DOI:
10.1039/D2RA08315A
(Review Article)
RSC Adv., 2023,
13, 11652-11684
Retracted Article: Aryl glyoxal: a prime synthetic equivalent for multicomponent reactions in the designing of oxygen heterocycles
Received
30th December 2022
, Accepted 6th March 2023
First published on 14th April 2023
Abstract
The category of bifunctional building blocks overrides many others because of their fascinating wide applicability in synthetic chemistry. Aryl glyoxal is one of the key molecules that has been extensively used in heterocyclic chemistry to afford nearly all types of five- and six-membered heterocycles, which are the structural constituents of many natural products. The multicomponent reaction is a practical strategy to utilize this wonderful moiety with different types of starting materials to obtain numerous diverse oxygen heterocycles. This review covers the advancement of aryl glyoxal as a prime synthetic equivalent in recent years for the synthesis of oxygen heterocycles.
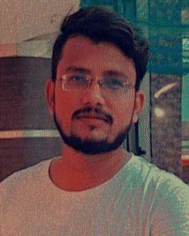 Abdur Rehman Sheikh | Abdur Rehman Sheikh was born and grew up in the district of Jaunpur, Uttar Pradesh, India. He obtained his BSc (Hons.) in chemistry and then MSc degree in organic chemistry in the year 2020 and 2022, respectively, from Aligarh Muslim University, (AMU), India. He has completed his Master's dissertation, titled “Synthesis of β-enamino imides”, under the supervision of Dr Md. Musawwer Khan. He is currently pursuing an Advance PG diploma (Nanotechnology) at AMU, Aligarh. He is an active member of the American Chemical Society (ACS) International Student Chapter AMU Aligarh. He has been working at Chegg Incorporated Limited as a subject matter expert since 2018. He has also attended various workshops, webinars, and conferences to explore this field of research. |
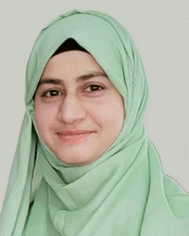 Anam Arif | Anam Arif was born in 1998 in Sambhal district, Uttar Pradesh, India. She obtained her bachelor's degree from Mahatma Jyotiba Phule Rohilkhand University, Bareilly in 2017 and master's degree in chemistry in 2019 from Aligarh Muslim University, Aligarh, India. She has completed her master's thesis, titled “DBU catalyzed one-pot synthesis of fused-1,4-dihydropyridines”. She is currently working as a research scholar under the supervision of Dr Md. Musawwer Khan, Department of Chemistry, Aligarh Muslim University, Aligarh, India. Her research interest is the development of green methodologies for the synthesis of novel heterocycles. |
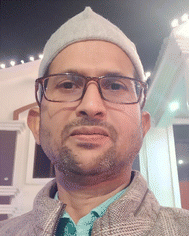 Md. Musawwer Khan | Dr Md. Musawwer Khan is presently working as an associate professor in the Department of Chemistry, Aligarh Muslim University. He has been awarded his PhD degree from IIT, Guwahati under the supervision of Prof. A. T. Khan. He completed his BSc, MSc, and B.Ed. degrees from AMU, Aligarh in 2001, 2003, and 2004, respectively. He has received several professional recognition fellowships/awards, including the Bentham Sciences Brand Ambassador of India (2020); RSC Best Cited Author Certificate (2018); Start-up Research grant (UGC) (2012); Indian Academy of Sciences-Summer Research Fellowship 2012; Senior Research Fellowship (2009); Junior Research Fellowship (2007); DST-Pre-Doctoral Fellowship (2006); and Post Graduate Merit Scholarship (2002 and 2003). Dr Khan has published more than 35 research papers in various international refereed journals of repute, including J. Org. Chem. (ACS), ACS Omega (ACS), Tetrahedron (Elsevier), Tetrahedron Letters (Elsevier), Carbohydrate Res. (Elsevier), RSC Advanced (RSC), New J. Chem. (RSC), ChemistrySelect (Wiley), Synthetic Communication (Taylor & Francis), Curr. Org. Chem. (Bentham Sciences), and J. Heterocyl. Chem. (Wiley). His current research interests include diversity oriented MCRs, the development of novel synthetic methodologies, heterocyclic chemistry, carbohydrate chemistry, and target-oriented synthesis for biologically significant molecules. |
1. Introduction
Organic synthesis has served as an all-time interesting topic for researchers from the very beginning. There have been many changes in synthesis paradigms since the first synthesis of an organic compound by Wöhler.1 The quest for easy and efficient methodologies brought about the concept of the one-pot multicomponent reaction approach. Multicomponent reactions (MCRs) are a type of reaction where at least three different starting materials are made to react in a single reaction vessel.2–5 The availability of various reacting sites in the reaction mixture increases the chances of probable novelty in the desired product. Therefore, MCRs have expedited organic synthesis by a significant level in novel compound formation. The pronounced benefits of multicomponent reactions, like efficient atom economy, minimization of extra time consumption, and reduced environmental waste, over traditional methods have revolutionized the work of synthetic chemists to produce a chemical library of novel moieties.6 MCRs are the only way to meet the intermittent demands of advanced technology-driven research, such as medicines, drugs, agrochemicals, polymers, and cosmetic industries. Many important building blocks and manifolds are the results of rationally designed multicomponent named reactions. Owing to their significance, recently reagent- and substrate-based multicomponent reactions are being reported in increasingly high numbers.7,8
Heterocycles occupy a key functional position in many living processes, and consequently provide an in-line sustained research area to be worked upon. Among the heterocycles, hydrocarbons with oxygen as their constituent element in the ring exhibit a special place in the category owing to their several benefits to the life. In addition to their occurrence in natural products and living systems, including the human body, scaffolds belonging to each class are known to show distinctive biological and pharmacological properties. Pyran derivatives are often screened for antianaphylatic, diuretic, spasmolytic, anticoagulant, and anticancer properties, etc.9–11 Alkaloids possessing pyranoquinoline as the structural unit feature anti-microbial characteristics.12 Also, a number of them act as photoactive enhancers in the treatment of neurodegenerative diseases.13,14 Another oxygen heterocycle, namely the furan skeleton, is available in combranolides,15 kailolides,16 in fragrances, and in dye-like commercial products. The furan derivative furoquinolinone blocks the potassium channel Kv1.3, which is the target for immunosuppressive therapy in the treatment of auto-immune diseases and transplantations.17 Likewise, the pyrazoline derivative containing a furan moiety shows anti-malarial activity against Plasmodium falciparum.18 Isoxazoles, as oxygen-nitrogen heterocycles, add to the synthetic utility of oxygen heterocycles as they offer anti-tubercular,19 anti-inflammatory,20 and COX-2 inhibitor properties,21 and are thus a constituent of many therapeutic drugs. The newly synthesized 2H-chromene-2-one derivative was tested and found to show the potential anti-convulsant22 activity. One of the benzopyranone derivatives, Enasculin, is a pharmacologically tested neuronal activator KA 672-HCl, which works as an antagonist by simultaneously activating several neurotransmitters that are deactivated in dementia. Further, the structural presence of benzo[g]chromene in many natural products with reported anticancer activity and the synergistic effect of β-lapachone with Taxol against tumour growth23–25 are driving the search for newer strategies to synthesize oxygen heterocyclic moieties in economical and environmentally friendly conditions. The therapeutic functions of some O-heterocycles are illustrated in Fig. 1.
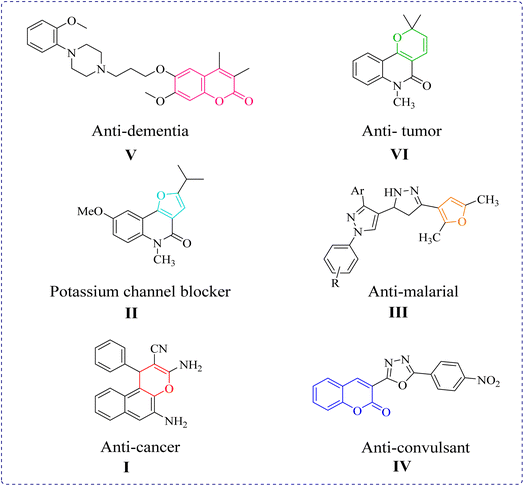 |
| Fig. 1 Some biologically active O-heterocycles. | |
The revolutionary advancements in the physiological studies of living beings for the sake of mankind and the environment seek acknowledgment from organic biomimetic pathways adopted by synthetic chemists to produce optimum results. So, the challenging process of synthesizing heterocyclic moieties can be facilitated by employing bifunctional building blocks in the reaction design, in which the multiple reactive centres lead to product diversity. Aryl glyoxal is one of the Aldo-ketone bifunctional building block molecule used by synthetic chemists to produce a diverse library of molecules.26 The presence of a reactive aldehyde group adjacent to the carbonyl group is the peculiar structural feature that makes aryl glyoxal distinctive for the synthesis of heterocyclic as well as carbocyclic compounds. The electron-withdrawing ketone group makes aryl glyoxal more reactive than benzaldehyde and allows the site to be open to nucleophilic attack followed by cyclization in various ways. Further, the non-enolizability of the ketone group under acidic or basic conditions is responsible for its sufficient stability and for making aryl glyoxal monohydrate commercially available. Interestingly, besides the numerous applications of all types of oxygen heterocycles as drugs and therapeutic agents, there are some biologically potent molecules with a phenyl glyoxal unit embedded in their structure (as shown in Fig. 2), such as 4-aroyl chromene derived from phenyl glyoxal hydrate and naphthyl glyoxal, which have shown antibacterial properties by inhibiting Escherichia coli growth with a minimum inhibitory concentration (MIC) of 32 μg cm−3.27 Also, a furan-substituted guaiazulene moiety synthesized through a simple route by utilizing phenyl glyoxal exhibited significant in vitro anti-oxidant activity against lipid peroxidation with a minimum IC50 value of 3.9 μg mL−1.28 Guaiazulene, a derivative of azulene, acts therapeutically against skin and asthma-like diseases due to allergy or inflammation reactions.29 Coumarin targets the α-glucosidase enzyme, which is responsible for the hydrolysis of starch and higher carbohydrates into simple sugars.30 Dihydrochromeno[4,3-b]pyrrol-3-yl obtained by using phenyl glyoxal as one of the starting materials exhibits α-glucosidase inhibitory activity, thus aiding the drug-development process for hyperglycaemia and Type 2 diabetes mellitus (T2DM).31 This in vitro evaluation was further extended by in silico docking studies with reference to the standard drug acarbose.32 Also, attempts are being made to synthesize the C-4 aroyl group-substituted pyrano[3,2-c]chromene and benzo[g]chromene by employing phenyl glyoxal due to their divergent properties.33,34 A new HIV integrase inhibitor, namely a pyrano[2,3-d:6,5-d′]dipyrimidine (V-165)-like framework of pyrano[2,3-d:6,5-d′]dipyrimidines, constructed through phenyl glyoxal showed increased chances for extensive use in drug precursors development.35 Phenyl glyoxal was further used in the synthesis of a furo(2,3-b)furan moiety36 mimicking drug candidates like Brecanavir (GW640385), an HIV inhibitor37 and Darunavir (TMC-114),38,39, another HIV-1 protease inhibitor. A coumarin-glyoxal hybrid, namely the tartrate salt of a Mannich base bearing coumarin derivatives,40 was found to be an efficient contraceptive as it shows activity as both a spermicide and microbicide. The compound was tested for spermicidal activity against nonoxynol (N-9),41 a contraceptive. The two compounds of the series showed activity better than N-9. For anti-microbial activity, again the two compounds showed activity better than the metronidazole42 chosen as the standard for the study. For this reason, from many years in the past up to recent years, aryl glyoxal has been extensively used and studied as a key building block in multicomponent single-pot reactions.43
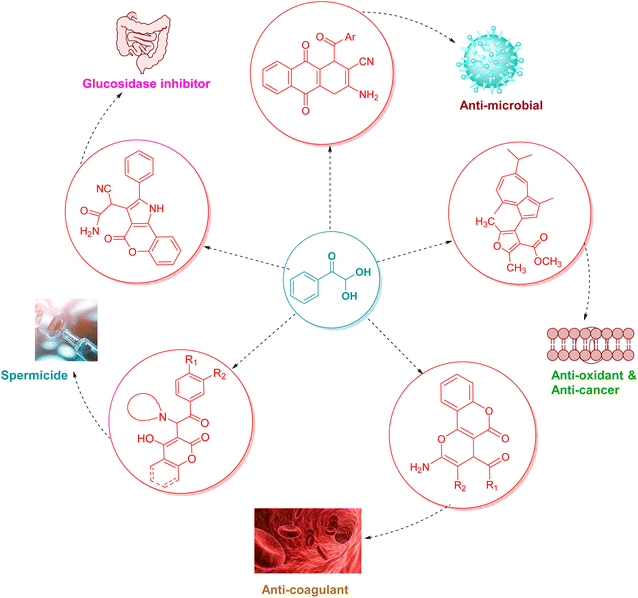 |
| Fig. 2 Biologically active O-heterocycles obtained from phenyl glyoxal. | |
In this review article, we intend to highlight the extensive use of the bifunctional building block aryl glyoxal monohydrate as a key starting material in the construction of many novel and mimics of naturally found heterocyclic moieties. Here, is a brief account of the reactivity pattern of aryl glyoxal with different substrates leading to the synthesis of various derivatives and fused oxygen heterocycles.
2. Use of aryl glyoxal in designing O-heterocycles using multicomponent reactions
Aryl glyoxal is unique in having two adjacent carbonyl groups, but it mostly exists in the hydrated form. It forms different types of five-membered and six-membered heterocyclic rings depending on the type of other substrates, attacking nucleophiles, and cyclization pattern in the reaction. The application of aryl glyoxal in the synthesis of five- and six-membered O-heterocycles is discussed in the following sections.
2.1. Synthesis of five-membered furan derivatives
Jian's group efficiently utilized aryl glyoxal 1 in the gold-catalyzed three-component reaction with amine 2 and a terminal alkyne 3 to obtain substituted furans 4 through cyclization in a nitrogen atmosphere with methanol as a solvent. This reaction provides an effective protocol for the preparation of synthetic and pharmacological derivatives of furan (Scheme 1).44 The plausible mechanism of the protocol started from the coupling of aryl glyoxal 1, amine 2, and alkyne 3 in a Mannich–Grignard pattern to give a propargyl intermediate aa, which was followed by the attack of the oxygen lone pair to the electrophilic triple bond, forming a cation ab and then leading to the final product indolizines 4 through deprotonation and demetallation, respectively (Scheme 2).
