DOI:
10.1039/D3RA00135K
(Paper)
RSC Adv., 2023,
13, 4007-4010
Detection of alkali and alkaline earth metal ions using birefringence of hyperswollen lamellar phase†
Received
8th January 2023
, Accepted 20th January 2023
First published on 27th January 2023
Abstract
Chemical sensors have targeted various substances. Most sensors electrically amplify signals. Here, we propose a visual detection system that uses a hyperswollen lamellar phase and detects targets in a solution without electric amplification. Amphiphiles with an oligo(ethylene glycol) chain can catch alkali and alkaline earth metal ions and amplify to macroscopic birefringence.
Introduction
Chemical sensors have been applied to various substances, from simple ions to complicated bacteria. They have employed various detection methods: chromatography,1 flame reaction,2 fluorescent detection,3 precipitation reaction,4 conductivity detection,5 and discoloration.6 Fluorescent detection, the most widely used method for biology, diagnosis, and environmental chemistry benefits from the substrate-specific interactions between fluorescent molecules and the target substances: alkali metal ions,7 alkaline earth metal ions,8 protons,9 and nucleic acids.10 This method detects target substances by converting the interactions to fluorescent changes, which can be quantitatively detected as electric signals. Because each probe molecule reacts with its target substance at a molar ratio of one to one and diffuses three-dimensionally, we cannot detect the substance without any amplification processes that require electric power sources and devices: lasers, detectors, and fluorescence microscopes.11
As the detection methods without electric supplies, pH-test papers and gas detector tubes have been used.12–14 In these methods, we can visually observe the color change of the detector in response to the small number of targets because the probe molecules are immobilized to the substrates like filter papers and silica gels. The two-dimensional immobilization of the probe molecules can prevent signal attenuation caused by diffusion. However, it is not easy to prevent the diffusion of the probe molecules in the liquid phase. To visually detect the small number of targets in a solution without electric amplification, the self-assembly of the probe molecules should be effective in amplifying their small molecular deformation into large changes in the molecular assembled structures.
Liquid crystals can amplify signals through molecular cooperative behavior.15–19 They show texture changes in polarized optical microscopy when a certain chemical exists. To avoid using microscopies, we focused on the hyperswollen lamellar (HL) phase, a highly-diluted lyotropic liquid crystalline phase. The HL phase consists of the several-nanometers-thickness bilayers that keep several-hundred-nanometer intervals. The HL phase can visually display centimeter-sized typical birefringence textures without any microscopes due to their transparency and long correlation length.20–24 In vivo, chemicals are detected in cell membranes consisting of bilayers and proteins. The signals are transferred and amplified by a second messenger after the target substance binds with a receptor on a nerve cell. The bilayers in HL phases could also immobilize the probe molecules. The HL phase can probably amplify the change of the probe molecules induced by the interactions with the target. The added target substance binds with the probe molecules in the bilayers. The complexation breaks the bilayers, and the HL phase disappears. We can detect it through the disappearance of birefringence.
Fluorescent detection of alkali metals is possible by using a crown ether as a probe.25 The oligo(ethylene glycol) moiety of a crown ether attached to a chromophore strongly interacts with alkali metal ions, and the chromophore emits fluorescent light.26,27 Some organic solutions of a commercially available surfactant Brij L4 with an oligo(ethylene glycol) group show HL phases.23 Here we demonstrate a visual detection system using HL phases of amphiphilic compounds with an oligo(ethylene glycol) chain whose self-assembled structures sensitively respond to the addition of alkali metal ions, as shown in Fig. 1.28,29 We confirm the texture change of the HL phase with the addition of alkali and alkaline earth metal ions.
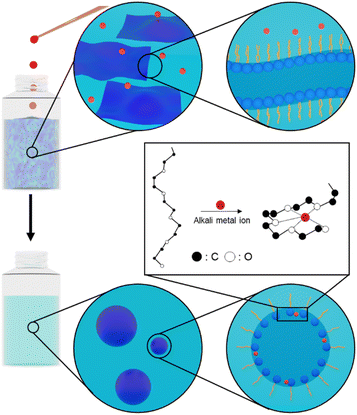 |
| Fig. 1 Schematic illustration of the present detection method with the deformation of the HL phase. The metal ions (red dots) bind with the oligo ethylene glycol moieties (blue parts) of the amphiphiles. The complexation breaks the bilayers, and the birefringence disappears. | |
Experimental
Chemicals
Heptane, methanol, lithium chloride, sodium chloride, potassium chloride, calcium chloride, barium chloride, lithium hydroxide, sodium hydroxide, potassium hydroxide, sodium carbonate, sodium hydrogen carbonate, and sodium nitrate were purchased from Wako Pure Chemical Industries Co. Brij L4 was purchased from Merck KGaA. The water with a resistivity of 18.2 MΩ cm was obtained from a water purifier (Direct-Q UV, Millipore Co).
