DOI:
10.1039/D3RA00403A
(Paper)
RSC Adv., 2023,
13, 9231-9236
Solvent-controlled amidation of acid chlorides at room temperature: new route to access aromatic primary amides and imides amenable for late-stage functionalization†
Received
19th January 2023
, Accepted 6th March 2023
First published on 21st March 2023
Abstract
Herein, we report a solvent-controlled highly selective amidation and imidation of aroyl chlorides using an alkali-metal silyl-amide reagent (LiHMDS), which serves as a nitrogen source at room temperature. A unique feature of this method lies in the sequential silyl amidation of aryol chlorides and nitrogen–silicon bond cleavage of the corresponding N,N-bis(trimethylsilyl)benzamide in a one-pot method in a very short reaction time. This effective strategy was extended to late-stage functionalization.
Introduction
Amides and imides are vital and appealing functionalities that are ubiquitous in biology, pharmaceutical intermediates, natural products, and materials science.1 They are bestowed with remarkable properties which substantiate their existence in engineering plastics, lubricants, fertilizers, detergents, agrochemicals, proteins, etc.2,3 In particular, primary benzamides are found in various drugs, such as salicylamide, nicotinamide, labetalol, frovatriptan, niraparib, lenvatinib, ethosuximide and amonafide (Scheme 1a). Therefore, tremendous efforts have been made to develop synthetic methods for the preparation of primary amides. Classically, primary amides are accessed using carboxylic acid derivatives,4 aldehydes,5 alcohols,6,7 nitriles,8 and oximes.9–11 Later, various nonclassical strategies, such as oxidative-amidation,12 hydroamination13 and C–N coupling reactions,14 were developed. Very recently, new methodologies for the synthesis of primary amides were established. Mechanochemical, transamidation, ring-opening selective cleavage and direct amidation were developed by Menéndez,15 Lee,16 Lin17 and Chen,18 respectively.
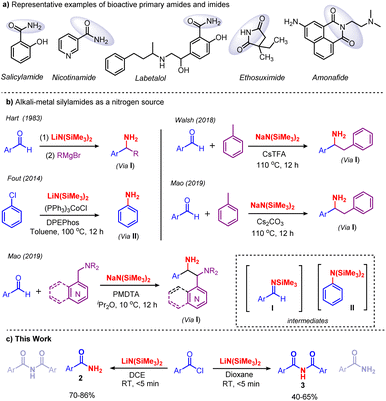 |
| Scheme 1 Metal-silylamides as a nitrogen source. | |
Traditionally, imides are prepared via two routes: (i) acylation of amides with acyl chlorides, carboxylic esters, and anhydrides,19 and (ii) a Mumm rearrangement of isoimides.20 However, both of these methods suffer from limited substrate scope; furthermore, Mumm rearrangement demands prefunctionalization and results in moderate yields.21 Recently, considerable effort has been devoted to the synthesis of imides, and in this regard several methods have been developed, such as the metal-catalyzed carbonylation of amides,22 the oxidation of amides,23 and the oxidative decarboxylation of amino acids,24 among others.25–33 Recently, the Liang group reported the synthesis of imides by the chemoselective acylation of N-acylglutarimides with N-acylpyrroles and aryl esters under transition-metal-free conditions.21 However, the reported imide synthesis displays certain drawbacks in terms of corrosive precursors, prefunctionalized substrates, specialized reagents, and excessive oxidants; besides, they are time-consuming with a limited substrate scope. The development of greener and more practical methods for the synthesis of primary amides and imides remains in demand. Our group has a long-standing interest in Brønsted base [MN(SiMe3)2, M = Li, Na and K] promoted organic transformations, especially the cleavage of C–C and C–O bonds in C-acyl imidazolium salts and esters, respectively.34 Recently, we have shown that KN(SiMe3)2 promotes the acylation of weakly acidic C–H bonds in toluene derivatives.35 Along these lines, in 1983 Hart and co-workers reported for the first time that [MN(SiMe3)2, M = Li, Na and K] acts as a base and nitrogen source in converting aryl aldehydes to aryl amines. Likewise, Fout, Walsh, and Mao used [MN(SiMe3)2, M = Li, Na and K] as a nitrogen source for amination reactions (Scheme 1b).36 To the best of our knowledge, the usage of [MN(SiMe3)2, M = Li, Na and K] as a nitrogen source for amination reactions at ambient temperature in a short reaction time is unknown. Herein, we report the solvent controlled metal-free, additive-free amidation and imidation of acid chlorides using LiN(SiMe3)2 as a nitrogen source in a very short reaction time at ambient temperature (Scheme 1c).