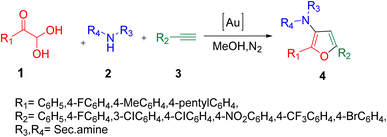 |
| Scheme 1 Gold-catalyzed three-component synthesis of furan derivatives 4.44 | |
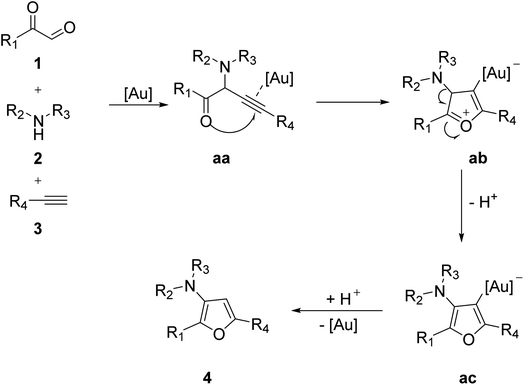 |
| Scheme 2 Mechanism proposed to explain gold-catalyzed furan synthesis. | |
For the synthesis of isoxazolyl amino furo[3,2-c]quinolinone scaffolds 7, Nagi and co-workers developed an efficient one-pot, three-component reaction of aryl glyoxal monohydrate, 4-hydroxy-1-methyl-2-quinilinone 6, and 4-amino-3-methyl-5-styrylisoxazoles 5 under reflux conditions in aqueous medium with a 5 mol% of p-TSA. The scheme was tested with different Lewis acids and organic acids, but p-TSA in water was found to give the best results in terms of the time, yield, and environmentally friendly protocol (Scheme 3).45 The reaction mechanism (as shown in Scheme 4) starts from the p-TSA-catalyzed condensation of compound 5 and 1 yielding the iminium ion intermediate ba, which serves as an electrophilic site for the nucleophile 6. The nucleophilic addition reaction between ba and 6 produces the bb intermediate, which is in tautomeric isomerization with bc. The intermediate bd undergoes intramolecular cyclization initiated by the acid to give the final product 7 through the dehydration of be (Scheme 4).
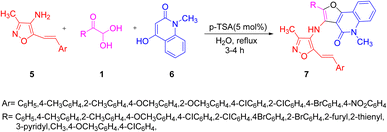 |
| Scheme 3 Synthesis of isoxazolyl amino furo[3,2-c]quinolinone scaffolds 7 using P-TSA.45 | |
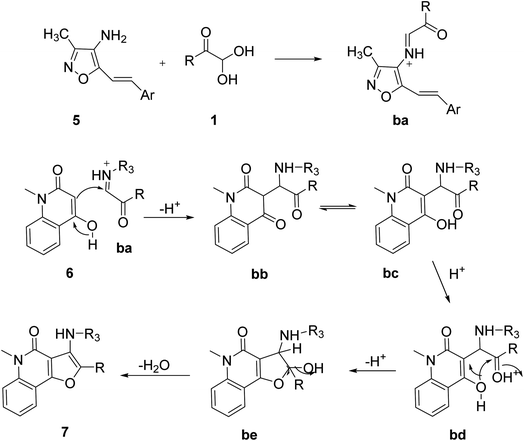 |
| Scheme 4 Mechanistic explanation of isoxazolyl amino furo[3,2-c]quinolinone synthesis 7. | |
For the synthesis of the furan-2(5H)-one derivative 10 with indole as a structural fragment, Andrey's group established a facile and one-pot novel methodology, which was completed in two steps starting with the interaction of aryl glyoxal 1, indole 9, and Meldrum's acid 8 in acetonitrile with triethylamine at reflux, followed by a further acidic reflux using acetic acid (Scheme 5).46 The formation of the new oxygen heterocycle was considered to go through the aryl glyoxal 1 condensation with Meldrum's acid (intermediate) ca followed by the Michael addition to indole cb and finally cyclization with the elimination of CO2 to give 10 (Scheme 6).
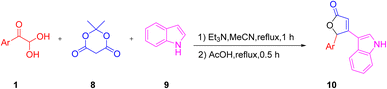 |
| Scheme 5 Three-component synthesis of furan-2(5H)-one derivative 10 using Meldrum's acid 8.46 | |
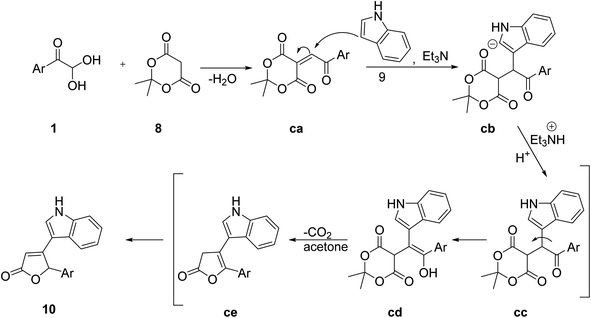 |
| Scheme 6 Mechanism to explain the synthesis of furan-2(5H)-one 10 using indole 9, Meldrum's acid 8, and aryl glyoxal 1 as starting materials. | |
Shahbazi-Alavi and co-workers demonstrated an environmentally friendly nanocatalyzed synthesis of substituted furans. The target molecule 13 was afforded through the heterogeneous catalysis of the aryl glyoxal 1, dimethyl acetylenedicarboxylate 11, and primary amine 12 reaction at room temperature by using the HPA-ZSM-5 nanocatalyst in dichloromethane (Scheme 7).47 The mechanistic pathway of the reaction is initiated by the nucleophilic attack of the amine lone pair 12 on the electrophilic site of dimethyl acetylenedicarboxylate 11 forming an enaminone, namely aminobutenedioate da. This aminobutenedioate acts as a C-nucleophile and attacks the electron-deficient carbon of phenyl glyoxal 1, thereby generating the second intermediate iminium-oxoanion db, which tautomerizes to the intermediate dc. Intermediate dc then undergoes γ-lactonization to give 5-oxo-2,5-dihydro-3-furancarboxylate 13 as the final product (Scheme 8). The low loading and reusability of the green catalyst for up to 6 cycles were particularly interesting aspects of this strategy.
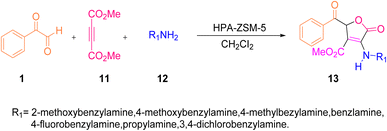 |
| Scheme 7 Nanocatalyzed protocol for the synthesis of substituted furans 13.47 | |
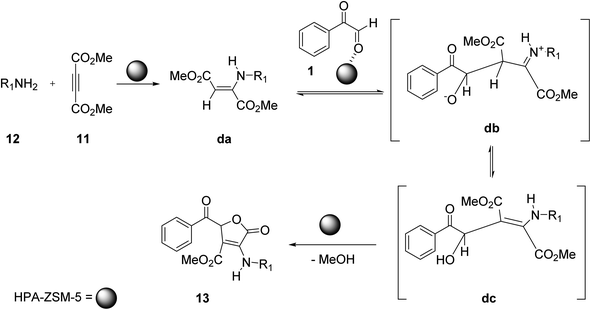 |
| Scheme 8 Mechanistic pathway to explain the nanocatalyzed synthesis of furan derivatives 13. | |
In 2021, Ebrahimi and co-workers synthesised 5-oxo-2,5-dihydro-3-furancarboxylate derivatives 13 by assembling aryl glyoxal 1, dimethyl acetylenedicarboxylate 11, and primary amines 12 in one-pot using nano-CuO at room temperature with dichloromethane as a reaction promoter. This work gained popularity due to its ease of operation, facile and quick extraction of the product, quick response time, high yield, and low loading and re-utilization of the catalyst (Scheme 9).48 The reaction design mimics the mechanism of HPA-ZSM-5 catalysis (Scheme 10).
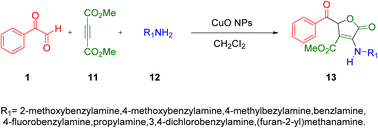 |
| Scheme 9 Three-component Cu nanoparticles-catalyzed synthesis of 5-oxo-2,5-dihydro-3-furancarboxylate derivatives 13.48 | |
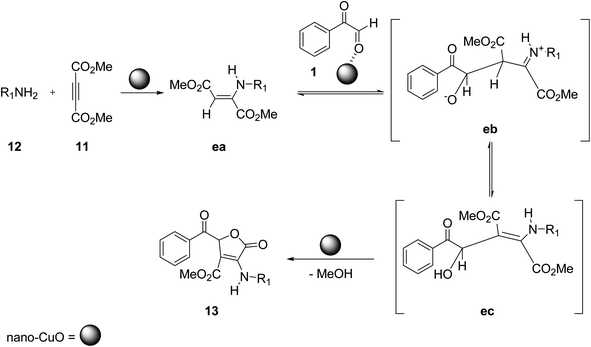 |
| Scheme 10 Mechanism that explains the synthesis of 5-oxo-2,5-dihydro-3-furancarboxylate 13 via Cu NPs. | |
Aldo-X reagents 1 and α-oxoketene dithioacetals 14 with indoles 15 as the precursors were utilized by Changhui's group to create six-multicomponent reactions, which were used to synthesize a variety of heterocycles, including quinolines, dihydrocoumarins, furans 16, and pyrroles 17. The discovery of these multicomponent reactions was made feasible by the coupling of two bifunctional aldo-X reagents and α-oxoketene dithioacetals, because these reagents contain a minimum of two reactive sites, which allows diverse substrates to be put together in different ways (Scheme 11).49
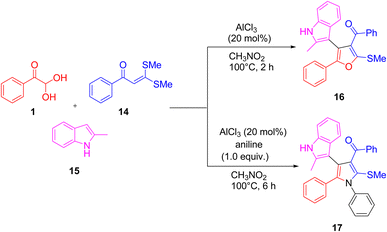 |
| Scheme 11 Syntheses of furan 16 and pyrrole 17 derivatives via three-component reactions.49 | |
Fatemah and co-workers applied aryl glyoxal 1 for the fabrication of 5-(furan-yl)barbiturate and 5-(furan-3-yl)thiobarbiturate 20 via a one-pot assembly of 1 with acetylacetone 18 and barbituric acid and thiobarbituric acid 19, respectively, in water at 60 °C (Scheme 12).50 The plausible mechanism starts with the Knoevenagel reaction between 1 and 18 to give the intermediate fa, which undergoes a 1,4-conjugated addition with barbiturate 19 yielding 1,4-diketone fb, followed by a Paal–Knorr cyclization fc to finally give 5-(furan-3-yl)barbiturate/thiobarbiturate 20 (Scheme 13).
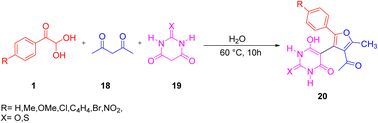 |
| Scheme 12 Three-component synthesis of 5-(furan-yl)barbiturate and 5-(furan-3-yl)thiobarbiturate 20 from acetylacetone 18 and barbituric acid 19.50 | |
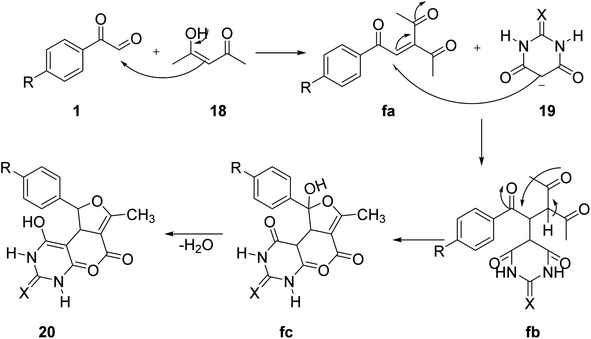 |
| Scheme 13 Mechanism proposed to explain the formation of 5-(furan-3-yl)thiobarbiturate 20. | |
2.2. Synthesis of benzofuran derivatives
In 2019, Ahmed and co-workers used aryl glyoxal for synthesizing functionalized benzofuran through an ionic liquid catalysis. The desired product 23 was obtained by the assembly of aryl glyoxal hydrate 1, sesamol 22, and indole 21 in an imidazolium-based Brønsted acid ionic liquid/butyl acetate green system. The above catalyst was recovered and used for further reactions (Scheme 14).51
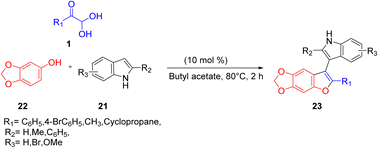 |
| Scheme 14 Synthesis of benzofuran 20 using sesamol 22 and indole 21 in a Brønsted acid system.51 | |
Further synthesis was done by Mahnaz Saraei's group using aryl glyoxal 1 as a building block for obtaining novel 4H-indeno[1,2-b]furan-4-ones 28 through a one-pot condensation with 2-aminopyridines 25 and 1,3-indandione 27 in water in the absence of any catalyst. In addition, by employing aryl glyoxal 1, 25, and barbituric acid 19 under the same green conditions, Khoeiniha et al. obtained furo[2,3-d]pyrimidine 29 derivatives in high yield (Scheme 15).52 The reaction was believed to start from the aldol condensation of 1 and 1,3-indanedione 27 generating the intermediate ga. Amine 25 was added to this intermediate through a Michael addition gb followed by intramolecular cyclization gc with subsequent dehydration to produce the final product 28. When 1,3-indandione was replaced with barbituric acid following the same methodology, a furo-pyrimidine derivative was obtained as the product (Scheme 16).
