-octadecene (ODE, 90%), trifluoroacetic acid (99%), ethanol and cyclohexane were purchased from Sigma-Aldrich and used without further purification.
2.2 Synthesis of core CaxGdyF(2x+3y):20% Yb,2% Er NPs
The synthesis of monodisperse CaxGdyF(2x+3y):20% Yb,2% Er nanocrystals was slightly modified from the previously reported method.27 x mmol of Ca(CH3COO)2, 0.78y mmol of Gd(CH3COO)3·xH2O, 0.2y mmol of Yb(CH3COO)3·xH2O, and 0.02y mmol of Er(CH3COO)3·xH2O (x
:
y = 0
:
1, 1
:
1, 2
:
1), 8 mL of oleic acid and 8 mL of 1-octadecene were added to a 100 mL flask under stirring. The solution was heated to 120 °C and then kept for 1 h under vacuum and then cooled to room temperature. After (2x + 3y) mmol of NH4F was added, the solution was heated to 120 °C and kept for 1 h under a vacuum. Finally, the solution became a clear yellow solution. Then the solution was heated to 300 °C under a vacuum for 1 h and then cooled to room temperature. The nanocrystals were precipitated with 10 mL of ethanol, collected after centrifugation, and redispersed in 10 mL of cyclohexane.
2.3 Synthesis of CaGdF5 and CaF2 precursor
To obtain 0.1 mmol per mL CaGdF5 precursor for shell coating, 1 mmol CaO, 0.5 mmol Gd2O3, and 5 mmol trifluoroacetic acid were added to a 100 mL flask and heated to 50 °C under vigorous stirring. After the system became a clear solution, 5 mL of oleic acid and 5 mL of 1-octadecene were added, and the solution was heated to 100 °C for 10 min and then to 156 °C for 30 min. The precursor is finally cooled to room temperature and waiting to use. The CaF2 precursor is obtained by the same method using only CaO instead of CaO and Gd2O3.
2.4 Synthesis of core@shell CaGdF5:20% Yb,2% Er@CaGdF5 NPs (CGF@CGF) and CaGdF5:20% Yb,2% Er@CaF2 NPs (CGF@CF)
To obtain core@shell NPs, a solution of 0.2 mmol core CaGdF5:Yb,Er nanocrystals in 2 mL of cyclohexane was added to the solution of 5 mL of oleic acid and 5 mL of 1-octadecene. Then the solution was kept at 70 °C for 30 min. After the cyclohexane was evaporated, the solution was heated to 300 °C under an argon atmosphere. The shell precursor is injected with a 0.02 mL min−1 rate into the solution. The core@shell nanocrystals were precipitated with 10 mL of ethanol, collected after centrifugation, and redispersed in 10 mL of cyclohexane.
3 Results and discussion
In order to acquire uniform and monodispersed CaLnF host-based UCNPs, the thermal decomposition method was explored. To ensure the successful formation of CaLnF NPs, a set of UCNPs samples (cores) (S1–3) were synthesized by changing the molar ratio of Ca to Gd acetate salts from 0
:
1 to 2
:
1. Transmission electron microscope (TEM) images in Fig. 1a–c show monodisperse and small diameters of 4.5 ± 1.2 nm, 4.6 ± 1.0 nm, and 4.0 ± 1.1 nm for S1, S2, and S3, respectively (Fig. 1d and S1†). From X-ray diffraction (XRD) patterns, the peaks of S1 well agreed with the characteristic peaks of orthorhombic GdF3, and the peaks became broadband due to their relatively small size. As we can see, the morphology of GdF3 NPs is oval, and coincide with their orthorhombic phase. Those XRD peaks of S2 and S3 well agreed with the characteristic peaks of cubic Ca0.55Gd0.3F2, indicating the synthetic CaxGdyF(2x+3y) samples are Ca0.55Gd0.3F2 crystals (denoted as CaGdF). The alkaline-earth rare-earth fluoride (MLnF) host is based on the MF2 crystal structure and every alkaline-earth cation position can be exchanged with rare-earth cations.27 To ensure all the Gd3+ ions were doped in the CaGdF NPs, the Ca2+/Gd3+ ratio was increased from 1
:
1 to 2
:
1. Compared to the GdF3 NPs, the spherical NPs of CaGdF are observed in Fig. 1b and c. The size of S3 is slightly smaller than that of S2, since the extra Ca2+ ions would produce more nuclear and decrease the average particle volume. Fig. S2† shows the energy dispersive spectrometer (EDS) patterns of the CaGdF NPs with Ca2+/Gd3+ molar ratio of 1
:
1, which reveals that the synthesized products are composed of F, Ca, Gd, Yb and Er.