Results and discussion
Initially, the metal-free amidation of aroyl chlorides with LiN(SiMe3)2 (in THF) was investigated. The reaction of 1.0 equiv. benzoyl chloride 1 with 2.5 equiv. LiN(SiMe3)2 at room temperature in DCE for 5 min resulted in the formation of the benzamide 2a in 80% yield. An increment in LiN(SiMe3)2 (from 2.5 to 3.0 equiv.) reduced the yield of 2, while decreasing it (from 2.5 to 1.0 equiv.) resulted in the appearance of unidentifiable products (without hampering the reaction progression). In the absence of LiN(SiMe3)2 (in THF) no product was observed, suggesting that LiN(SiMe3)2 promotes the process. Other silyl amides like NaHMDS (in THF) and KHMDS (in Toluene) were tried. No reaction was observed in case of KHMDS in toluene (Table 1, entry 4), whereas reduction in the yield of 2 was observed when NaHMDS in THF was used (Table 1, entry 3). We next tested various nitrogen sources (ammonium salts, azide and urea). Among these, ammonium salts gave the desired primary amides, whereas LiHMDS were the best (Scheme 2). The relative importance of various solvents on the reaction, such as CHCl3, TFE, DCM, dioxane, DMF, THF, diethyl ether, acetonitrile, toluene, acetone, DMSO, ethyl acetate, ethanol and methanol, was examined (Table 1, entries 5–18). Among these, halogenated solvents showed a trace amount of 2 (Table 1, entries 5–7), and, surprisingly, non-halogenated solvents showed the formation of imide 3 devoid of 2 (Table 1, entries 9–18). Thus, dioxane was found to the best solvent to give 3 in a higher yield (63%) (Table 1, entry 8). This investigation reveals that the solvents play a crucial role in the formation of 2 and 3. Furthermore, various acyl sources, such as acids, esters, amides, C-acyl imidazolium salts, aliphatic acid chlorides, aromatic side chain carboxyl chlorides and α-substituted aromatic side chain carboxyl chlorides were examined (Scheme 3). Among these C-acyl imidazolium salts and α-substituted aromatic side chain carboxyl chlorides gave products 2 and 3, which reveals that this protocol is a substrate selective reaction.
Table 1 Optimization of the reaction conditions

|
Entry |
Nitrogen source |
Solvent |
Yield (%) |
2 |
3 |
1 |
LiHMDS in THF |
DCE |
80 |
10 |
2 |
LiHMDS in toluene |
DCE |
0 |
0 |
3 |
NaHMDS in THF |
DCE |
60 |
5 |
4 |
KHMDS in toluene |
DCE |
0 |
0 |
5 |
LiHMDS in THF |
CHCl3 |
10 |
0 |
6 |
LiHMDS in THF |
TFE |
5 |
0 |
7 |
LiHMDS in THF |
DCM |
4 |
45 |
8 |
LiHMDS in THF |
Dioxane |
10 |
63 |
9 |
LiHMDS in THF |
DMF |
0 |
38 |
10 |
LiHMDS in THF |
THF |
0 |
35 |
11 |
LiHMDS in THF |
Et2O |
0 |
30 |
12 |
LiHMDS in THF |
CH3CN |
0 |
25 |
13 |
LiHMDS in THF |
Toluene |
0 |
23 |
14 |
LiHMDS in THF |
Acetone |
0 |
10 |
15 |
LiHMDS in THF |
DMSO |
0 |
6 |
16 |
LiHMDS in THF |
EtOAc |
0 |
4 |
17 |
LiHMDS in THF |
EtOH |
0 |
0 |
18 |
LiHMDS in THF |
MeOH |
0 |
0 |
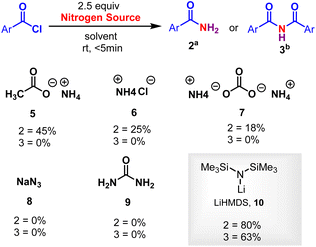 |
| Scheme 2 Other nitrogen sources. Reaction conditions were as follows: aroyl chloride 1 (0.2 mmol), LiHMDS (2.5 equiv.), aDCE (3 mL) and bdioxane (3 mL), RT, <5 min. | |