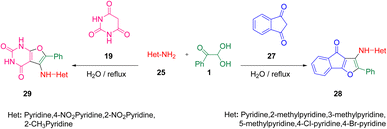 |
| Scheme 15 Three-component synthesis of 4H-indeno[1,2-b]furan-4-ones 28 via an aldol condensation.52 | |
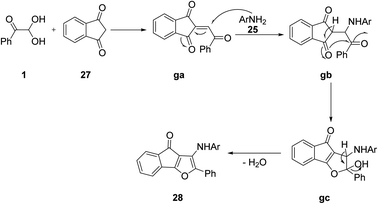 |
| Scheme 16 Plausible mechanism for the synthesis of 4H-indeno[1,2-b]furan-4-ones 28. | |
In 2020, Palanivel explored the combination of 1 and benzimidazole acetonitrile 30 with malononitrile 31 at room temperature in a trifilic acid/acetonitrile system to afford tricyclic aza-cyclopenta(cd)diindene 32. The above combination was also executed with benzoyl acetonitrile 35 at room temperature in ethanol/water to access the furo(2,3-b)furan derivative 36 in the presence of the organic base DABCO. Further, he obtained the pyrrolo-pyridine carboxamide 34 and furo-pyrrolo imidazole carboxamide 33 when the aryl glyoxal 1 was made to react with two equivalents of benzimidazole acetonitrile 30 in a pseudo-three-component reaction employing DABCO and NaOtBu as additives, respectively, in ethanol/water at room temperature (Scheme 17).36
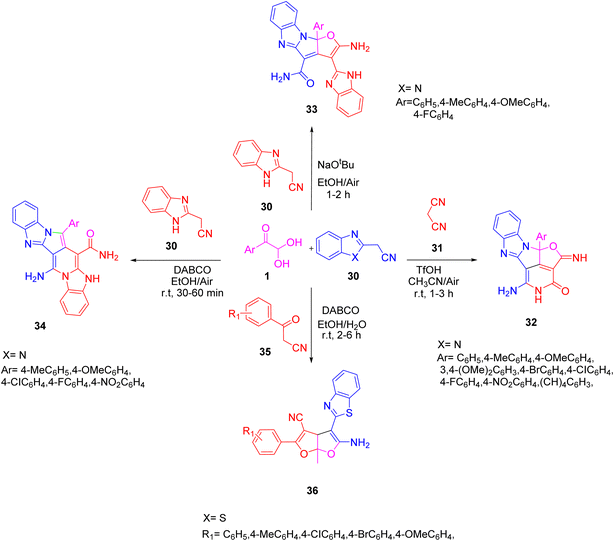 |
| Scheme 17 DABCO-catalyzed three-component reactions to yield various furo-furan derivatives 36, 32, and 33.36 | |
In 2020, Boris and co-workers effectively used aryl glyoxal for the preparation of terarylenes 39, a starting material for the synthesis of naphtho(1,2-b)benzofuran-7(8H)-ones 40 via a green photochemical rearrangement reaction of 4H-chromen-4-one derivatives. Aryl glyoxal 1 underwent a three-component tandem reaction with 3-(dimethylamino)-1-(2 hydroxyaryl)prop-2-en-1-one 37 and cyclic 1,3-diketone 38 in the presence of an inert solvent and base at room temperature followed by the subsequent addition of a hydrochloric and acetic acid mixture in the last. The model reaction was optimized by using different solvents and varying the system temperature. Terarylenes were best obtained in a good yield at room temperature with acetonitrile and triethylamine as the solvent/base system. The limitation of the novel multicomponent methodology to cyclic diketones was also confirmed by the unsuccessful test reaction of 2,4-pentanedione (Scheme 18).53 The plausible mechanism for this reaction includes the expected base-catalyzed condensation reaction of 1,3-diketone 38 with 1 followed by the subsequent addition of enaminone 37 to the generated Michael acceptor, forming the adduct hb. Intramolecular cyclization through nucleophilic attack of the hydroxyl group to the iminium ion generates an intermediate hc, which finally undergoes acid-catalyzed dehydration to form the desired product 39 with a new furan moiety in the structure (Scheme 19).
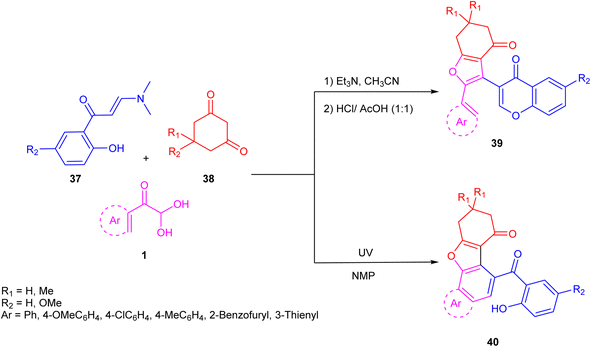 |
| Scheme 18 Syntheses of naphtho(1,2-b)benzofuran-7(8H)-ones and terarylene 39/40 via photochemical rearrangement reactions of 4H-chromen-4-one derivative.53 | |
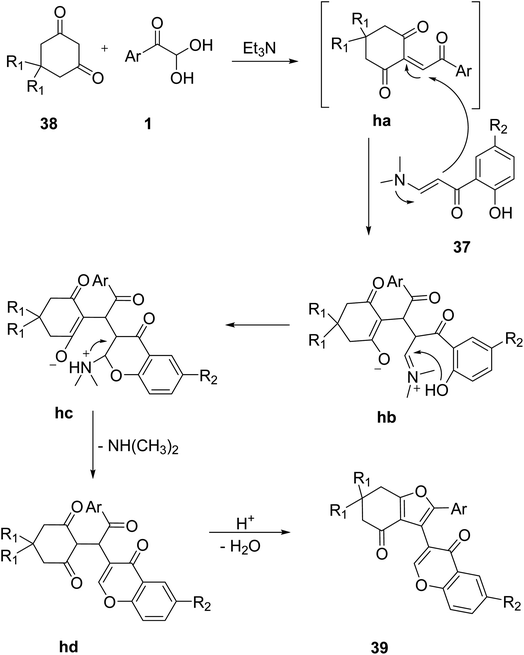 |
| Scheme 19 Mechanistic pathway representing the synthesis of naphtho(1,2-b)benzofuran-7(8H)-ones 39 via an acid-catalyzed dehydration. | |
Recently, P. et al. successfully demonstrated a three-component calcium-catalyzed approach for obtaining 3-aminofurans 43 in good yield via annulation of the in situ intermediate C, N-diacyliminium generated as a result of a three-component reaction between a variety of aryl glyoxal 1 and lactams 41 with 2-naphthols 42 under solvent-free conditions at 100 °C (Scheme 20).54 The proposed mechanism includes formation of the Ca2+-catalyzed imine ia in the initial step followed by ligand metathesis between Ca2+ and the hydroxyl group of β-naphthol to form a C–C bond ic, and then an intermediate id with a new C–O bond. Finally, id undergoes aromatization yielding the naphthofuran 43 (Scheme 21). The reaction was also tested against different solvents and Lewis acid catalysts, but none of them gave a yield of more than 50%. The ligand metathesis between Ca(OTf)2 and Bu4NPF6 increases the acidity of the catalyst by making it more efficient to provide an excellent yield. Interestingly, they also repeated the same protocol by changing the nucleophile source to mequinol (substituted phenol) 44 to access the corresponding benzofuran 45 and later glyoxal with the 9H-fluoren-3-ol derivative 46 and lactam-furnished fluorenofuran 47 in a good yield of 84%. Further, the annulation reaction of glyoxal and lactam with 4-hydroxycoumarin as a nucleophile did not prove efficient under solvent-free conditions. The products were studied for their photophysical properties and a broad substrate scope was established for all of these protocols.
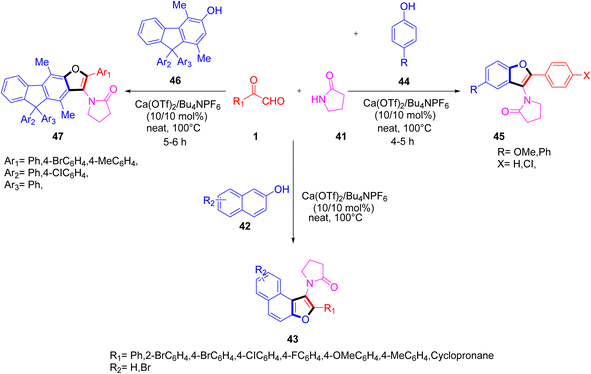 |
| Scheme 20 Three-component calcium-catalyzed protocol for the syntheses of 3-aminofurans 43, fluorenofuran 47, and benzofuran 45.54 | |
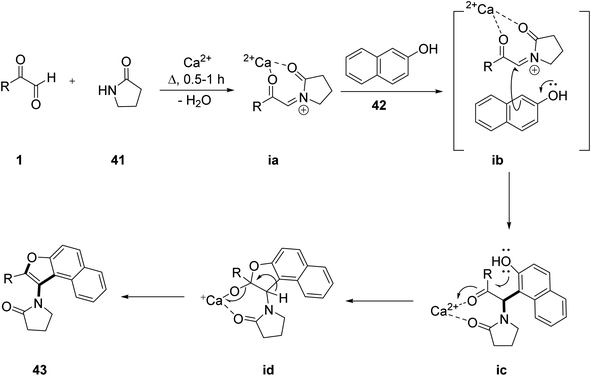 |
| Scheme 21 Plausible mechanism for the calcium-catalyzed synthesis of naphthofuran 43. | |
The utility of aryl glyoxal as a synthetic building block was explored by Lichitsky et al. through the triethylamine-promoted reaction of the 4-methoxy derivative of 1 with Meldrum's acid 8 and 8-hydroxyquinoline 48 in acetonitrile followed by cyclization in refluxing acetic acid to afford furylacetic acid 49. The protocol is marked by a simple work-up route and high atom economy (Scheme 22).55
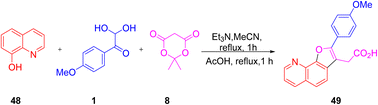 |
| Scheme 22 Synthesis of furylacetic acid 49 using Meldrum's acid 8 and 8-hydroxyquinoline 48.55 | |
In 2021, Komogortsev and co-workers performed a reaction involving 1, Meldrum's acid 8, and colchiceine 50, which led to a practical one-pot method to synthesize colchicine derivatives 51 with different substitutions. One of the distinctive aspects of the method was the production of the 6,7-dihydrobenzo[9,10] heptaleno[3,2-b]furan-9(5H)-one 51 molecule. Some of the key features of this method include the use of easily available precursors, facile execution of the protocol, and the ease with which the target products could be separated. The two-dimensional (2D) NMR spectrum verified the structure of one of the furotropolone products (Scheme 23).56
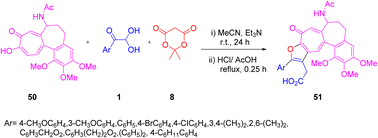 |
| Scheme 23 Synthesis of 6,7-dihydrobenzo[9,10] heptaleno[3,2-b]furan-9(5H)-one 51 using Meldrum's acid 8 and colchiceine 50.56 | |
A multicomponent reaction protocol was developed by Yang's group for the formation of 2-aryl-3-aminobenzofuran 53 derivatives by reacting aryl glyoxal monohydrates 1, phenols 44, and para-toluenesulfonamide 52, catalyzed by 10mol% of indium trichloride in the presence of dichloromethane as the solvent to obtain excellent yield (Scheme 24).57
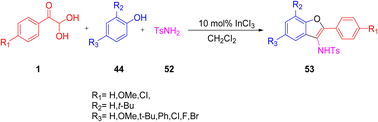 |
| Scheme 24 Indium-catalyzed multicomponent protocol for the synthesis of 2-aryl-3-aminobenzofuran 53.57 | |
Mosslemin's group in 2009 reported the isocyanide-based formation of furo[3,2-c]chromen-ones 56 through a condensation–cycloaddition of the easily available 4-hydroxy coumarin 54 with the extensively used aryl glyoxal and alkyl isocyanides 55 in neutral acetonitrile medium. The scheme also gave the same result when 4-hydroxycoumarin was substituted with dimethyl cyclohexandione (Scheme 25).58 The underlying mechanism for the synthesis begins with the generation of the Knoevenagel adduct ja of 4-hydoxycoumarin 54 and 1 with a subsequent [4 + 1] cycloaddition reaction between the resulting hetero-diene and isocyanide to form iminolactone jb as an intermediate. Lastly, a [1,3]-H shift in this iminolactone leads to furo[3,2-c]coumarin 56 (Scheme 26).
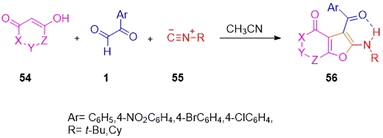 |
| Scheme 25 Synthesis of furo[3,2-c]coumarin 56 via a [4 + 1] cycloaddition reaction.58 | |
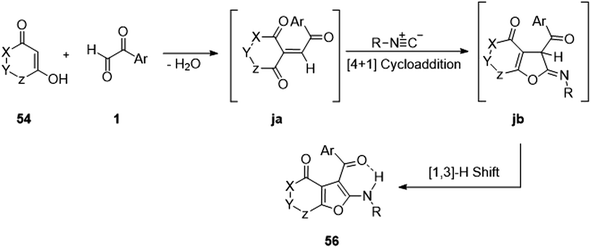 |
| Scheme 26 Mechanism to explain the synthesis of furo[3,2-c]coumarin 56 via a cycloaddition reaction. | |
The application of aryl glyoxal was demonstrated by Khodabakhshi and Hashemi in the construction of 2-aryl-3-benzamido-benzofurans 58 via a solvent-free three-component condensation reaction of 1, benzamide 57, and the phenolic substrate 44 with a catalytic amount of tungstate sulfuric acid (TSA) at 120 °C (Scheme 27).59 The plausible mechanism for the formation of benzofuran consisted of three steps, starting with the generation of the intermediate ka through an in situ condensation of amide 57 and 1, followed by the regio-specific formation of the oxygen-containing five-membered ring kc via the intramolecular cyclization of kb, and finally dehydration leading to the final product 58 (Scheme 28).
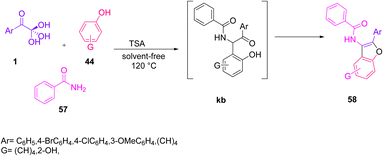 |
| Scheme 27 Three-component solvent-free TSA-catalyzed protocol for the synthesis of 2-aryl-3-benzamido-benzofuran 58.59 | |
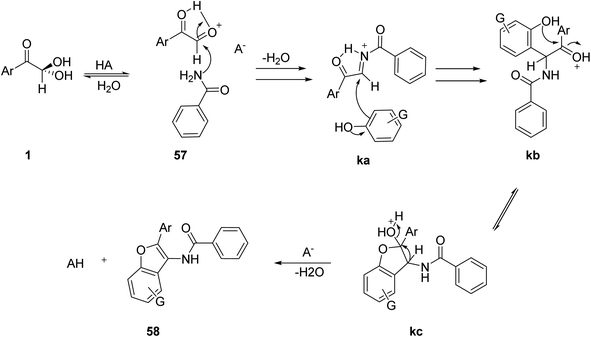 |
| Scheme 28 Mechanistic pathway representing the synthesis of 2-aryl-3-benzamido-benzofuran 58. | |
The use of aryl glyoxal 1 by Salari and co-workers set an efficient protocol for the synthesis of functionalized trans-tetrahydrobenzofuran-4-ones 60 via the condensation reaction of aryl glyoxal, N-(4-halophenacyl)-pyridinium bromide 59, and cyclic 1,3- diketone 8 utilizing DABCO in a catalytic amount with the evergreen solvent water under reflux. Further optimization of the protocol was accomplished by using different derivatives of aryl glyoxal, showing that p-nitroaryl glyoxal and o-bromoaryl glyoxal gave the highest yields under the same conditions (Scheme 29).60
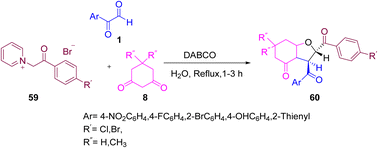 |
| Scheme 29 Three-component DABCO-catalyzed synthetic protocol for the synthesis of trans-tetrahydrobenzofuran-4-ones 60.60 | |
A pentacyclic product named as trans-(4-chloroAryl)-7-aryl-6,7-dihydro(1,3)dioxolo(4,5-f)(1)benzofuran-6-yl)methanones 60 was afforded by Selari et al., via a one-pot 1,4-diaza-bicyclo[2.2.2]octane (DABCO)-catalyzed condensation of three components, namely 2-(2-(4-chloroAryl)-2-oxoethyl))isoquinolinium bromide 59, benzo(1,3)dioxol-5-ol 22, and aryl glyoxal 1 in water at reflux. Further, a library of substrates was constructed by using different halo-, nitro-, and hydroxyl-substituted aryl glyoxals (Scheme 30).61
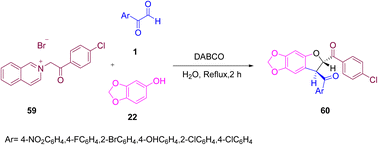 |
| Scheme 30 Synthesis of trans-(4-chloroAryl)-7-aryl-6,7-dihydro(1,3)dioxolo(4,5-f)(1)benzofuran-6-yl)methanones 60 in a one-pot approach using DABCO.61 | |
In 2020, Zhang et al. reported a simple and straightforward protocol for the quick synthesis of the benzofuran derivatives 63 and 64, which involved an FeCl3-mediated intermolecular tandem reaction between anisole 61 and 1, via a Friedel–Craft alkylation and oxidative annulation. This reaction offers a lot of benefits, like readily available starting materials, high atom economy, and strong functional group tolerance (Scheme 31).62 The mechanistic steps for the annulation reaction include the Friedel–Craft alkylation of 1 with anisole 61 forming the intermediate la, which in turn is oxidized by FeCl3 to generate the second radical cation intermediate lb. FeCl3 is then reduced second time to further oxidize lc with a subsequent intramolecular cyclization followed by dehydration to yield the annulated benzofuran 63 (Scheme 32).