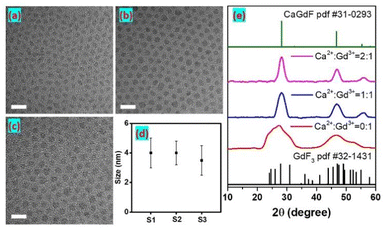 |
| Fig. 1 TEM images of (a) S1, (b) S2, and (c) S3 CaxGdyF(2x+3y) samples with the molar ratio of Ca2+ to Gd3+ (0 : 1, 1 : 1, and 2 : 1, respectively), scale bar: 5 nm; (d) the size and (e) XRD patterns of three CaxGdyF(2x+3y) samples. | |
Next, the UCL performance of as-obtained UCNPs was studied under 980 nm excitation, as seen in Fig. 2a. The Yb3+ as sensitizers strongly absorb the excitation irradiation and transfer the excited energy to the nearby Er3+ emitter to produce upconversion emissions.
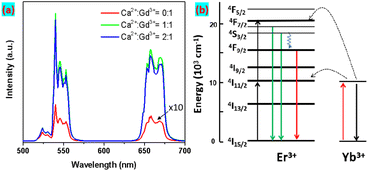 |
| Fig. 2 (a) Upconversion emission spectra of the CaxGdyF(2x+3y) samples under 980 nm excitation (10 W cm−2). (b) Simplified energy level diagrams of Er3+ and Yb3+ ions and proposed energy-transfer mechanisms under 980 nm excitation. | |
The CaGdF:Yb/Er (20/2 mol%) NPs exhibited characteristic emission peaks resulting from 2H11/2, 4S3/2 → 4I15/2 (520–550 nm), and 4F9/2 → 4I15/2 (640–670 nm) transitions of Er3+. Fig. S3† displays the UC integrated intensity bar chart of the samples with fixed Yb/Er (20%/2%) and varying Ca2+/Gd3+ molar ratio. The UC emission intensity drastically increased with the increase of Ca2+/Gd3+ from 0
:
1 to 1
:
1 due to the added Ca3+ ions changing the symmetry of the crystal lattice from orthorhombic GdF3 to cubic CaGdF, and increasing the transition possibility of emitter ions. That is to say, the CaGdF host matrix is better than GdF3 for upconversion emissions. When the Ca2+/Gd3+ molar ratio increased to 2
:
1, the UC emission kept almost the same intensity, indicating that CaGdF:Yb,Er were successfully synthesized with a Ca2+/Gd3+ molar ratio of more than 1
:
1, which is consistent with the XRD results. Compared to CaF2:20% Yb,2% Er NPs, CaGdF:20% Yb,2% Er showed stronger UCL (Fig. S4†), which suggested that the added Gd3+ reduced the symmetry of the CaF2 crystal lattice and increased the transition possibility of Er3+ ions. As we can see, CaGdF:20% Yb,2% Er NPs showed stronger luminescence intensity than NaGdF4:20% Yb,2% Er with similar size, which is consistent with the previous work.29 This may be related to the fact that the value of cation doping concentration in CaGdF is closer to the set optimal value. In general, the smaller the particle size, the lower the UCL efficiency because more quenching defects are generated on their surface as size decreases (specific surface area increases). Therefore, an optically inert shell is needed to prevent the excited state energy from coupling to the surface defects and thus quench the UCL. Next, we studied the UCL performance of homogeneous shells (CGF@CGF) and heteroepitaxial shells (CGF@CF). For comparison, the same core and same moles of injection precursor were employed during the shelling procedure. The TEM and high resolution TEM (HR-TEM) images of core@shell UCNPs are shown in Fig. 3a and b, presenting a diameter of 8.2 ± 0.2 nm for CGF@CGF nanospheres and an edge length of 7.0 ± 0.1 nm for CGF@CF nanocubes. It can be found a typical core–shell structure of CGF@CF NPs because the lanthanide ions (Gd3+) show high contrast (darker) over Ca2+. However, the core–shell structure of CGF@CGF NPs failed due to the same composition in the core and shell layers. Furthermore, to study the shell growth kinetics of these two structures, we took intermediate samples at different precursor injection time points.