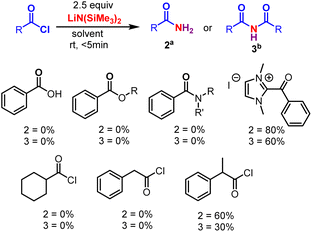 |
| Scheme 3 Other acyl sources. Reaction conditions were as follows: aroyl chloride 1 (0.2 mmol), LiHMDS (2.5 equiv.), aDCE (3 mL) and bdioxane (3 mL), RT, <5 min. | |
With the optimized conditions in hand, the substrate scope was first surveyed for the reaction of primary amides and symmetric imides (Scheme 4). Various examples of acid chlorides were subjected to the synthesis of corresponding products. The substrates with electron-withdrawing groups, 2d, 2e, 2g, 3d, 3e and 3g, were well tolerated compared with that to electron-releasing groups, 2b, 2c, 2f, 3b, 3c and 3f, in amidation and imidation reactions. Importantly, the amidation reaction does not progress with substrates possessing ortho substituents, 2m–2o. Successively, our intent was to explore unsymmetrical imides 4 (Scheme 4) by adopting this approach. The reaction of acid chloride 1 with primary amide 2a in the presence LiHMDS in dioxane at room temperature afforded unsymmetrical imides 4. There were no significant changes in yield with respect to electron-releasing or electron-withdrawing groups, 4a–4g. Additionally, poly- and heterocyclic substrates were compatible to give amides 2h–2k, symmetric imides 3h–3k, and unsymmetric imides 4h–4k in moderate to good yields. This protocol could be extended to the synthesis of drug nicotinamide 2l from its corresponding acid chloride. Scale-up experiments were performed in order to put forth the practicality of the solvent-controlled amidation and imidation methodology by using aroyl chloride (1 g, 7.14 mmol) as a test molecule under the optimized reaction conditions. As can be clearly seen from Scheme 4, the isolated yields of 2a (70% yield) and 3a (51%) are quite satisfactory. Late-stage functionalization strategies are currently receiving great interest in both the drug discovery and chemical biology, and in this regard, solvent controlled amidation and imidation studies were carried out on three different drug molecules, clofibric acids 2p and 3l, naproxens 2q and 3m, and ketoprofens 2r and 3n (Scheme 5).
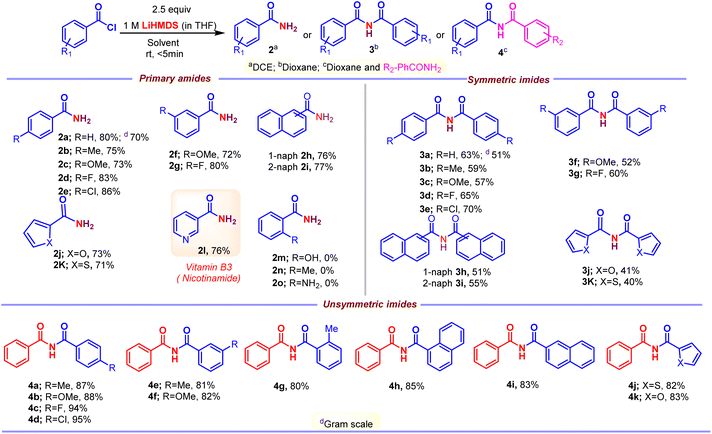 |
| Scheme 4 Synthesis of primary amides, symmetric and unsymmetric imides. Reaction conditions were as follows: aroyl chloride 1 (0.2 mmol), LiHMDS (2.5 equiv.), DCE (3 mL) and dioxane (3 mL), RT, <5 min. | |
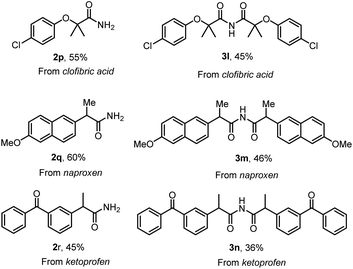 |
| Scheme 5 Late-stage functionalization of drugs. Reaction conditions were as follows: acid chloride 1 (0.2 mmol), LiHMDS (2.5 equiv.), DCE (3 mL) and dioxane (3 mL), RT, <5 min. | |
Further, to understand this methodology, a control experiment was carried out (Scheme 6). Precursor 1 reacted with other nitrogen sources (ammonium salts, azide and urea) under the optimal reaction conditions resulted in the formation of 2, and no 3 was formed. From this result, it is deciphered that non-silylamide nitrogen sources preferentially gave primary amides 2 whereas silylamide ones move forward to give 3 by reacting with aroyl chloride.