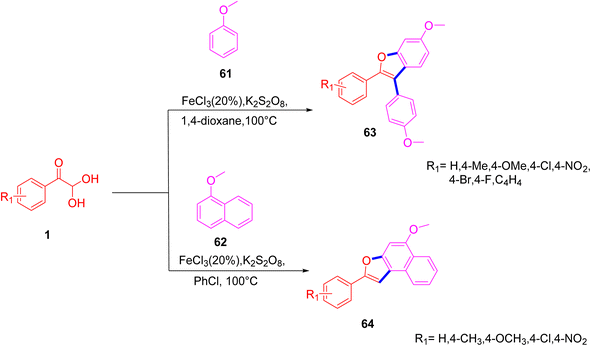 |
| Scheme 31 Syntheses of benzofurans 63/64 via FeCl3-mediated intermolecular tandem reactions.62 | |
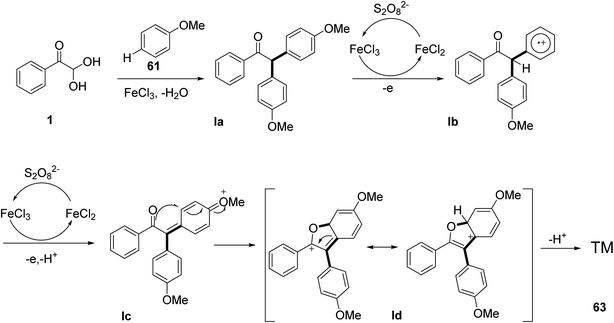 |
| Scheme 32 Plausible mechanism for the syntheses of benzofurans 63/64 in the presence of FeCl3. | |
By employing microwave irradiation in the presence of the H3PW12O40@Fe3O4–ZnO catalyst, Taheri and co-workers in 2021 developed an effective and convenient single-pot, three-component reaction (3CR) of 2-hydroxynaphthalene-1,4-dione 65, aryl glyoxal 1, and indoles 67 to produce benzo[a]furo[2,3-c]phenazine 68 derivatives with excellent yields. The facile and quick extraction of the products, short reaction time, mild reaction conditions, high product yield, solvent-free conditions, low energy demand, and economically affordable chemicals were the major advantages of this reaction protocol (Scheme 33).63
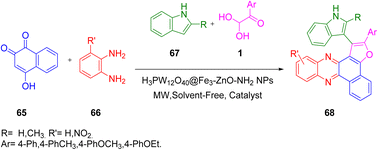 |
| Scheme 33 Synthesis of benzo[a]furo[2,3-c]phenazine 68 via microwave irradiation in the presence of H3PW12O40@Fe3O4–ZnO as a catalyst.63 | |
Taheri and colleagues performed the same reaction in 2021 to develop an easy, efficient, and straightforward protocol for the synthesis of benzo[a]furo[2,3-c]phenazine 68 derivatives in excellent yields by the reaction of benzo[a]phenazin-5-ol 65, 1, and 67 utilizing the Fe3O4@rGO@ZnO-HPA catalyst and keeping the other conditions similar to the previous reaction (Scheme 34).64
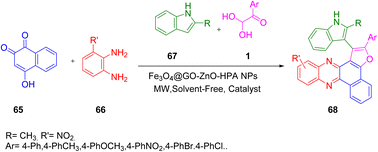 |
| Scheme 34 Synthesis of benzo[a]furo[2,3-c]phenazine 68 using Fe3O4@rGO@ZnO-HPA as a catalyst.64 | |
A one-pot multicomponent reaction leading to the synthesis of urea-substituted 2-arylfurans 71 was reported for the first time by Andrey N. Komogortsev's group by reacting numerous carbo and heterocyclic enols 69, cyanamide 70, and aryl glyoxal 1 under reflux conditions in the presence of the solvent acetonitrile and base triethylamine (Scheme 35).65
 |
| Scheme 35 One-pot protocol for the synthesis of urea-substituted 2-arylfurans 71 by employing cyanamide 70 and heterocyclic enols 69.65 | |
2.3. Synthesis of pyran derivatives
A green nanoparticles-catalyzed strategy for the production of dihydropyrano(c)chromenes 74 was demonstrated by Khodabakhshi's group using aryl glyoxal 1, 4-hydroxycoumarin 72, and malononitrile 73 in a single pot with an ethanol–water solvent system at reflux. The same reaction was tested against p-TSA, Na2CO3, FeCl3, ZnCl2, and AcOH and gave no significant yields of the desired products, except for the magnetically recyclable Fe3O4 nanoparticles (Scheme 36).66
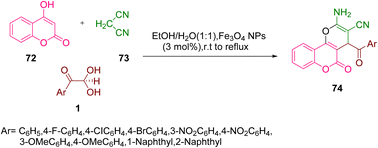 |
| Scheme 36 Nanocatalyzed synthesis of dihydropyrano(c)chromenes 74 by the reaction of aryl glyoxal 1 with 4-hydroxycoumarin 72, and malononitrile 73.66 | |
Again in 2014, Khodabakhshi and co-workers explored the use of nanosheets of graphene oxide with the same one-pot three-component combination under reflux in an ethanol/water system with a little amount of loading of GO catalyst. The advantages of the above protocol were marked by the recyclable catalyst with a minimal loading of 0.005 g with a high yield and simple work-up procedure (Scheme 37).67
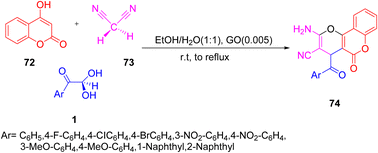 |
| Scheme 37 Graphene oxide-catalyzed synthesis of dihydropyrano(c)chromenes 74 by the reaction of aryl glyoxal 1 with 4- hydroxycoumarin 72 and malononitrile 73.67 | |
A synthetic library of multifunctional pyrano(c)chromenes 74 was developed by Saeed et al. by exploiting differently substituted aryl glyoxal 1 with malononitrile 73 and 4-hydroxycoumarin 72 in the presence of ammonium dihydrogen phosphate at room temperature, first for 30–40 min and then at reflux in an ethanol/water system to obtain the desired product (Scheme 38).68
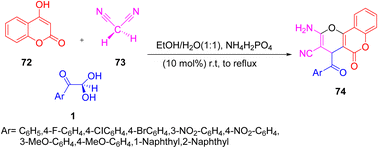 |
| Scheme 38 Pyrano(c)chromenes 74 synthesis in the presence of ammonium dihydrogen phosphate.68 | |
In 2015, a novel synthetic route was developed by Rimaz et al. to afford a library of biologically active substituted pyrano(2,3-d)pyrimidines 75. They obtained the desired products through an excess ammonium acetate-catalyzed one-pot condensation of aryl glyoxal monohydrate 1 with barbituric acid 19 using the greenest solvent water at room temperature (Scheme 39).69
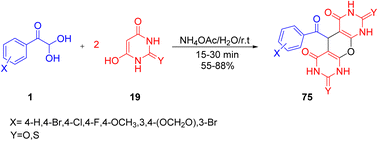 |
| Scheme 39 Synthesis of pyrano(c)chromenes 75 via ammonium acetate-catalyzed reaction in the presence of a green solvent.69 | |
Rimaz and co-workers reported the synthesis of pyrano-fused pyrimidines derivatives 75 by the regioselective pseudo-three-component condensation reaction of aryl glyoxal monohydrate 1 and 1-ethyl-2-thioxodihydropyrimidine-4,6(1H,5H)-dione 19 under a catalytic system employing DABCO or ZrOCl2·8H2O in ethanol at 50 °C. The pyrano-fused-pyrimidine scaffold in the synthesized compound was identified as possessing HIV integrase inhibitor activity (Scheme 40).35
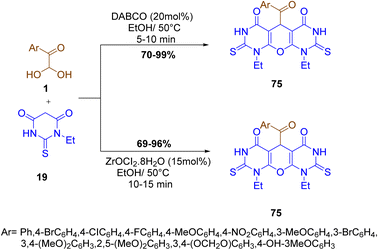 |
| Scheme 40 Pseudo-three-component reaction to yield the pyrano-fused pyrimidines derivative 75 under DABCO or ZrOCl2·8H2O catalysis.35 | |
The potassium phthalimide-N-oxyl (PPINO) organocatalyzed one-pot green reaction of aryl glyoxal monohydrate 1 with barbituric acid 19 or thiobarbituric acid and β-naphthol 42 at reflux in water yielded benzo[5,6]chromene 76 and derivatives was single-handedly reported by Etivand. The distinguishing features of the protocol as highlighted were the use of green solvents, short reaction time of only 30 min, and the product contained more than one heterocycle centre (Scheme 41).70
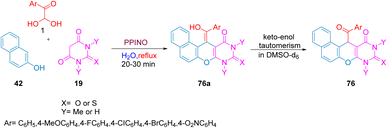 |
| Scheme 41 Organocatalyzed reaction of (PPINO) to yield benzo[5,6]chromene 76 using a green solvent.70 | |
A convenient method for the synthesis of a novel series of 2-amino-4-aroyl-4H-benzo[h]chromene-3-carbonitriles 78 was developed by Marjani et al. under solvent-free microwave (MW) conditions, in high yields by using a multicomponent condensation reaction of aryl glyoxals 1, 1-naphthol 77, and malononitrile 73 in the presence of Mg–Al hydrotalcite (Scheme 42).71 A plausible outline of the scheme is starting from the Mg–Al hydrotalcite accelerated Knoevenagel reaction of 1 with C–H acid 73 to form an intermediate, namely 2-(2-oxo-2-arylethylidene)malononitrile ma. In the second step, α-naphthol is added as a C-nucleophile to ma, forming a new species with the C–C bond mb. The intermediate mc undergoes intramolecular cyclization to generate the species md, which tautomerizes to give the product 78 (Scheme 43).
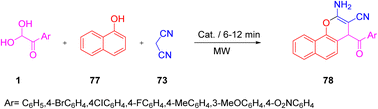 |
| Scheme 42 Microwave-assisted reaction to afford 2-amino-4-aroyl-4H-benzo[h]chromene-3-carbonitriles 78 using Mg–Al hydrotalcite.71 | |
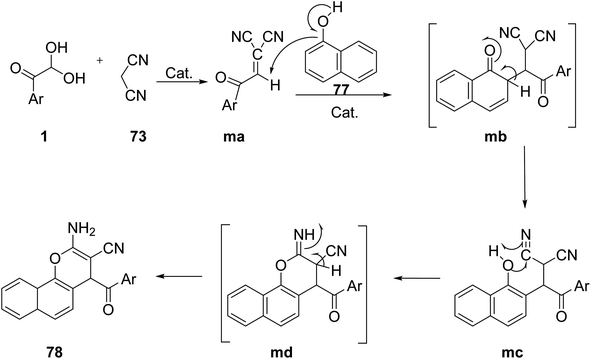 |
| Scheme 43 Mechanism for the formation of 2-amino-4-aroyl-4H-benzo[h]chromene-3-carbonitrile 78. | |
Mishra and Choudhury investigated the possibility of using microwave irradiation as one of the synthetic routes with the assembly of aryl glyoxal monohydrate 1 along with malononitrile 73 and cyclic 1,3-dicarbonyls 79 to afford several pyrans fused with many functionalities, such as coumarins, quinolones, naphthoquinones, and pyrones 80. This approach is a basic and simple way of obtaining functionalized pyrans without the need for a catalyst or column chromatographic purification (Scheme 44).72
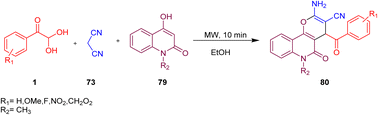 |
| Scheme 44 Microwave-assisted protocol for the synthesis of fused pyrone 80.72 | |
In addition, Mishra and Choudhury also explored the scope of this technique to obtain different fused pyrans 74 and 74a by changing 1,3-dicarbonyls to other cyclic functionalities, such as 4-hydroxy coumarin 72 and 4-hydroxy-6-methyl-2H-pyran-2-one 72a. The corresponding products were isolated in high yields (Scheme 45).72 The reaction pathway is outlined in Scheme 46.