Stability test
The HL phase solution consists of decane (4.8 × 10−3 M), Brij L4 (1.5 × 10−4 M) and deionized water (5.6 × 10−4 M). The target salts were added to the decane solution (40 mL). After sonication, we confirmed the birefringence of the solution at 25 °C. As long as the birefringence was maintained, we added target compounds. We defined the concentration at which birefringence disappears as a critical concentration (Cc).
Results and discussion
The organic solutions of Brij L4, a mixture containing tetraethyleneglycol monododecyl ether (C12E4) as the main component, exhibit HL phases when a small amount of water is added, as shown in Fig. S1.†23 We can check if the solutions show the birefringence using polarizing films, as shown in Fig. 2a.22 The polarized photograph of the decane solution of Brij L4 (1.5 × 10−4 M) and water (5.6 × 10−4 M) shows a texture typical of HL phases originating from their birefringence, as shown in Fig. 2b. When we added sodium chloride (NaCl, >1.9 × 10−5 M) to the decane solution showing an HL phase (40 mL), the HL phase disappeared, as shown in Fig. 2c. Even when we added hydrogen chloride (HCl, 6.1 × 10−5 M) to the decane solution, the solution kept showing the HL phase maintained, as shown in Fig. S2.† It indicates that the main factor is Na+ ions. Adding Na+ ions should destabilize the HL phases due to the interactions between oligo(ethylene glycol) chains of Brij L4 and Na+ ions, as shown in Fig. 1. The interactions probably affect the single-molecular shape of Brij L4 and the intermolecular interactions between Brij L4 molecules.30,31 They could be important for the stability of the HL phase.32
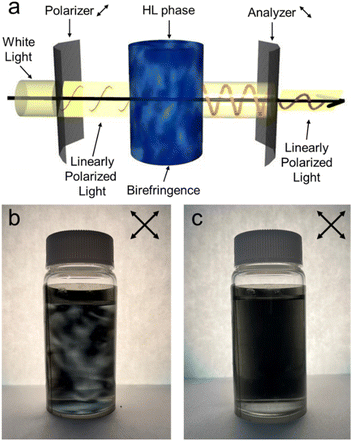 |
| Fig. 2 Observation of birefringence. (a) The optical system to observe the birefringence using a polarizing film wrapped around a vessel while tilted at 45°. Polarized photographs of decane solution of water (5.6 × 10−4 M) and Brij L4 (1.5 × 10−4 M) (b) without additives and (c) with NaCl (1.9 × 10−5 M). | |
We should examine if the destabilization should occur by adding different cations. When we added some other chloride salts to the decane solution of Brij L4 that shows an HL phase (40 mL), as shown in Table 1, lithium chloride (LiCl, >3.0 × 10−5 M), sodium chloride (NaCl, >1.9 × 10−5 M), potassium chloride (KCl, >3.2 × 10−5 M), calcium chloride (CaCl2, >9.0 × 10−6 M) and barium chloride (BaCl2, >5.9 × 10−6 M) destabilized the HL phase, as shown in Fig. S3.† The molar ratio required for destabilizing the HL phase (critical concentration, Cc) depends on the type of cations. It seems to come from the association constant of the oligo(ethylene glycol) chain and cations. It depends on the combination of the length of the oligo(ethylene glycol) chain and the ionic diameter of the cations.26 It also depends on the charge density of cations.33 Although Na+ and Ca2+ ions are almost the same sizes, Ca2+ ions interact more strongly with the oligo(ethylene glycol) chains than Na+ ions.
Table 1 Cc of alkali and alkaline earth metal chloride
|
LiCl |
NaCl |
KCl |
CaCl2 |
BaCl2 |
Cc [M] |
3.0 × 10−5 |
1.9 × 10−5 |
3.2 × 10−5 |
9.0 × 10−6 |
5.9 × 10−6 |
Cation radius [pm] |
60 |
95 |
133 |
99 |
135 |
We added alkali metal hydroxides to the decane solution (40 mL), showing an HL phase, as shown in Table 2. The addition of lithium hydroxide (LiOH, >1.1 × 10−5 M), sodium hydroxide (NaOH, >4.9 × 10−6 M), and potassium hydroxide (KOH, >9.2 × 10−6 M) also destabilized the HL phase, as shown in Fig. S4.† The solubilities of calcium hydroxide (Ca(OH)2) and barium hydroxide (Ba(OH)2) are too low to be applied to the same tests. Among the three alkali metal ions, Na+ ion is the most effective. The critical concentrations of alkali hydroxide salts to Brij L4 are about one-third that of alkali chloride salts. These results imply that the anions also play important roles in the disturbance of HL phases.