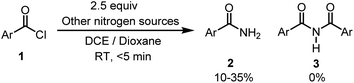 |
| Scheme 6 Control experiment. Reaction conditions were as follows: acid chloride 1 (0.2 mmol), LiHMDS (2.5 equiv.), DCE (3 mL) and dioxane (3 mL), RT, <5 min. | |
Based on the above studies and literature report, a plausible mechanism for the formation of primary amides and imides is depicted in Scheme 7. Initially, nucleophilic addition of the nitrogen in LiN(SiMe3)2 to the electrophilic carbonyl carbon of 1 forms a tetrahedral intermediate I. Then, I proceeds to form a key silylamide intermediate II 21 (ArC (
O)N(SiMe3)2) via LiCl elimination. Intermediate II can proceed through two different pathways based on the chosen solvent. In the presence of DCE (polar solvent), the chlorine atom in DCE coordinates with silicon in silyl amide intermediate II, which helps to cleave both nitrogen–silicon bonds simultaneously in silyl amide intermediate II instead of one more acid chloride to deliver amide 2 during the acidic work up. In the case of dioxane (non-polar solvents), the cleavage of the nitrogen–silicon bond in silyl amide intermediate II is difficult due to less solubility.37 Further, one more acid chloride is required to cleave the nitrogen–silicon bond in silyl amide intermediate II to form a new intermediate III, which leads to symmetric imides 3 during acidic work up.
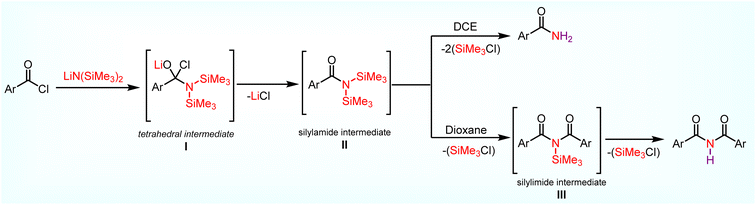 |
| Scheme 7 Plausible mechanistic pathways. | |
Conclusions
In summary, we developed a straightforward and efficient synthetic methodology for the synthesis of primary amides, symmetric and unsymmetric imides. Both the amidation (34 examples) and the late-stage functionalization of drugs (7 examples) worked smoothly with the yields ranging from 50 to 70%. The major advantages of the presented methodology over the existing ones are that (i) there is no need for the external amine source and oxidant, (ii) it does not require any metal catalyst, (iii) it enables the selective synthesis of primary amides and imides in high yields, (iv) it allows the derivatization of drug molecules, (v) it uses safe solvents at room temperature, and (vi) the reaction completes in a very short time, thus avoiding harsh conditions. Therefore, we think that this solvent-controlled amidation and imidation protocol will create a new perspective for the synthesis of primary amides/imides and related drug molecules.
Conflicts of interest
There are no conflicts to declare.
Acknowledgements
The Department of Science and Technology-SERB, India (CRG/2021/001143) and the Seed grant-SG20210167, Vellore Institute of Technology, Vellore, are gratefully acknowledged.