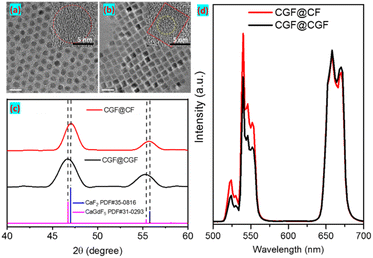 |
| Fig. 3 TEM images of (a) CGF@CGF and (b) CGF@CF NPs, scale bar: 20 nm. Inset: HR-TEM of CGF@CGF and CGF@CF, scale bar 5 nm. (c) XRD patterns of CGF@CGF and (b) CGF@CF NPs. (d) Upconversion emission spectra of the core@shell UCNP samples at 10 W cm−2. | |
As we can see from TEM images (Fig. S5†), morphological change of CGF@CF NPs from spherical to cubic is observed after the second aliquot when the nanoparticle size exceeds approximately 6 nm. For CGF@CGF NPs, however, remain nearly spherical. This morphology could be related to a kinetically dominated shell growth regime due to the larger lattice parameters of CaGdF-based host. Furthermore, XRD analysis revealed the detailed lattice changes caused by different interfacial strains and epitaxial shell growth (Fig. 3c). It can be found that the diffraction peaks of CGF@CGF shift toward lower angles for Gd3+ doping in the host lattice because of the larger lattice parameters according to the Bragg diffraction equation.
Then, we investigated the UCL intensities of these two core@shell samples. To ensure that the UCL intensities of the two kinds of NPs were comparable, we calculated the effective diameter by equating the cubic volume with the spherical volume. For example, the CGF@CF sample with an edge length of 7.0 ± 0.1 nm converts into an effective diameter of 8.1 nm, comparable to spherical CGF@CGF particles. As we can see, upon 980 nm excitation, the upconversion emission was greatly enhanced by coating the NPs with both CaGdF and CaF2 shells. Interestingly, the CGF@CF NPs with CaF2 shell are more efficient in upconversion than those with CaGdF shell and the green to red ratio in CGF@CGF NPs is smaller than CGF@CF NPs (Fig. 3d). The luminescence intensity of energy level of lanthanide ions is expressed as below:
|
 | (1) |
where,
I0 is a constant,
Ar is the radiative transition rate,
Wnr is the multiphonon nonradiative relaxation rate. For UCL of CGF@CGF and CGF@CF NPs, the values of
Ar are the same because the luminescent Er
3+ ions are in the same host lattice and the same core. Therefore, the stronger UC emission of the latter is due to the smaller nonradiative relaxation rate
Wnr, which is showed in
Fig. 2b.
We next investigated the performance of the CGF@shell NPs as a temperature sensor in the 200–300 K range. The emission intensity ratio of the 520 nm (2H11/2 → 4I15/2) to the 540 nm (4S3/2 → 4I15/2) shows a strong temperature dependence because the 2H11/2 and 4S3/2 states of Er3+ ions are thermally coupled. It is observed that the emission band at 520 nm increases with the increasing temperature from 200 to 300 K after being normalized at the peak of 540 nm (Fig. 4a and b). Only several hundred wavenumbers separate these two states, and the thermal equilibrium of states population is determined by the Boltzmann distribution.