Table 2 Cc of alkali metal hydroxide and chlorides
|
LiOH |
NaOH |
KOH |
LiCl |
NaCl |
KCl |
Cc [M] |
1.1 × 10−5 |
4.9 × 10−6 |
9.2 × 10−6 |
3.0 × 10−5 |
1.9 × 10−5 |
3.2 × 10−5 |
We measured the effect of the anion by adding various sodium compounds to the decane solution (40 mL), as shown in Table 3. The data shows that the effect of the sodium compounds on the stability of the HL phase depends on the type of anions. As mentioned above, the main factor of the additives is cations. Cations make complexes with the oligo(ethylene glycol) chain, and the bilayers in the HL phases change their stabilities. The effect of the anions would be secondary. We came up with several scenarios. Acidity constant, size, pH, charge density and salting out could be effective. We found that the order of the critical concentrations is consistent with that of the salting-out effects of the anions, i.e., the Hofmeister series: OH− ≈ CO32− > HCO3− > Cl− > NO3−.34–37 These results indicate that the bilayers destabilized by the association of oligo(ethylene glycol) chains and metal ions are salted-out in the presence of anions.
Table 3 Cc of sodium compounds
|
NaOH |
Na2CO3 |
NaHCO3 |
NaCl |
NaNO3 |
Cc [M] |
4.9 × 10−6 |
2.2 × 10−6 |
1.3 × 10−5 |
1.9 × 10−5 |
3.8 × 10−5 |
[Na+] [M] |
4.9 × 10−6 |
4.4 × 10−6 |
1.3 × 10−5 |
1.9 × 10−5 |
3.8 × 10−5 |
Conclusions
We have confirmed that the present system can detect the existence of alkali and alkaline earth metal ions by observing the birefringence of the HL phase. The addition of alkali and alkaline earth metal compounds destabilized the HL phase. The association of oligo(ethylene glycol) chains and cations and the salting-out-like effect of anions probably originate the destabilization. The critical concentration seems to depend on the diameter and charge density of cations. Anions should be the second factor of destabilization; anions might induce undesired interactions between the complexes of oligo(ethylene glycol) chains and cations like salting-out. We have successfully detected the presence of the alkali and alkaline earth metal ions; however, it is still difficult to identify which ions exist. This method could apply to detecting various materials by choosing the appropriate probes, though it cannot apply to no-water and extreme pH conditions. In principle, the detection limit can be arbitrarily controlled by appropriately setting the initial HL phase stability.
Conflicts of interest
There are no conflicts to declare.
Acknowledgements
We thank Prof. H. Kamada and Prof. F. Sakurai for helpful advice. This work was supported in part by JSPS KAKENHI Grant Number JP22H04477 and JP22J10688.
Notes and references
- C. E. Dent, Biochem. J., 1947, 41, 240–253 CrossRef CAS PubMed.
- A. R. Clark, J. Chem. Educ., 1935, 12, 242–243 CrossRef CAS.
- A. H. Coons and K. H. Melvin, J. Exp. Med., 1950, 91, 1–13 CrossRef CAS PubMed.
- M. Arnac and G. Verboom, Anal. Chem., 1974, 46, 2059–2061 CrossRef CAS.
- J. S. Fritz, D. T. Gjerde and R. M. Becker, Anal. Chem., 1980, 52, 1519–1522 CrossRef CAS.
- R. Ishida, H. Narita and K. Tonosaki, Bull. Chem. Soc. Jpn., 1978, 51, 1751–1754 CrossRef CAS.
- A. Minta and R. Y. Tsien, J. Biol. Chem., 1989, 264, 19449–19457 CrossRef CAS PubMed.
- H. Komatsu, N. Iwasawa, D. Citterio, Y. Suzuki, T. Kubota, K. Tokuno, Y. Kitamura, K. Oka and K. Suzuki, J. Am. Chem. Soc., 2004, 126, 16353–16360 CrossRef CAS PubMed.
- J. Han and K. Burgess, Chem. Rev., 2010, 110, 2709–2728 CrossRef CAS PubMed.
- G. Patonay, J. Salon, J. Sowell and L. Strekowski, Molecules, 2004, 9, 40–49 CrossRef CAS PubMed.