Notes and references
-
(a) A. Greenberg, C. M. Breneman and J. F. Liebman, The amide linkage: selected structural aspects in chemistry, bio-chemistry, and materials science, WileyInterscience, 2000 Search PubMed;
(b) G. Benz, Synthesis of amides and related compounds: comprehensive organic synthesis, Pergamon, 1991 Search PubMed.
-
(a) J. M. Humphrey and A. R. Chamberlin, Chemical synthesis of natural product peptides: coupling methods for the incorporation of noncoded amino acids into peptides, Chem. Rev., 1997, 97, 2243–2266 CrossRef CAS PubMed;
(b) M. K. Hargreaves, J. G. Pritchard and H. R. Dave, Cyclic carboxylic monoimides, Chem. Rev., 1970, 70, 439–469 CrossRef CAS.
-
(a) L. Mei and Y. Bing, A simple synthesis of salicylamide and 2,5-diphenylpyrazine, Res. Chem. Intermed., 2015, 41, 3491–3496 CrossRef CAS;
(b) A. Tahara, A. Matsuyama-Yokono and M. Shibasaki, Effects of antidiabetic drugs in high-fat diet and streptozotocin- nicotinamide-induced type 2 diabetic mice, Eur. J. Pharmacol., 2011, 655, 108–116 CrossRef CAS.
-
(a) Q. Song, Q. Feng and K. Yang, Synthesis of primary Amides via Copper-Catalyzed Aerobic Decarboxylative ammoxidation of Phenylacetic Acids and α-Hydroxyphenylacetic acids with Ammonia in water, Org. Lett., 2014, 16, 624–627 CrossRef CAS PubMed;
(b) A. R. Chhatwal, H. V. Lomax, A. J. Blacker, J. M. J. Williams and P. Marcé, Direct synthesis of amides from nonactivated carboxylic acids using urea as nitrogen source and Mg(NO3)2 or imidazole as catalysts, Chem. Sci., 2020, 11, 5808–5818 RSC.
-
(a) Y. Wang, W. Guo, A. L. Guan, S. Liu and Z. J. Yao, Half-Sandwich Iridium Complexes Based on β-Ketoamino Ligands: Preparation, Structure, and Catalytic Activity in Amide Synthesis, Inorg. Chem., 2021, 60, 11514–11520 CrossRef CAS PubMed;
(b) J. M. Concellón, H. Rodríguez-Solla, C. Concellón, C. Simal and N. Alvaredo, Sequential synthesis of (E)-α,β-unsaturated primary amides with complete stereoselectivity, J. Org. Chem., 2010, 75, 3451–3453 CrossRef PubMed;
(c) S. C. Ghosh, Copper-catalyzed oxidative amidation of aldehydes with amine salts: synthesis of primary, secondary, and tertiary amides, J. Org. Chem., 2012, 77, 8007–8015 CrossRef CAS PubMed.
- K. Yamaguchi, H. Kobayashi, T. Oishi and N. Mizuno, Heterogeneously Catalyzed Synthesis of Primary Amides Directly from Primary Alcohols and Aqueous Ammonia, Angew. Chem., Int. Ed., 2012, 51, 544–547 CrossRef CAS PubMed.
- K. Yamaguchi, H. Kobayashi, Y. Wang, T. Oishi, Y. Ogasawara and N. Mizuno, Green oxidative synthesis of primary amides from primary alco-hols or aldehydes catalyzed by a cryptomelane-type manganese oxide-based octahedral molecular sieve, OMS-2, Catal. Sci. Technol., 2013, 3, 318–327 RSC.
- X. Fan, W. Deng, Z. Liu and Z. Yao, Half-Sandwich Iridium Complexes for the One-Pot Synthesis of Amides: Preparation, Structure, and Diverse Catalytic Activity, Inorg. Chem., 2020, 59, 16582–16590 CrossRef CAS PubMed.
- R. García-Álvarez, A. E. Díaz-Álvarez, J. Borge, P. Crochet and V. Cadierno, Ruthenium-catalyzed rearrangement of aldoximes to primary amides in water, Organometallics, 2012, 31, 6482–6490 CrossRef.