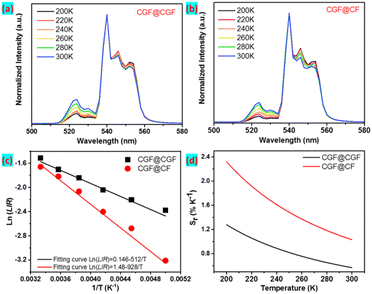 |
| Fig. 4 Normalized emission spectra of (a) CGF@CGF and (b) CGF@CF. core@shell NPs as a function of temperature under 980 nm excitation. (c) LIR of 520 nm and 540 nm as a function of temperature. (d) Relative thermal sensitivity of the CGF@CGF (black curve) and CGF@CF (red curve) NPs in the temperature range of 200–300 K. | |
In our study, the thermometric parameter LIR is defined as the intensity ratio of the two emission peaks, which shows an excellent exponential relationship, described as follows:
|
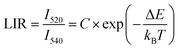 | (2) |
where
C is a constant,
T is the absolute temperature, Δ
E is the energy gap between excited states of
2H
11/2 and
4S
3/2, and
kB is the Boltzmann constant. From
eqn (2), we can see that the LIR is independent of photons since the theoretical model only considers the Boltzmann distribution and radiative transitions between two thermally coupled levels (
2H
11/2 and
4S
3/2). However, the nonradiative relaxation could not be neglected in the upconversion processes at high temperatures due to the increased multiphonon relaxation. From the fitting of ln(LIR)
vs. 1/
T in
Fig. 4c, the linear behavior is obtained with the slope values of −512 and −928 for CGF@CGF and CGF@CF UCNPs, respectively. It indicates that the temperature increase has a stronger influence on the UCL of CGF@CF than that of CGF@CGF. This consequent can be supported by the lifetime of upconversion emission in Fig. S6.
† As we can see, the lifetime of upconversion emission at 520 nm or 540 nm for CGF@CGF only changed slightly while the lifetime for CGF@CF changed a lot when the temperature increased from 200 K to 300 K. Thus, the CGF@CF nanocrystals provide a luminescent probe for radiometric thermometry. The XRD pattern (
Fig. 3c) revealed that the crystal plane spacing of the CaF
2 lattice was smaller than that of CaGdF with the same crystal plane index, which was in agreement with their difference in lattice constants (CaF
2:
a = 5.46 Å, CaGdF:
a = 5.50 Å). Besides, after coating with a CaF
2 shell, the peaks of CGF@CF NPs had a lightly right shift compared to those of CGF@CGF NPs. It means that there is noticeable lattice distortion at the interface of CaGdF@CaF
2. That means the interface lattice distortion can benefit the process of sensing temperature. A similar result has been reported in NaGdF
4@NaYF
4 heterojunctions.
30
Based on the ratiometric thermometer parameter, the relative sensitivity (Sr) was calculated by eqn (3):
|
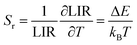 | (3) |
The dependence of relative thermal sensitivity Sr on temperature is shown in Fig. 4d, revealing that the maximum relative thermal sensitivity reaches an ultrahigh value of 2.20% K−1 at 200 K for CGF@CF NPs, which is higher than the value of 1.28% K−1 for CGF@CGF. Additionally, the relative thermal sensitivity of CGF@CF UCNPs (200 K) is more promising than that of similar reported UCNPs, as shown in Table S1.† Based on the results outlined, the excellent performance of the CGF@CF as a sensitive luminescence thermometer makes them promising candidates for applications where the temperature needs to be monitored with high precision and real-time. In Fig. S7,† we demonstrate the potential of this luminescent temperature probe for an in situ measurement technique in an extreme environment, such as a space satellite. Under the excitation of 980 nm diode lasers, a spectrometer collects the luminescence spectra, and the corresponding temperature changes can be obtained in real-time.