- Y.-H. Shin, M. T. Gutierrez-Wing and J.-W. Choi, J. Electrochem. Soc., 2021, 168, 017502 CrossRef CAS.
- E. W. Rice, Ind. Eng. Chem., 1912, 4, 229 CrossRef CAS.
- A. B. Lamb and C. R. Hoover, US Pat., US1321062A, 1918 Search PubMed.
- K. Kawamura, K. Miyazawa and L. Kent, Appl. Chem., 2021, 1, 14–41 Search PubMed.
- H. Eimura, D. S. Miller, X. Wang, N. L. Abbott and T. Kato, Chem. Mater., 2016, 28, 1170–1178 CrossRef CAS.
- Y. Wang, Q. Hu, T. Tian, Y. Gao and L. Yu, Colloids Surf., B, 2016, 147, 100–105 CrossRef CAS PubMed.
- P. Popov, L. W. Honaker, E. E. Kooijman, E. K. Mann and A. I. Jákli, Sens. Bio-Sens. Res., 2016, 8, 31–35 CrossRef.
- J. Shen, F. J. He, L. C. Chen, L. Ding, H. L. Liu, Y. Wang and X. L. Xiong, Microchim. Acta, 2017, 184, 3137–3144 CrossRef CAS.
- Y. Han, K. Pacheco, C. W. M. Bastiaansen, D. J. Broer and R. P. Sijbesma, J. Am. Chem. Soc., 2010, 132, 2961–2967 CrossRef CAS PubMed.
- F. C. Larche, J. Appell, G. Porte, P. Bassereau and J. Marignan, Phys. Rev. Lett., 1986, 56, 1700–1703 CrossRef CAS PubMed.
- R. Strey, R. Schomäcker, D. Roux, F. Nallet and U. Olsson, J. Chem. Soc. Faraday. Trans., 1990, 86, 2253–2261 RSC.
- K. Sasaki, T. Okue, T. Nakai, Y. Uchida and N. Nishiyama, Langmuir, 2021, 37, 5872–5877 CrossRef CAS PubMed.
- K. Sasaki, T. Okue, Y. Shu, K. Miyake, Y. Uchida and N. Nishiyama, Dalton Trans., 2021, 50, 10394–10399 RSC.
- K. Sasaki, K. Miyake, Y. Uchida and N. Nishiyama, ACS Appl. Nano Mater., 2022, 5, 4998–5005 CrossRef CAS.
- C. J. Pedersen, J. Am. Chem. Soc., 1967, 89, 7017–7036 CrossRef CAS.
- J. Yin, Y. Hu and J. Yoon, Chem. Soc. Rev., 2015, 44, 4619–4644 RSC.
- D. E. Fenton, J. M. Parker and P. V. Wright, Polymer, 1973, 14, 589 CrossRef CAS.
- T. Kato, Science, 2002, 295, 2414–2418 CrossRef CAS PubMed.
- M. Yoshio, T. Mukai, K. Kanie, M. Yoshizawa, H. Ohno and T. Kato, Adv. Mater., 2002, 14, 351–354 CrossRef CAS.
- S. Yanagida, K. Takahashi and M. Okahara, Bull. Chem. Soc. Jpn., 1978, 51, 3111–3120 CrossRef CAS.
- S. Yanagida, K. Takahashi and M. Okahara, Bull. Chem. Soc. Jpn., 1978, 51, 1294–1299 CrossRef CAS.
- Y. Uchida, T. Nishizawa, T. Omiya, Y. Hirota and N. Nishiyama, J. Am. Chem. Soc., 2016, 138, 1103–1105 CrossRef CAS PubMed.
- Y. Tian, W. Chen, Z. Zhao, L. Xu and B. Tong, J. Mol. Model., 2020, 26, 67 CrossRef CAS PubMed.
- M. Randall and C. F. Failey, Chem. Rev., 1927, 4, 285–290 CrossRef CAS.
- P. Lo Nostro and B. W. Ninham, Chem. Rev., 2012, 112, 2286–2322 CrossRef CAS PubMed.
- A. M. Hyde, S. L. Zultanski, J. H. Waldman, Y.-L. Zhong, M. Shevlin and F. Peng, Org. Process Res. Dev., 2017, 21, 1355–1370 CrossRef CAS.
- K. P. Gregory, G. R. Elliott, H. Robertson, A. Kumar, E. J. Wanless, G. B. Webber, V. S. J. Craig, G. G. Andersson and A. J. Page, Phys. Chem. Chem. Phys., 2022, 24, 12682–12718 RSC.
|
This journal is © The Royal Society of Chemistry 2023 |
Click here to see how this site uses Cookies. View our privacy policy here.