- P. J. González-Liste, V. Cadierno and S. E. García-Garrido, Catalytic Rearrangement of Aldoximes to Primary Amides in Envi-ronmentally Friendly Media under Thermal and Microwave Heat-ing: Another Application of the Bis(allyl)-Ruthenium (IV) Dimer [{RuCl(μ-Cl) (η3:η3-C10H16)}2], ACS Sustain. Chem. Eng., 2015, 3, 3004–3011 CrossRef.
- J. Bosson, N. Marion and S. P. Nolan, Au/Ag-Cocatalyzed Aldoximes to Amides Rearrangement under Solvent- and Acid-Free Conditions, J. Org. Chem., 2010, 75, 1197–1202 CrossRef.
-
(a) J. Gao and G.-W. Wang, Direct oxidative amidation of aldehydes with anilines under mechanical milling conditions, J. Org. Chem., 2008, 73, 2955–2958 CrossRef CAS PubMed;
(b) S. C. Ghosh, J. S. Ngiam, A. M. Seayad, D. T. Tuan, C. L. Chai and A. Chen, Copper-catalyzed oxidative amidation of aldehydes with amine salts: synthesis of primary, secondary, and tertiary amides, J. Org. Chem., 2012, 77, 8007–8015 CrossRef CAS PubMed.
-
(a) Y. Uenoyama, T. Fukuyama, O. Nobuta, H. Matsubara and I. Ryu, Alkyne carbonylation by radicals: Tin-radical-catalyzed synthesis of alpha-methylene amides from 1-alkynes, carbon monoxide, and amines, Angew. Chem., Int. Ed., 2005, 44, 1075–1078 CrossRef CAS PubMed;
(b) W.-K. Chan, C.-M. Ho, M.-K. Wong and C.-M. Che, Oxidative amide synthesis and N-terminal α-amino group ligation of peptides in aqueous medium, J. Am. Chem. Soc., 2006, 128, 14796–14797 CrossRef CAS PubMed.
- G. Meng, S. Shi and M. Szostak, Cross-coupling of amides by N–C bond activation, Synlett, 2016, 27, 2530–2540 CrossRef CAS.
- J. Gómez-Carpintero, J. D. Sánchez, J. F. González and J. C. Menéndez, Mechanochemical Synthesis of Primary Amides, J. Org. Chem., 2021, 86, 14232–14237 CrossRef.
- J. Chen, Y. Xia and S. Lee, Transamidation for the Synthesis of Primary Amides at Room Temperature, Org. Lett., 2020, 22, 3504–3508 CrossRef CAS PubMed.
- K. Govindan and W. Y. Lin, Ring Opening/Site Selective Cleavage in N-Acyl Glutarimide to Synthesize Primary Amides, Org. Lett., 2021, 23, 1600–1605 CrossRef CAS PubMed.
- Y. Guo, R. Wang, J. Kang, Y. Ma, C. Xu, J. Li and X. Chen, Efficient synthesis of primary and secondary amides via reacting esters with alkali metal amidoboranes, Nat. Commun., 2021, 12, 1–9 CrossRef.
-
(a) A. Giovannini, D. Savoia and A. Umani-Ronchi, Organometallic ring-opening reactions of N-acyl and N-alkoxycarbonyl lactams. Synthesis of cyclic imines, J. Org. Chem., 1989, 54, 228–233 CrossRef CAS;
(b) M. B. Andrus and R. F. Keyes, Synthesis of mixed acyclic imides using pentafluorophenyl esters, Tetrahedron Lett., 1998, 39, 5465–5468 CrossRef CAS.
-
(a) J. P. Schwarz, Preparation of acyclic isoimides and their rearrangement rates to imides, J. Org. Chem., 1972, 37, 2906–2908 CrossRef CAS;
(b) K. Brady and A. F. Hegarty, The isoimide–imide rearrangement, J. Chem. Soc., Perkin Trans. 1, 1980, 2, 121–126 RSC.
- J. Li, J. Yao, L. Chen, D. Zou, P. J. Walsh and G. Liang, Chemoselective acylation of: N-acylglutarimides with N-acylpyrroles and aryl esters under transition-metal-free conditions, Org. Chem. Front., 2021, 8, 6344–6349 RSC.