4 Conclusions
We have developed a coprecipitation synthesis method to synthesize ultra-small and efficient lanthanide-doped core@shell UCNPs. By optimizing of Ca2+/Gd3+ molar ratio, sub-5 nm alkaline-earth rare-earth fluoride cores were prepared. On the one hand, Gd3+ ions doping can reduce the CaF2 lattice symmetry, enhancing UCL. On the other hand, compared with homogeneous CaGdF shell of CGF@CGF NPs, heterogeneous CaF2 shell of CGF@CF NPs further increases the UCL intensity. Notably, the CGF@CF heterostructure is more sensitive to temperature changes due to lattice distortion in the interface, which is particularly interesting as multiphoton nanoprobes for applications in temperature sensing.
Author contributions
Y. C. and Q. L. conceived the experiments. X. X., H. C., W. W., and performed the experiments. X. X., Q. L., B. L., X. K., and Y. C. analyzed the data and prepared the manuscript. All authors contributed to manuscript writing and discussions.
Conflicts of interest
There are no conflicts to declare.
Acknowledgements
This work is financially supported by the National Key Research and Development Program of China (2021YFA0715603), National Natural Science Foundation of China (62075217, 11874354, 11874355, 61575194, 22172154). Project of Science and Technology Agency, Jilin Province (20210101148JC, 202512JC010475440, 20230508104RC) and the State Key Laboratory of Luminescence and Applications (SKLA-2019-02, SKLA-2020-09 and SKLA-Z-2022-08).
Notes and references
- D. Baziulyte-Paulaviciene, N. Traskina, R. Vargalis, A. Katelnikovas and S. Sakirzanovas, J. Lumin., 2019, 215, 116672–116679 CrossRef CAS.
- D. Li, Q. Shao, Y. Dong and J. Jiang, J. Alloys Compd., 2014, 617, 1–6 CrossRef CAS.
- C. Hu, L. Lei, E. Liu, Z. Lu and S. Xu, J. Lumin., 2022, 247, 118905–118910 CrossRef CAS.
- S. W. Hao, G. Y. Chen and C. H. Yang, Theranostics, 2013, 3, 331–345 CrossRef PubMed.
- X. F. Wang, Q. Liu, Y. Y. Bu, C. S. Liu, T. Liu and X. H. Yan, RSC Adv., 2015, 5, 86219–86236 RSC.
- G. G. Lin and D. Y. Jin, ACS Sens., 2021, 6, 4272–4282 CrossRef CAS PubMed.
- A. Nexha, J. J. Carvajal, M. C. Pujol, F. Diaz and M. Aguilo, Nanoscale, 2021, 13, 7913–7987 RSC.
- H. Liu, X. K. Jian, M. T. Liu, K. L. Wang, G. Y. Bai and Y. H. Zhang, RSC Adv., 2021, 11, 36689–36697 RSC.
- Y. F. Bai, Y. Yang, Y. W. Deng, S. H. Dang, Z. L. He, Z. W. Zhou and L. Liu, J. Phys. Chem. C, 2022, 126, 3830–3838 CrossRef CAS.
- W. Li, D. Wang, X. F. Li, P. Li, P. Fu, C. C. Hu, J. G. Hao, W. Li and Q. W. Zhang, J. Mater. Chem. C, 2022, 10, 11891–11902 RSC.
- J. S. Liao, M. H. Wang, F. L. Lin, Z. Han, B. A. Fu, D. T. Tu, X. Y. Chen, B. Qiu and H. R. Wen, Nat. Commun., 2022, 13, 2090–2100 CrossRef CAS PubMed.
- H. Liu, H. Y. Wang, X. K. Zheng, P. C. Wang and Y. H. Zhang, Dalton Trans., 2022, 51, 13106–13118 RSC.