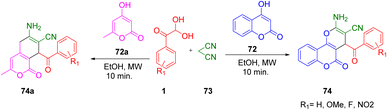 |
| Scheme 45 Synthesis of fused pyrans 74/74a using a microwave technique in the presence of ethanol.72 | |
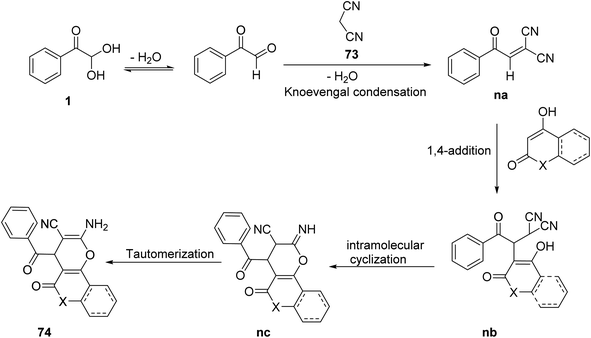 |
| Scheme 46 Reaction pathway for the formation of fused pyran 74. | |
Furthermore, Khalafy's group reported the nanocatalyzed synthesis of 2-amino-4-aroyl-5-oxo-5,6-dihydro-2H-pyrano[3,2-c] 80 via the reaction of aryl glyoxal 1, active methylene group 73, and 4-hydroxyquinolin-2(1H)-one 79 in a single-pot approach with the SBA-15 nanocatalyst in a green solvent. The simplicity of the work-up procedure, the use of an ethanol/water system as a green medium, and the good to extraordinary product yields represent the key benefits of this synthetic technique (Scheme 47).73
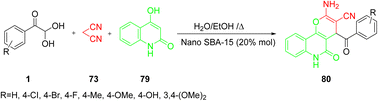 |
| Scheme 47 SBA-15-catalyzed green synthesis of 2-amino-4-aroyl-5-oxo-5,6-dihydro-2H-pyrano[3,2-c] 80.73 | |
Khalafy et al. extended the library of oxygen heterocycles in 2018 through 4-aroyl-4H-benzo[g]chromene 82 synthesis using 1, 2-hydroxy-1,4-naphtoquinone(lawsone) 81, and one active methylene species 73 with the effective use of a Zn(L-Pr)2 metal–amino acid complex. The Zn(L-Pr)2 is a water-soluble catalyst that shows Lewis acid behaviour together with significant reusability. The protocol was also tested with different acid catalysts, namely sulfanilic acid, p-toluenesulfonic acid, the phase-transfer catalyst tetrabutylammonium bromide, and L-cystein, obtaining the target molecule 82 in high yields with 20% L-proline in ethanol/water at 50 °C (Scheme 48).74
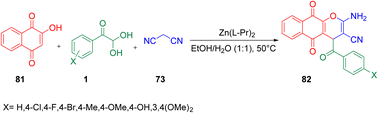 |
| Scheme 48 Three-component synthesis of 4-aroyl-4H-benzo[g]chromene 82.74 | |
Next, in 2018, Nasri et al. successfully employed aryl glyoxal to obtain the biologically important oxygen heterocycle and useful chemical synthon chromene. A chemical library of pyrano[3,2-c]chromene 74 and benzo[g]chromene 82 was constructed through a catalyst-free one-pot assembly of aryl glyoxal monohydrate 1, malononitrile as the active methylene group 73, and 4-hydroxycoumarin 72/2-hydroxy 1,4-nephthaquinone 81 under reflux with ethanol as the solvent. Ethyl cyanoacetate and methyl cyanoacetate and cyanoacetamide favoured the enol product 83 (Scheme 49).75 This assembly was also considered to follow the same mechanistic pathway as explained in the case of other equivalent functionalities starting from Knoevenagel condensation to form a Michael acceptor oa followed by 1–4 addition, thereby generating the open chain intermediates ob and od, which subsequently undergo cyclization intramolecularly to yield the desired products (Scheme 50).
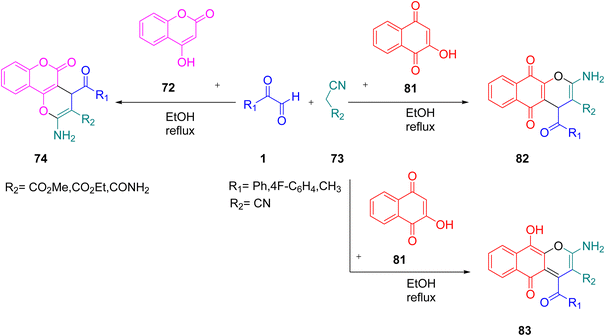 |
| Scheme 49 MCR-based synthesis of the different pyran derivatives 74, 82, and 83.75 | |
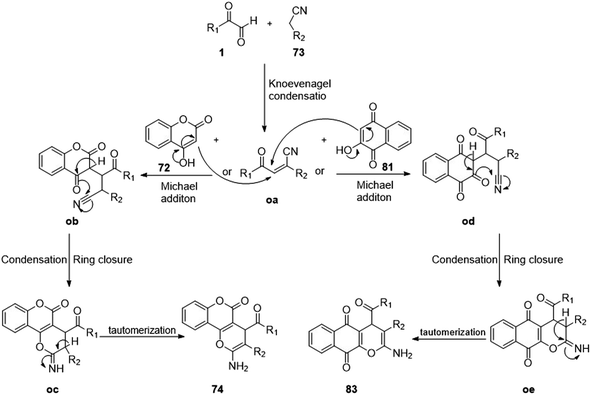 |
| Scheme 50 Mechanisms for 74 and 83 formation through Knoevenagel condensation with a subsequent intramolecular cyclization. | |
Khaligh in 2017 synthesized 2-amino-4H-benzo[g]chromenes 82 using the same tactic of a condensation–Michael addition reaction by stirring aryl glyoxal 1, malononitrile 73, and 2 hydroxynephthaquinone 81 with the catalyst poly(N-vinylimidazole), i.e. PVIm, for 2 h in refluxing ethanol. The resulting product showed antibacterial activity against Escherichia coli at 32 mg cm−3 (Scheme 51).27
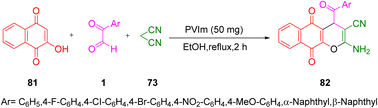 |
| Scheme 51 Poly(N-vinylimidazole)-catalyzed synthesis of 2-amino-4H-benzo[g]chromenes 82.27 | |
In 2014, Khodbakhshi's group successfully executed the three-component reaction of 4-hydroxycoumarin 72, aryl glyoxal 1, and malononitrile 73 to access pyrano[3,2-c]coumarins 74 with an aroyl group in excellent yield with a high degree of purity in the presence of Mohr's salt. The overall reaction process was simple, facile, atom economical, and environmentally beneficial (Scheme 52).76
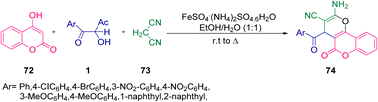 |
| Scheme 52 Three-component synthesis of pyrano[3,2-c]coumarins 74.76 | |
In the same year, Khodbakhshi's group repeated a similar three-component reaction protocol but replacing Mohr's salt with TiO2 nanoparticles. Their process involved the coupling of 1 with malononitrile 73 and 72 in the presence of a catalytic amount of TiO2 nanoparticles to give a novel family of pyranochromenes 74 in excellent yield. Their reaction was both environmentally feasible and economically cost effective since it utilized a greener solvent, and reusable and safer catalyst (Scheme 53).77
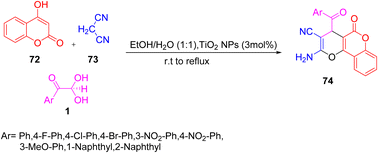 |
| Scheme 53 TiO2 nanoparticles-catalyzed synthesis of pyrano[3,2-c]coumarins 74.77 | |
Marjani and co-workers revealed the synthetic utility of aryl glyoxal by bringing together substituted aryl glyoxal 1 in a reaction vessel with 4-hydroxyquinolin-2(1H)-one 79 and ethyl cyanoacetate 84 in the presence of the catalyst TPAB in a water/ethanol system at reflux. This assembly yielded a series of ethyl 2-amino-4-benzoyl-5-oxo-5,6-dihydro-4H-pyrano[3,2-c]quinoline-3-carboxylate 85 compounds (Scheme 54).78
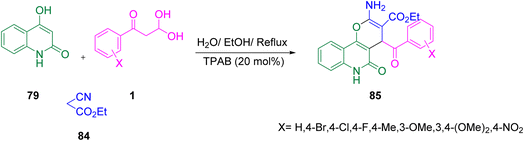 |
| Scheme 54 Synthesis of ethyl 2-amino-4-benzoyl-5-oxo-5,6-dihydro-4H-pyrano[3,2-c]quinoline-3-carboxylate 85 through 4-hydroxyquinoline-2(1H)-one.78 | |
Aryl glyoxal monohydrate as the same starting material was explored by Ahmad et al. in 2018, with malononitrile 73 and 1,3-diketones 38 in the presence of the catalyst L-proline in ethanol solvent for the facile construction of 4H-chromenes, namely 2-amino-4-aroyl-5-oxo-5,6,7,8-tetrahydro-4H-chromene-3-carbonitrile 86 (Scheme 55).79
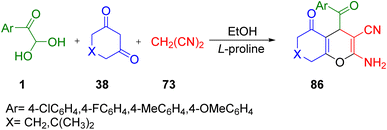 |
| Scheme 55 Formation of 2-amino-4-aroyl-5-oxo-5,6,7,8-tetrahydro-4H-chromene-3-carbonitrile 86.79 | |
M. Taheri and R. Mohebat in 2020 proposed a unique greener and environmentally friendly one-pot four-component protocol for the synthesis of pyrazolo[4′,3′:5,6]pyrano[2,3-c]phenazin-15-yl)methanone 88 scaffolds in a solvent-free medium using nano Fe3O4@TiO2–SO3H as the catalyst under microwave monitoring at 75 °C. The major benefit of this green synthetic protocol was the mild reaction conditions, high product yield, fast reaction time, solvent-free condition, low energy demand, and economic affordability (Scheme 56).80 The underlying mechanism for the following protocol starts from the tautomerization of 2-hydroxy-1,4-naphthalene-1,4-dione 65 to form an intermediate, which then undergoes condensation with benzene-1,2-diamine 66 resulting in the formation of benzo[a]phenazine-5-ol pa. After this, Fe3O4@TiO2–SO3H catalyzes the formation of pyrazolo[4′,3’:5,6]pyrano[2,3-c]phenazine-15-yl) pd, which after Michael addition with 1 undergoes cyclization–dehydration to finally give the desired product 88 (Scheme 57).
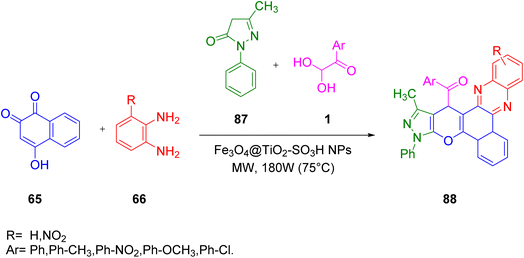 |
| Scheme 56 Nano Fe3O4@TiO2–SO3H-catalyzed formation of pyrazolo[4′,3′:5,6]pyrano[2,3-c]phenazin-15-yl)methanone 88.80 | |
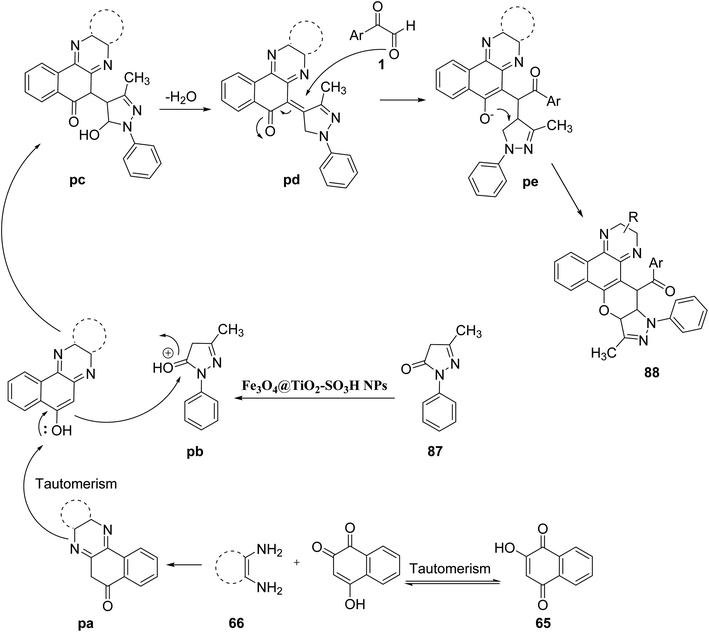 |
| Scheme 57 Mechanism for the formation of 88. | |
2.4. Synthesis of furo-pyran
In 2020, Boris' group explored a new one-pot protocol for the synthesis of various substituted 2-aminofuran moieties 90 based on the multicomponent reaction of 3-hydroxy-4H-pyran-4-ones 89, a-ketoaldehydes 1, and methylene active nitriles 73. The formation of 2-aminofuran was a distinguishing aspect of this protocol in contrast to the previously reported literature, which resulted in the formation of 2-aminopyrans. The excellent yield, high atom economy, simple work-up procedure, and maintenance of mild reaction conditions for the reaction to proceed were all major benefits of this protocol (Scheme 58).81 The suggested mechanism (Scheme 59) begins with the Michael acceptor generation from the C–H active site of malononitrile 73 reaction with 1. The allomaltol anion (deprotonated by triethylamine) adds to the intermediate, thus forming an adduct qa. This adduct undergoes deprotonation to form an oxoanion qb followed by cyclization at the nitriles qc, finally leading to furo-pyran as the final product 90.