-
(a) A. Schnyder and A. F. Indolese, Synthesis of unsymmetrical aroyl acyl imides by aminocarbonyla-tion of aryl bromides, J. Org. Chem., 2002, 67, 594–597 CrossRef CAS PubMed;
(b) L. Ran, Z. H. Ren, Y. Y. Wang and Z. H. Guan, Palladium-Catalyzed Aminocarbonylation of Aryl Iodides with Amides and N-alkyl Anilines, Chem.–Asian J., 2014, 9, 577–583 CrossRef CAS PubMed;
(c) H. Li, K. Dong, H. Neumann and M. Beller, Palladium-Catalyzed Hydroamidocarbonylation of Olefins to Imides, Angew. Chem., Int. Ed., 2015, 127, 10377–10381 CrossRef;
(d) Y. Li, K. Dong, F. Zhu, Z. Wang and X. F. Wu, Copper-Catalyzed Carbonylative Coupling of Cycloalkanes and Amides, Angew. Chem., Int. Ed., 2016, 55, 7227–7230 CrossRef CAS PubMed.
-
(a) L. Xu, S. Zhang and M. L. Trudell, Novel chromium (VI) catalyzed oxidation of N-alkylamides to imides with periodic acid, Chem. Commun., 2004, 14, 1668–1669 RSC;
(b) K. C. Nicolaou and C. J. Mathison, Synthesis of Imides, N-Acyl Vinylogous Carbamates and Ureas, and Nitriles by Oxidation of Amides and Amines with Dess–Martin Periodinane, Angew. Chem., Int. Ed., 2005, 117, 6146–6151 CrossRef;
(c) J. Sperry, The oxidation of amides to imides: a powerful synthetic trans-formation, Synthesis, 2011, 2011, 3569–3580 CrossRef;
(d) H. Yu, Y. Chen and Y. Zhang, TBHP/TEMPO-Mediated Oxidative Synthesis of Imides from Amides, Chin. J. Chem., 2015, 33, 531–534 CrossRef CAS.
-
(a) A. Itoh, T. Kodama, S. Inagaki and Y. Masaki, New synthetic method of imides through oxidative photodecar-boxylation reaction of N-protected α-amino acids with FSM-16, Chem. Lett., 2000, 29, 542–543 CrossRef;
(b) W. Huang, M. Wang and H. Yue, Conversion of N-acyl amino acids into imides via oxidative decarboxylation induced by Ag+/Cu2+/S2O82- in water, Synthesis, 2008, 1342–1344 CrossRef CAS.
- X. Ye, C. Xie, Y. Pan, L. Han and T. Xie, Copper-Catalyzed Synthesis of α-Amino Imides from Tertiary Amines: Ugi-Type Three-Component Assemblies Involving Direct Functionalization of sp3 C–Hs Adjacent to Nitrogen Atoms, Org. Lett., 2010, 12, 4240–4243 CrossRef CAS PubMed.
- T. Tomizawa, K. Orimoto, T. Niwa and M. Nakada, Preparation of imides via the palladium-catalyzed coupling reac-tion of organoborons with methyl N-[methoxy(methylthio)methylene]carbamate as a one-carbon elongation reaction, Org. Lett., 2012, 14, 6294–6297 CrossRef CAS PubMed.
- H. Aruri, U. Singh, S. Kumar, M. Kushwaha, A. P. Gupta, R. A. Vishwakarma and P. P. Singh, I2/aqueous TBHP-catalyzed coupling of amides with methylarenes/aldehydes/alcohols: metal-free synthesis of imides, Org. Lett., 2016, 18, 3638–3641 CrossRef CAS PubMed.
- C. S. Digwal, U. Yadav, P. V. S. Ramya, S. Sana, B. Swain and A. Kamal, Vanadium-Catalyzed Oxidative C(CO)-C(CO) Bond Cleavage for C-N Bond Formation: One-Pot Domino Transformation of 1,2-Diketones and Amidines into Imides and Amides, J. Org. Chem., 2017, 82, 7332–7345 CrossRef CAS PubMed.
- Y. Shen, Q. Li, G. Xu and S. Cui, Coupling of Carboxylic Acids with Ynamides and Subsequent Rear-rangement for the Synthesis of Imides/Amides, Org. Lett., 2018, 20, 5194–5197 CrossRef CAS PubMed.