- M. Y. Luo, X. Z. Sha, B. J. Chen, X. Z. Zhang, H. Q. Yu, X. P. Li, J. S. Zhang, S. Xu, Y. Z. Cao, Y. C. Wang, X. Wang, Y. H. Zhang, D. Gao and L. Wang, J. Am. Ceram. Soc., 2022, 105, 3353–3363 CrossRef CAS.
- S. Y. Han, R. R. Deng, X. J. Xie and X. G. Liu, Angew. Chem., Int. Ed., 2014, 53, 11702–11715 CrossRef CAS PubMed.
- B. Golesorkhi, H. Nozary, A. Furstenberg and C. Piguet, Mater. Horiz., 2020, 7, 1279–1296 RSC.
- B. Ren, B. Chen, J. Zhao, Y. Guo, X. Zhang, X. Chen, Y. Du, Z. Deng, G. Zhu and F. Wang, Chem. Mater., 2020, 33, 158–163 CrossRef.
- F. Wang and X. G. Liu, Chem. Soc. Rev., 2009, 38, 976–989 RSC.
- F. Wang, D. Banerjee, Y. S. Liu, X. Y. Chen and X. G. Liu, Analyst, 2010, 135, 1839–1854 RSC.
- S. Fischer, C. Siefe, D. F. Swearer, C. A. McLellan, A. P. Alivisatos and J. A. Dionne, Angew. Chem., Int. Ed. Engl., 2020, 59, 21603–21612 CrossRef CAS PubMed.
- F. Vetrone, V. Mahalingam and J. A. Capobianco, Chem. Mater., 2009, 21, 1847–1851 CrossRef CAS.
- M. Zeng, S. Singh, Z. Hens, J. Liu, F. Artizzu and R. Van Deun, J. Mater. Chem. C, 2019, 7, 2014–2021 RSC.
- Z. C. Li, D. C. Zhou, L. R. Jensen, J. B. Qiu, Y. F. Zhang and Y. Z. Yue, J. Am. Ceram. Soc., 2021, 104, 4471–4478 CrossRef CAS.
- F. Y. Ren, Y. Li, E. Y. B. Pun and H. Lin, J. Phys. Chem. C, 2021, 125, 12107–12117 CrossRef CAS.
- S. Chen, Z. Chu, L. Cao, L. Xu, Q. Jin, N. Liu, B. Chen, M. Fang, W. Wang, H. Qian and M. Shao, Nano Res., 2022, 15, 9298–9308 CrossRef CAS.
- Z. Chu, T. Tian, Z. Tao, J. Yang, B. Chen, H. Chen, W. Wang, P. Yin, X. Xia, H. Wang and H. Qian, Bioact. Mater., 2022, 17, 71–80 CrossRef CAS PubMed.
- K. Liu, X. Yan, Y.-J. Xu, L. Dong, L.-N. Hao, Y.-H. Song, F. Li, Y. Su, Y.-D. Wu, H.-S. Qian, W. Tao, X.-Z. Yang, W. Zhou and Y. Lu, Biomater. Sci., 2017, 5, 2403–2415 RSC.
- S. Fischer, R. D. Mehlenbacher, A. Lay, C. Siefe, A. P. Alivisatos and J. A. Dionne, Nano Lett., 2019, 19, 3878–3885 CrossRef CAS.
- X. Wu, S. Zhan, J. Han and Y. Liu, Nano Lett., 2021, 21, 272–278 CrossRef CAS PubMed.
- S. Fischer, R. D. Mehlenbacher, A. Lay, C. Siefe, A. P. Alivisatos and J. A. Dionne, Nano Lett., 2019, 19, 3878–3885 CrossRef CAS.
- X. Wu, S. Zhan, J. Han and Y. Liu, Nano Lett., 2021, 21, 272–278 CrossRef CAS.
|
This journal is © The Royal Society of Chemistry 2023 |
Click here to see how this site uses Cookies. View our privacy policy here.