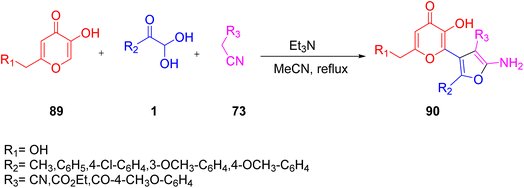 |
| Scheme 58 3-Hydroxy-4H-pyran-4-ones-derived synthesis of 2-aminofuran 90.81 | |
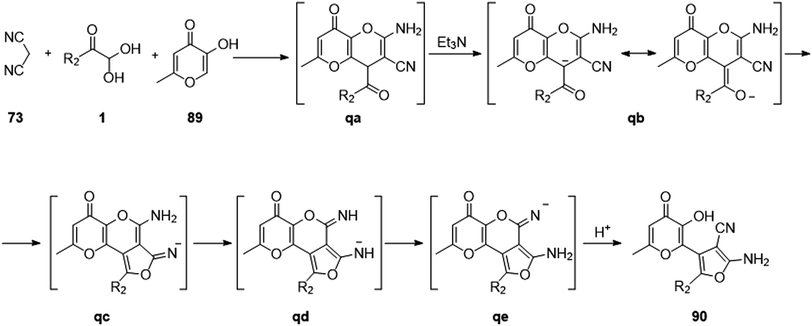 |
| Scheme 59 Synthesis of 2-aminofuran through a Michael reaction followed by cyclization. | |
In 2021, Mirza and co-workers proposed a methodology for the synthesis of a novel, green, and highly efficient β-amido-aroyl carbonyl derivatives 91 via a three-component, one-pot reaction of dimedone 38/barbituric acid 19 derivatives, aryl glyoxal 1, and amides 57a in a deep eutectic solvent of choline chloride/urea (DES). The utilization of biodegradable ingredients, rapid reaction times, and high product yields established this process as effective and ecologically friendly (Scheme 60).82
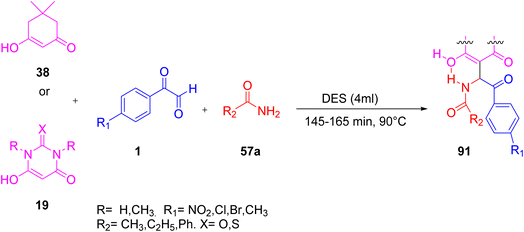 |
| Scheme 60 Synthesis of β-amido-aroyl carbonyl 91 in a deep eutectic solvent.82 | |
In 2013, Karami and co-workers regiospecifically synthesized amido-substituted furo[4,5-c] coumarins 92 by reacting aryl glyoxal, 4-hydroxy coumarin 72, and benzamide 57 in a single pot through coupling, followed by cyclization in acetic acid at reflux. The product showed selectivity over isoxazolo-substituted coumarin. The plausible mechanism for the reaction started from the condensation of 1 and 57 to generate an intermediate, followed by its intramolecular cyclization and then dehydration to give the final product (Scheme 61).83
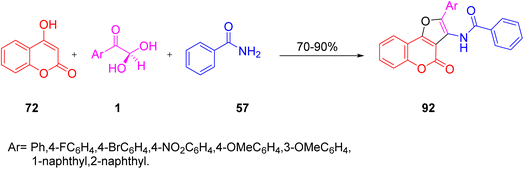 |
| Scheme 61 Benzamide 57-derived amido-substituted furo[4,5-c]coumarin 92 synthesis.83 | |
Huang and co-workers reported an efficient Lewis acid-catalyzed reaction for synthesizing diverse furo[3,2-c]coumarins 93 and 94 by exploiting aryl glyoxal, 4-hydroxycoumarins 72, and methylketone in 1,4-dioxane at 130 °C using 20mol% Zn(OTf)2. The substrate scope of the protocol showed that both electron-rich and electron-neutral aryl glyoxal favoured an annulation reaction, while that with an electron-withdrawing group, such as the NO2-bearing aryl ring of aryl glyoxal, did not yield any product. When the strategy was extended by involving aliphatic cyclic ketones, like cyclochexanone, to diversify the products, the use of Cu(OTf)2 in the solvent dichloroethane (DCE) at 110 °C in a sealed vessel gave the best results, with a 76% yield (Scheme 62).84 The proposed reaction design (Scheme 63) follows the pathway initially from the loss of a water molecule through the condensation reaction of 1 and 72 to give the intermediate ra, which reacts with the enol form of acetone in a Michael-type addition to produce the second intermediate rb. The electrophilicity of the condensed adduct ra is enhanced by the Zn(OTf)2. Finally, dehydration preceded by an intramolecular cyclization leads to the formation of furo[3,2-c]coumarin 93.
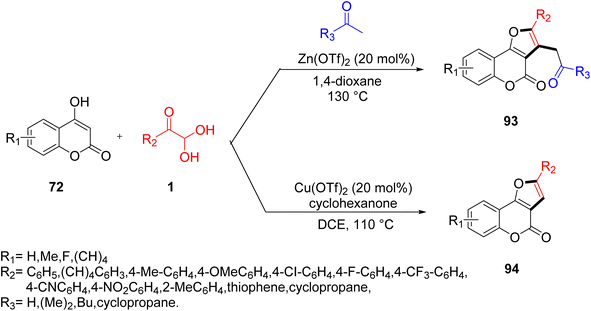 |
| Scheme 62 Lewis acid-catalyzed protocols for yielding furo[3,2-c]coumarins 93 and 94.84 | |
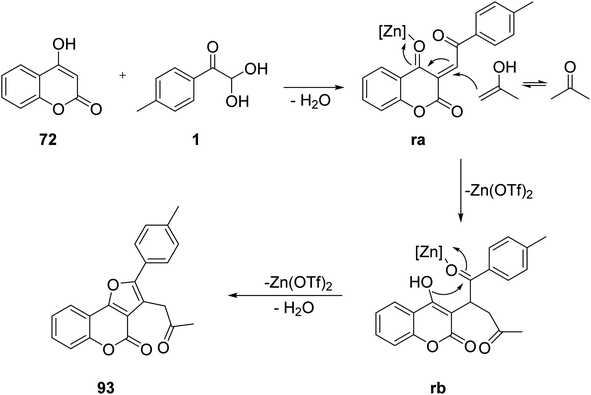 |
| Scheme 63 Mechanism showing furo[3,2-c]coumarin synthesis through a condensation reaction followed by an intramolecular cyclization. | |
Next, a facile and one-pot novel methodology was described by Melekhina's group for the synthesis of furan-2(5H)-one derivative 10 with indole 9 as a structural fragment. The reaction was carried out in two steps, starting with the interaction of 4-methoxy aryl glyoxal 1, indole, and Meldrum's acid 8 in acetonitrile with triethylamine at reflux, followed by a further acidic reflux using acetic acid. The formation of a new oxygen heterocycle was considered to occur through the aryl glyoxal condensation with Meldrum's acid followed by Michael addition to give the indole and finally cyclization occurs with the elimination of CO2 (Scheme 64).46
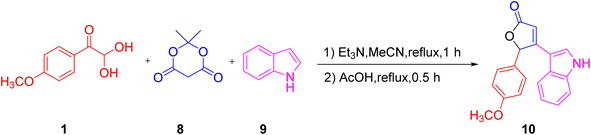 |
| Scheme 64 Furan-2(5H)-one derivative 10 synthesized using 4-methoxy aryl glyoxal 1, indole 9, and Meldrum's acid 8.46 | |
After this, Boris et al. replaced the indole with hydroxycoumarin derivative 95 to access the novel furylacetic acid moiety 96. The pronounced advantage offered by the use of the photosensitizer and the biologically important furo-coumarin derivative synthesis is that the formation was possible through a single-pot approach using easily available staring materials with no harsh reaction conditions (Scheme 65).85
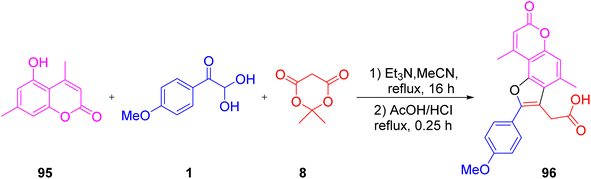 |
| Scheme 65 Formation of the hydroxycoumarin 95-derived furylacetic acid moiety 96.85 | |
In 2021, Lichitsky and co-workers described a simple one-pot approach for synthesizing a regiospecific 4H-furo[2,3-h]chromene 98 core using aryl glyoxal. The proposed method was based on a multicomponent reaction of aryl glyoxal, flavones 97, and Meldrum's acid 8. The mild reaction conditions, atom economy, and simple work-up procedure were all advantages of this method, which eliminated the need for chromatographic purification (Scheme 66).86
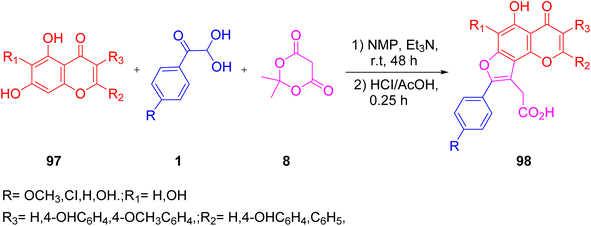 |
| Scheme 66 Regiospecific synthesis of 4H-furo[2,3-h]chromene 98 through flavone 97, Meldrum's acid 8, and aryl glyoxal 1.86 | |
In 2018, Chang et al. reported the formation of the highly functionalized furan derivatives 99 and 100 via aryl glyoxal, phenols 44, and 4-hydroxycoumarin 72 in the presence of MeSO3H or FeCl3 as a catalyst. By altering the reaction media, a range of furan derivatives with various substitution patterns were produced. This method's atom-economical traits and moderate conditions were consistent with the idea of contemporary green chemistry. In a short time, a substantial number of heterocycles of biological importance were created from this protocol (Scheme 67).87
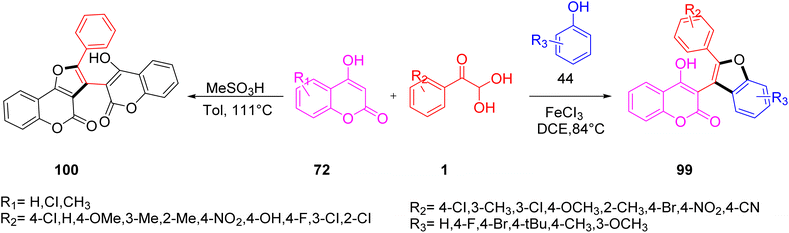 |
| Scheme 67 Synthesis of 4-hydroxycoumarin 72-derived functionalized furans 99 and 100.87 | |
Chang et al. developed an effective method for synthesising furo[3,2-c]-coumarins utilizing 1 in multicomponent tandem reactions driven by FeCl3 or ZnCl2. As per the reports, this reaction between 4-hydroxycoumarin 72 and allyltrimethylsilane 101 in toluene produced two C–C bonds and one C–O bond in compounds 103 and 104. This approach provided the desired furo[3,2-c]coumarin structures in good to outstanding yields. Some notable traits of the method include the ease with which the starting materials may be obtained, the great functional group tolerance, and the outstanding atom economy (Scheme 68).88
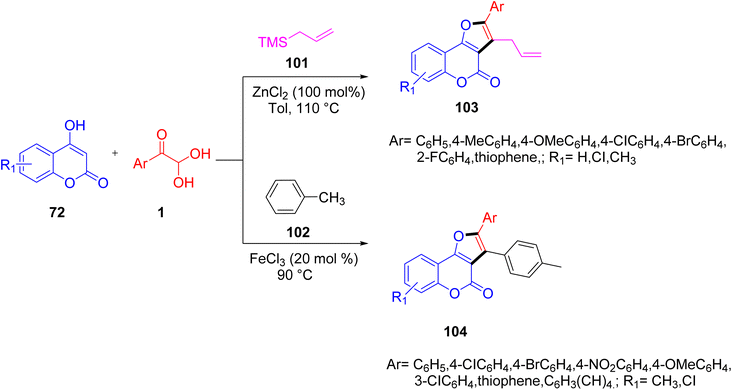 |
| Scheme 68 Tandem reactions leading to diverse furo[3,2-c] compounds 103 and 104 using allyltrimethylsilane 101 and toluene 102, respectively.88 | |
Next in 2019, the synthetic application of aryl glyoxal was further explored to construct novel moieties of biological importance. Komogortsev et al. devised a new convenient method to obtain 7-oxo-7H-furo[3,2-b]pyran-3-ylacetic acid 106 through the reaction of pyranone 105, Meldrum's acid 8, and aryl glyoxal 1 as a carbonyl compound in one pot. The reaction was accomplished by the catalysis of Et3N in the presence of MeCN at reflux, starting from the condensation of 1 with Meldrum's salt, thus setting the intermediate sa possessing an aroyl fragment as the starting material for the recyclization in acidic medium to form the substituted furan-3-acetic acid 106 (Scheme 69).89 The distinguishing feature of the protocol was the application of the Kojic acid analogue 3 hydroxy-pyran-4-one 105.
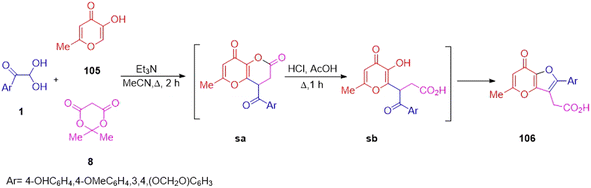 |
| Scheme 69 Synthesis of 7-oxo-7H-furo[3,2-b]pyran-3-ylacetic acid 106 through a condensation reaction.89 | |
Lichitsky et al. in 2020 developed a systematic telescopic protocol to synthesize substituted furan 2(5H)-one derivatives containing the 4H-chromen-4-one fragment via the multicomponent reaction of 3-(dimethylamino)-1-(2-hydroxyaryl)prop-2-en-1-one 37, aryl glyoxal 1, and Meldrum's acid 8. The simultaneous production of 4H-chromen-4-one and furan-2(5H)-one fragments 107 in one synthetic stage was a distinguishing aspect of the suggested methodology. This approach was also atom economical along with having other virtues, such as mild reaction conditions and a simple work-up approach, which eliminates the need for chromatographic purification (Scheme 70).90
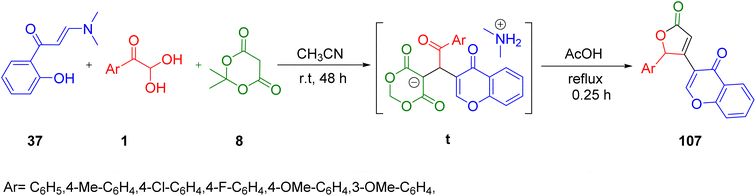 |
| Scheme 70 3-(Dimethylamino)-1-(2-hydroxyaryl)prop-2-en-1-one 37-derived formation of the substituted furan 2(5H)-one derivative 107.90 | |
In 2022, Ali et al. developed a straightforward, facile, and efficient approach for the synthesis of novel thioether-linked coumarin-fused furans via a Sc(OTf)3-catalyzed one-pot combination of aryl glyoxal 1, 4-hydroxycoumarin 72, and different aromatic thiols 108. This approach could yield either a three-component thioether-linked coumarin-fused furan 109 or a two-component furo-coumarin product 110, depending on the thiols. The key attributes of this approach were its broad substrate range, good to exceptional yields, and products with multiple pharmaceutically significant motifs (Scheme 71).91
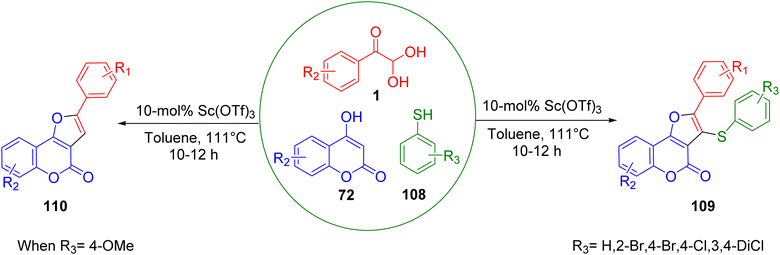 |
| Scheme 71 Syntheses of thioether-linked coumarin-fused furan 109 and furo-coumarin product 110 through aromatic thiols 108.91 | |
For the first time, the synthesis of substituted 2-aminooxazoles containing the 3-hydroxy-4H-pyran-4-one moiety 111 was accomplished by Komogortsev's group in a one-step method via the multicomponent condensation of allomaltol derivatives 105 with α-ketoaldehydes 1 and cyanamide 70, followed by an acid-catalyzed recyclization into substituted furo[3,2-b]pyrans 112. The formation of the 2-aminooxozole core as opposed to a urea-containing condensed furan was the distinctive feature of this approach (Scheme 72).92
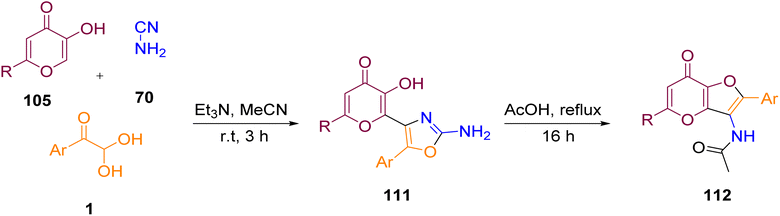 |
| Scheme 72 Synthesis of substituted furo[3,2-b]pyrans 112 derived from allomaltol 111.92 | |
2.5. Miscellaneous reactions
A novel method was developed by Pogaku's team for the production of oxazoles 114 from methyl ketones 24 and TosMIC (tosyl methyl isocyanide) 113 using a self-sorting domino reaction approach. TosMIC was used as an ammonium surrogate in contrast to its typical reactivity as a C–N C synthon in the production of oxazoles 114 (Scheme 73).93 The technique is appealing due to its ease of operation, the wide availability of the starting materials, absence of bases and metals, and the production of C–N and C–O bonds with excellent yields.