- S. Ghorai and D. Lee, Synthesis of Imides, Imidates, Amidines, and Amides by Intercept-ing the Aryne-Isocyanide Adduct with Weak Nucleophiles, Org. Lett., 2019, 21, 7390–7393 CrossRef CAS PubMed.
- T. Brandhofer, A. Gini, S. Stockerl, D. G. Piekarski and O. García Mancheño, Direct C–H Bond Imidation with Benzoyl Peroxide as a Mild Oxi-dant and a Reagent, J. Org. Chem., 2019, 84, 12992–13002 CrossRef CAS PubMed.
- J. L. Xu, H. Tian, J. H. Kang, W. X. Kang, W. Sun, R. Sun, Y. M. Li and M. Sun, Ag(I)-catalyzed addition of cyclopropenones and nitrones to ac-cess imides, Org. Lett., 2020, 22, 6739–6743 CrossRef CAS PubMed.
- T. Yao, T. Yao, B. Wang, D. He, X. Zhang, X. Li and R. Fang, Ligand-controlled palladium-catalyzed chemoselective multicomponent reaction of olefin-tethered aryl halides, isocyanides, and carboxylic acids: Diversified synthesis of imides, Org. Lett., 2020, 22, 6784–6789 CrossRef CAS PubMed.
-
(a) S. Karthik, K. Muthuvel and T. Gandhi, Base-Promoted Amidation and Esterification of Imidazolium Salts via Acyl C–C bond Cleavage: Access to Aromatic Amides and Esters, J. Org. Chem., 2018, 84, 738–751 CrossRef PubMed;
(b) K. Muthuvel, S. Karthik and T. Gandhi, DBU-Promoted α-Acylation of 2-Oxindoles through Acyl C–C Bond Cleavage: Imidazolium Salts as an Emerging Acylating Agent, Asian J. Org. Chem., 2020, 9, 769–772 CrossRef CAS;
(c) P. K. R. Panyam, R. Sreedharan and T. Gandhi, Synthesis of topologically constrained naphthalimide appended palladium (II) – N-heterocyclic carbene complexes-insights into additive controlled product selectivity, Org. Biomol. Chem., 2020, 18, 3843–3847 RSC.
- R. Sreedharan, P. K. Pal, P. K. R. Panyam, U. D. Priyakumar and T. Gandhi, Synthesis of α-Aryl Ketones by Harnessing the Non-Innocence of Toluene and its Derivatives: Enhancing the Acidity of Methyl Arenes by a Brønsted Base and their Mechanistic Aspects, Asian J. Org. Chem., 2022, 11, 3–8 CrossRef.
-
(a) D. J. Hart, K. Kanai, D. G. Thomas and T. K. Yang, Preparation of primary amines and 2-azetidinones via N-Trimethylsilyl imines, J. Org. Chem., 1983, 48, 289–294 CrossRef CAS;
(b) Z. Wang, Z. Zheng, X. Xu, J. Mao and P. J. Walsh, One-pot aminobenzylation of aldehydes with toluenes, Nat. Commun., 2018, 9, 1–8 CrossRef PubMed;
(c) H. Guan, X. Cao, P. J. Walsh and J. Mao, One-Pot Aminoalkylation of Aldehydes: Diastereoselective Synthesis of Vicinal Diamines with Azaarylmethylamines, Org. Lett., 2019, 21, 8679–8683 CrossRef CAS PubMed;
(d) G. Liu, P. J. Walsh and J. Mao, Alkaline-Metal-Catalyzed One-Pot Aminobenzylation of Aldehydes with Toluenes, Org. Lett., 2019, 21, 8514–8518 CrossRef CAS PubMed.
- J. R. Bowser, P. J. Williams and K. Kurz, Cleavage of silicon-nitrogen bonds by acid chlorides: an unusual synthetic route to amides, J. Org. Chem., 1983, 48, 4111–4113 CrossRef CAS.
|
This journal is © The Royal Society of Chemistry 2023 |
Click here to see how this site uses Cookies. View our privacy policy here.