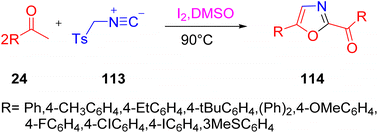 |
| Scheme 73 Synthesis of oxazole 114 under a domino reaction through tosyl methyl isocyanide 113.93 | |
Nagarjuna Babu's group reported the simple and efficient syntheses of oxazoles 118 and furocoumarins 117. The essential step in these transformations comprised the in situ formation of N-acyliminiumion (NAI) precursors 116 from aryl glyoxal and 2-pyrrolidinone 115 in the absence of a catalyst or solvent, followed by their further transformations aided by trifilic acid in the same vessel. It was demonstrated experimentally that the special exocyclic proto-solvated N-acyliminium ion was involved in the reaction. Additionally, the results of the fluorescence and UV–visible experiments revealed that a limited number of the compounds emitted blue light when exposed to light in EtOH in the 404–422 nm wavelength range (Scheme 74).94 An insight into the mechanism initially revealed the formation of the N-acyliminium ion (NAI) precursor ua from 1 and 2-pyrrolodinone 115 under acid catalysis. There were two probable cyclization routes. In route a, the attack of acetonitrile on the iminium intermediate ub leads to the formation of the nitrilium ion uc, which undergoes cyclization to form the final product 118 through the involvement of an adjacent carbonyl group; while in route b, neutralization of the nitrilium ions by water molecules generate the bisamide ud, which subsequently undergoes cyclization, losing a water molecule to yield the desired product 118 (Scheme 75).
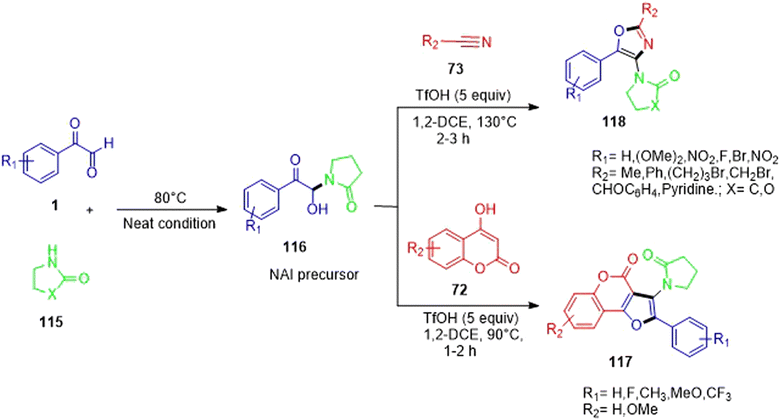 |
| Scheme 74 Syntheses of oxazoles 118 and furocoumarins 117 through the in situ N-acyliminium ion (NAI) precursor.94 | |
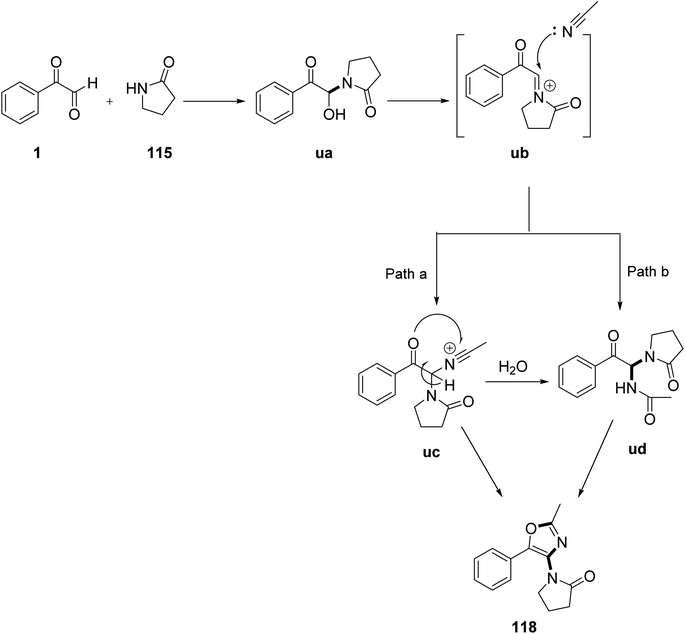 |
| Scheme 75 Reaction pathway for the synthesis of the substituted oxazoles 118. | |
In 2008, Valverde et al. reported the use of aryl glyoxal 1 with aniline 119, cyclohexyl-isocyanide 120, and trichloroacetic acid 121 in dichloromethane, passing through a 3 Å molecular sieve to afford the oxazolone derivative 122 through the Ugi multicomponent reaction pathway group. The remarkable feature of the strategy was the carbonic acid function of the trichloroacetyl group (Scheme 76).95
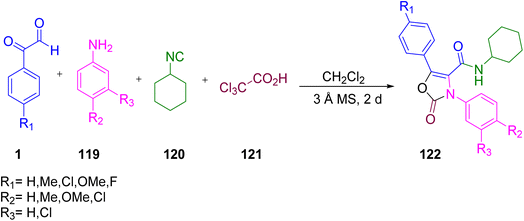 |
| Scheme 76 Synthesis of isoxazole 122 through an Ugi multicomponent reaction.95 | |
2.6. In situ domino reactions of aryl glyoxal to afford oxygen heterocycles
A facile, straightforward, novel, and highly efficient protocol was given by Borah and co-worker in 2016 to obtain functionalized furo[3,2-c]coumarins 123 from 4-hydroxy coumarins 72. The reaction was allowed to proceed by reacting aldehyde/aryl methyl ketone 24 with 72 in the presence of molecular iodine in DMSO at 80 °C. DMSO here works as a solvent as well as an oxidizing agent to recycle the iodine during the reaction process (Scheme 77).96 The plausible mechanism of the reaction is that initially phenylglyoxal 1 is formed from phenyl methyl ketone 24 via sp3-CH activation and oxidation, and is subsequently allowed to react with 4-hydroxycoumarin 72 in the presence of iodine to form the intermediate vb by eliminating a water molecule. Nucleophilic attack by the second molecule of 4-hydroxycoumarin on the intermediate vb yields the final product 123 (Scheme 78).
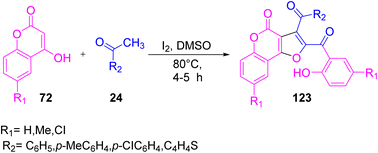 |
| Scheme 77 Furo[3,2-c]coumarin 123 synthesis through in situ-generated phenyl glyoxal.96 | |
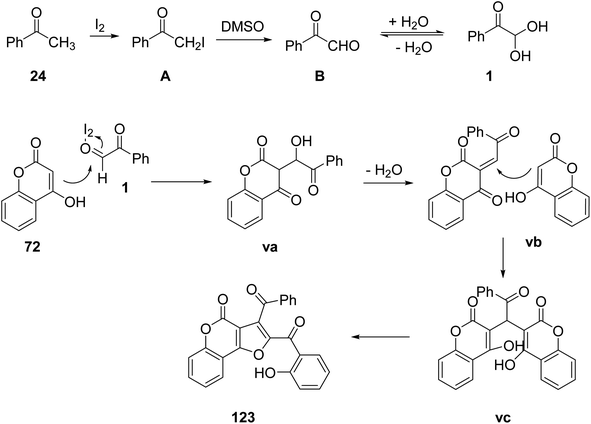 |
| Scheme 78 In situ domino synthesis of furo[3,2-c]coumarin 123 by sp3-CH activation and oxidation. | |
Cheng's group prepared the highly dense benzofuran 23 by bringing aryl glyoxal monohydrate 1, phenol derivatives 22, and indole 21 together in a reaction vessel with molecular iodine in a catalytic amount and DMSO as a solvent. Later, aryl glyoxal 1 was replaced with methylketones 24 and further tested by changing the nucleophiles to thiophenol and 1,2,4-trimethoxybenzene, etc. in place of indole (Scheme 79).7
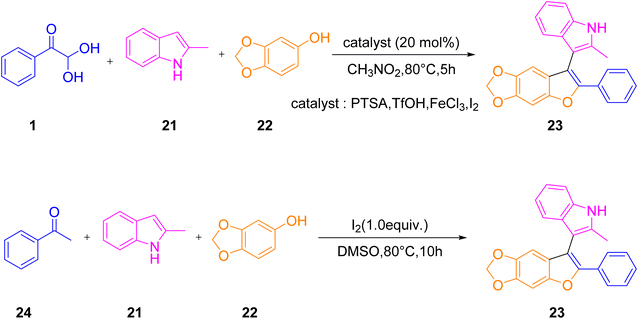 |
| Scheme 79 Synthesis of benzofuran with the indole fragment 23 through methylketones 24.7 | |
In 2012 Jian and colleagues proposed a novel and highly efficient protocol to synthesize polysubstituted oxazole derivatives 125 from simple and easily available starting materials, that is by reacting methyl ketone 24, benzoin 124, and ammonium acetate via the convergent integration of two “self-labour domino sequences”. This reaction has wide application in medicinal and life science chemistry (Scheme 80).97
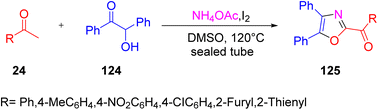 |
| Scheme 80 Domino reaction leading to benzoin 124-derived oxazole 125.97 | |
Zhao and co-workers in 2018 proposed a novel path for the synthesis of fused heterocycles by employing an iodine-promoted fragment assembly method. They formulated pyrazolone-oxepines-pyrazoles 128 by the reaction of phenylhydrazine 126, aryl methyl ketones 24, and acetoacetate ester 127 using molecular iodine at 140 °C in the presence of TfOH, and also explored the application of five-component reactions. Acetoacetate ester engaged in two crucial steps: formation of the 3-methyl-5-pyrazolone skeleton and formation of the C(sp3)–O bond (Scheme 81).98
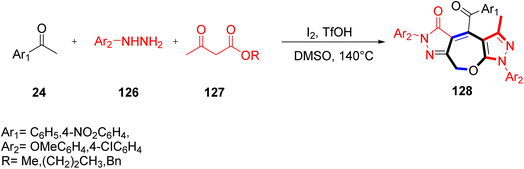 |
| Scheme 81 Synthesis of pyrazolone-oxepines-pyrazoles 128 through phenylhydrazine 126, acetoacetate ester 127, and in situ-generated phenyl glyoxal 1.98 | |
An I2-promoted domino oxidative cyclization method was proposed by Gao and colleagues in 2013 from easily available starting materials, methyl ketones 24, and benzylamines 12 at 100 °C in the absence of any metal or peroxide catalyst to afford 2,5-disubstituted oxazole 129. This reaction occurred with a metal-free catalyst and involved the breaking of C–H bond and the assembly of C–N and C–O bonds (Scheme 82).100 As the reaction involved I2-promoted dual sp3 C–H bond functionalization, its mechanism involved converting acetophenone into the α-iodoacetophenone intermediate A by utilizing I2, which was further oxidized by DMSO to yield the phenylglyoxal intermediate 1. Further, benzylamine 12 reacted with 1 to form the imino-derivative wa or its enolized form wb, which underwent intramolecular cyclization via an oxygen atom at the double bond, resulting in the formation of another intermediate wc. wc deprotonated in the presence of excess iodine to yield the disubstituted oxazole 129 (Scheme 83).
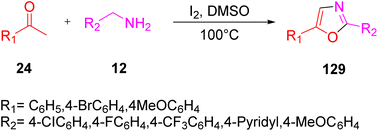 |
| Scheme 82 Oxazole 129 synthesis through methyl ketone 24 and benzylamine 12.98 | |
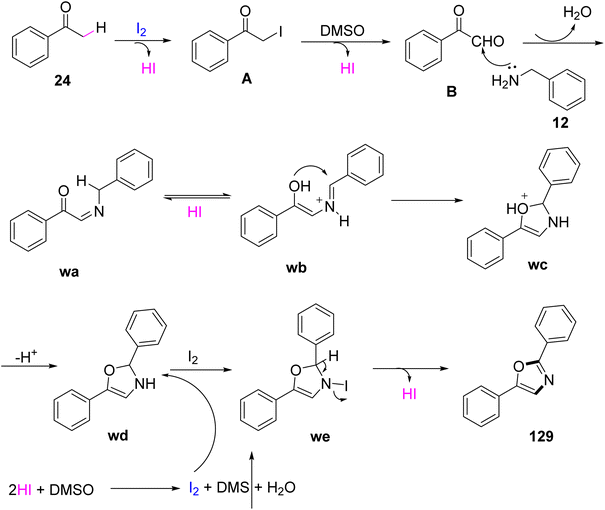 |
| Scheme 83 Formation of oxazole 129 through a domino oxidative cyclization. | |
In 2011, Yang and colleague performed a convergent and linear domino reaction for the first time from easily available inexpensive substrates, like methyl ketone 24, indole 15, and 1,3-dicarbonyl 18, to access the 3-(furan-3-yl or 4-yl) indole derivative 130 via a direct two-step process without the need for purification of the intermediate. This reaction has wide applications in synthetic and medicinal chemistry due to its operational simplicity (Scheme 84).99
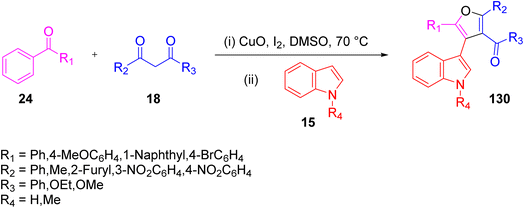 |
| Scheme 84 Synthesis of 3-(furan-3-yl or4-yl) indole derivative 130 through 1,3-diketone 18, indole 15, and methylketone 24.99 | |
3. Conclusion
As a unique molecule with robust applications in organic synthesis, ranging from C–C bond formation reactions to the synthesis of heterocycle libraries, aryl glyoxal is an efficient and easily accessible building block that has been extensively used in the past decade. Exploitation of this commercially available precursor all over the world by chemists has produced many novel functionalized moieties that are both pharmacologically and biologically beneficial to mankind. The different synthetic protocols of aryl glyoxal along with amines and nitrogen heterocycles in multicomponent reaction systems gives rise to highly complex synthetic diversity in the products, attracting researchers to think beyond the current ambit towards new horizons.
After the gigantic number of nitrogen heterocycles, oxygen heterocycles are the second category approved for clinical use as medicinal drugs. Their synthesis through different resources has raised the attention of researchers to a significant level, but there is still a huge gap between the synthetic methodologies and application patterns of nitrogen and oxygen scaffolds. The lower reactivity and somewhat complicated biomimicking process compared to nitrogen members are the possible reasons behind their limited number of applications to date in bio-systems. Further, researchers have brought phenyl glyoxal into action using it either as a substitute for mono-functional aldehydes for forming a structural backbone by adding carbon to the target structure or as an oxygen donor in the product molecule. The optimum use of phenyl glyoxal with new combinations of reagents possessing multiple functionalities is still awaited. The mounting up of oxygen heterocycles' applications is conditional on the proper execution of advanced tools and techniques for their strategic biosynthesis followed by extensive tests and trials.
Conflicts of interest
There is no conflict to declare.
References
- F. Wöhler, Ann. Phys. Chem., 1828, 88, 253–256 CrossRef.
- A. Dömling, W. Wang and K. Wang, Chem. Rev., 2012, 112, 3083–3135 CrossRef PubMed.
- S. Banerjee, A. Horn, H. Khatri and G. Sereda, Tetrahedron Lett., 2011, 52, 1878–1881 CrossRef CAS.
- I. Ugi, A. Dömling and W. Hörl, Endeavour, 1994, 18, 115–122 CrossRef CAS.
- M. M. Khan, R. Yousuf, S. Khan and S. Shafiullah, RSC Adv., 2015, 5, 57883–57905 RSC.
- R. C. Cioc, E. Ruijter and R. V. A. Orru, Green Chem., 2014, 16, 2958–2975 RSC.
- C. Cheng, C. Liu and Y. Gu, Tetrahedron, 2015, 71, 8009–8017 CrossRef CAS.
- M. M. Khan, S. Khan, S. Saigal and S. Iqbal, RSC Adv., 2016, 6, 42045–42061 RSC.
- A. M. El-Agrody, A. M. Fouda and A.-A. M. Al-Dies, Med. Chem. Res., 2014, 23, 3187–3199 CrossRef CAS.
- A. Zonouzi, R. Mirzazadeh, M. Safavi, S. Kabudanian Ardestani, S. Emami and A. Foroumadi, Iran. J. Pharm. Res., 2013, 12, 679–685 CAS.
- A. Poursattar Marjani, J. Khalafy and A. Farajollahi, J. Heterocycl. Chem., 2019, 56, 268–274 CrossRef CAS.
- P. Prasad, P. G. Shobhashana and M. P. Patel, R. Soc. Open Sci., 2017, 4, 170764 CrossRef PubMed.
- D. Armesto, W. M. Horspool, N. Martin, A. Ramos and C. Seoane, J. Org. Chem., 1989, 54, 3069–3072 CrossRef CAS.
- M. Eghtedari, Y. Sarrafi, H. Nadri, M. Mahdavi, A. Moradi, F. Homayouni Moghadam, S. Emami, L. Firoozpour, A. Asadipour, O. Sabzevari and A. Foroumadi, Eur. J. Med. Chem., 2017, 128, 237–246 CrossRef CAS PubMed.
- X. L. Hou, H. Y. Cheung, T. Y. Hon, P. Lo Kwan, T. H. Lo, S. Y. Tong and H. N. C. Wong, Tetrahedron, 1998, 54, 1955–2020 CrossRef CAS.
- W. Fenical, R. K. Okuda, M. M. Bandurraga, P. Culver and R. S. Jacobs, Science, 1981, 212, 1512–1514 CrossRef CAS PubMed.
- I. Butenschön, K. Möller and W. Hänsel, J. Med. Chem., 2001, 44, 1249–1256 CrossRef PubMed.
- H. N. Akolkar, S. G. Dengale, K. K. Deshmukh, B. K. Karale, N. R. Darekar, V. M. Khedkar and H. Mubarak, Polycyclic Aromat. Compd., 2020, 1–13 Search PubMed.
- A. Lilienkampf, Annamaria, M. Pieroni, S. G. Franzblau, R. B. William and P. Kozikowski, Curr. Top. Med. Chem., 2012, 12(6), 729–734 CrossRef CAS PubMed.
- J. J. Talley, D. L. Brown, J. S. Carter, M. J. Graneto, C. M. Koboldt, J. L. Masferrer, W. E. Perkins, R. S. Rogers, A. F. Shaffer, Y. Y. Zhang, B. S. Zweifel and K. Seibert, J. Med. Chem., 2000, 43, 775–777 CrossRef CAS PubMed.
- G. Daidone, D. Raffa, B. Maggio, F. Plescia, V. M. C. Cutuli, N. G. Mangano and A. Caruso, Arch. Pharm., 1999, 332, 50–54 CrossRef CAS PubMed.
- V. Raj and J. Lee, Front. Chem., 2020, 8, 623 CrossRef CAS PubMed.
- A. N. Panche, A. D. Diwan and S. R. Chandra, J. Nutr. Sci., 2016, 5, e47 CrossRef CAS PubMed.
- G. Brahmachari and B. Banerjee, ACS Sustainable Chem. Eng., 2014, 2, 411–422 CrossRef CAS.
- H. Sourgens, R. Hoerr, A. Biber, H. Steinbrede and H. Derendorf, J. Clin. Pharmacol., 1998, 38, 373–381 CrossRef CAS PubMed.
- P. Ravichandiran, B. Lai and Y. Gu, Chem. Rec., 2017, 17, 142–183 CrossRef CAS PubMed.
- N. G. Khaligh, Monatsh. Chem., 2018, 149, 33–38 CrossRef CAS.
- D.-L. Wang, S.-Q. Zhang, S.-T. Guo, J. Xu, X.-L. Zhang, X.-S. Xiong and L. Zhang, Heterocycles, 2021, 102, 105 CrossRef.
- M. Guarrera, L. Turbino and A. Rebora, J. Eur. Acad. Dermatol. Venereol., 2001, 15, 486–487 CrossRef CAS PubMed.
- A. S. Alqahtani, S. Hidayathulla, M. T. Rehman, A. A. ElGamal, S. Al-Massarani, V. Razmovski-Naumovski, M. S. Alqahtani, R. A. El Dib and M. F. AlAjmi, Biomolecules, 2019, 10, 61 CrossRef PubMed.
- M. Karami, A. Hasaninejad, H. Mahdavi, A. Iraji, S. Mojtabavi, M. A. Faramarzi and M. Mahdavi, Mol. Diversity, 2022, 26, 2393–2405 CrossRef CAS PubMed.
- M. Hanefeld and F. Schaper, Expert Rev. Cardiovasc. Ther., 2008, 6, 153–163 CrossRef PubMed.
- M. M. Heravi, B. A. Jani, F. Derikvand, F. F. Bamoharram and H. A. Oskooie, Catal. Commun., 2008, 10, 272–275 CrossRef CAS.
- R. Gašparová, P. Koiš, M. Lácová, S. Kováčová and A. Boháč, Open Chem., 2013, 11, 502–513 Search PubMed.
- M. Rimaz, H. Mousavi, B. Khalili and L. Sarvari, J. Iran. Chem. Soc., 2019, 16, 1687–1701 CrossRef CAS.
- L. Palanivel and V. Gnanasambandam, Org. Biomol. Chem., 2020, 18, 3082–3092 RSC.
- R. Hazen, R. Harvey, R. Ferris, C. Craig, P. Yates, P. Griffin, J. Miller, I. Kaldor, J. Ray, V. Samano, E. Furfine, A. Spaltenstein, M. Hale, R. Tung, M. St. Clair, M. Hanlon and L. Boone, Antimicrob. Agents Chemother., 2007, 51, 3147–3154 CrossRef CAS PubMed.
- A. K. Ghosh, Z. L. Dawson and H. Mitsuya, Bioorg. Med. Chem., 2007, 15, 7576–7580 CrossRef CAS PubMed.
- K. McKeage, C. M. Perry and S. J. Keam, Drugs, 2009, 69, 477–503 CrossRef CAS PubMed.
- S. Gupta, B. Kushwaha, A. Srivastava, J. P. Maikhuri, S. N. Sankhwar, G. Gupta and A. K. Dwivedi, RSC Adv., 2016, 6, 76288–76297 RSC.
- A. Glasier, in Endocrinology: Adult and Pediatric, Elsevier, 2016, pp. 2297–2309.e2 Search PubMed.
- S. A. Dingsdag and N. Hunter, J. Antimicrob. Chemother., 2018, 73, 265–279 CrossRef CAS PubMed.
- B. Eftekhari-Sis, M. Zirak and A. Akbari, Chem. Rev., 2013, 113, 2958–3043 CrossRef CAS PubMed.
- J. Li, L. Liu, D. Ding, J. Sun, Y. Ji and J. Dong, Org. Lett., 2013, 15, 2884–2887 CrossRef CAS PubMed.
- N. R. Modugu and P. K. Pittala, Tetrahedron Lett., 2017, 58, 3859–3863 CrossRef CAS.
- A. N. Komogortsev, B. V. Lichitsky and V. G. Melekhina, Molbank, 2021, 2021, 2–6 CrossRef.
- H. Shahbazi-Alavi, R. Teymuri and J. Safaei-Ghomi, Nanochem Res., 2021, 6(2), 135–142 CAS.
- S. M. Ebrahimi, B. Hamah-Ameen, A. Kareem Abbas, H. Shahbazi-Alavi, H. Gholamzadeh and J. Safaei-Ghomi, Polycyclic Aromat. Compd., 2021, 1–9 CAS.
- C. Liu, L. Zhou, D. Jiang and Y. Gu, Asian J. Org. Chem., 2016, 5, 367–372 CrossRef CAS.
- F. Dehghanzadeh, F. Shahrokhabadi and M. Anary-Abbasinejad, Arkivoc, 2019, 2019, 133–141 Search PubMed.
- A. El-Harairy, Yiliqi, M. Yue, W. Fan, F. Popowycz, Y. Queneau, M. Li and Y. Gu, ChemCatChem, 2019, 11, 4403–4410 Search PubMed.
- R. Khoeiniha, A. Olyaei and M. Saraei, Synth. Commun., 2018, 48, 155–160 CrossRef CAS.
- B. V. Lichitskii, V. G. Melekhina, A. N. Komogortsev, C. V. Milyutin, A. N. Fakhrutdinov, Y. O. Gorbunov and M. M. Krayushkin, Org. Biomol. Chem., 2020, 18, 2501–2509 RSC.
- R. P, A. I. Almansour, N. Arumugam and S. Yaragorla, Org. Biomol. Chem., 2021, 19, 1060–1065 RSC.
- B. V. Lichitsky, A. N. Komogortsev and V. G. Melekhina, Molbank, 2022, 2022, M1315 CrossRef.
- A. N. Komogortsev, B. V. Lichitsky and V. G. Melekhina, Tetrahedron Lett., 2021, 78, 153292 CrossRef CAS.
- C. X. Chen, L. Liu, D. P. Yang, D. Wang and Y. J. Chen, Synlett, 2005, 2047–2051 CAS.
- M. H. Mosslemin, M. Anary-Abbasinejad, A. F. Nia, S. Bakhtiari and H. Anaraki-Ardakani, J. Chem. Res., 2009, 599–601 CrossRef CAS.
- B. Karami, S. Khodabakhshi and F. Hashemi, Tetrahedron Lett., 2013, 54, 3583–3585 CrossRef CAS.
- M. R. Salari, M. H. Mosslemin and A. Hassanabadi, J. Chem. Res., 2017, 41, 657–660 CrossRef CAS.
- M. Reza Salari, M. H. Mosslemin and A. Hassanabadi, J. Chem. Res., 2019, 4–7 Search PubMed.
- X. Zhang, P. Zeng, S. Zhang and Z. Chen, ChemistrySelect, 2020, 5, 3934–3938 CrossRef CAS.
- M. Taheri, R. Mohebat and M. H. Moslemin, Artif. Cells, Nanomed., Biotechnol., 2021, 49, 250–260 CrossRef CAS PubMed.
- M. Taheri, R. Mohebat and M. H. Moslemin, Polycyclic Aromat. Compd., 2021, 1–11 Search PubMed.
- A. N. Komogortsev, V. G. Melekhina, B. V. Lichitsky, V. A. Migulin, T. T. Karibov and M. E. Minyaev, Tetrahedron, 2022, 111, 132716 CrossRef CAS.
- S. Khodabakhshi, B. Karami and M. Baghernejad, Monatsh. fur Chem., 2014, 145, 1839–1843 CrossRef CAS.
- S. Khodabakhshi and B. Karami, New J. Chem., 2014, 38, 3586–3590 RSC.
- S. Khodabakhshi, B. Karami, K. Eskandari and M. Farahi, Tetrahedron Lett., 2014, 55, 3753–3755 CrossRef CAS.
- M. Rimaz, A. Mirshokraie, B. Khalili and P. Motiee, Arkivoc, 2015, 2015, 88–98 Search PubMed.
- N. Etivand, J. Khalafy and M. G. Dekamin, Synth, 2020, 52, 1707–1718 Search PubMed.
- M. Poursattar Marjani, Ahmad, J. Khalafy, P. Eslamipour and A. Sabegh, Iran. J. Chem. Chem. Eng., 2019, 38 Search PubMed.
- R. Mishra and L. H. Choudhury, RSC Adv., 2016, 6, 24464–24469 RSC.
- J. Khalafy, F. M. Arlan and S. S. Chalanchi, J. Heterocycl. Chem., 2018, 55, 149–153 CrossRef CAS.
- J. Khalafy, S. Ilkhanizadeh and M. Ranjbar, J. Heterocycl. Chem., 2018, 55, 951–956 CrossRef CAS.
- S. Nasri and M. Bayat, J. Mol. Struct., 2018, 1164, 77–83 CrossRef CAS.
- S. Khodabakhshi, F. Jafari, F. Marahel and M. Baghernejad, Heterocycl. Commun., 2014, 20, 285–288 CrossRef CAS.
- S. Khodabakhshi, M. Shahamirian and M. Baghernejad, J. Chem. Res., 2014, 38, 473–476 CrossRef CAS.
- A. Poursattar Marjani, J. Khalafy and A. Farajollahi, J. Heterocycl. Chem., 2019, 56, 268–274 CrossRef CAS.
- A. Poursattar Marjani, B. Ebrahimi Saatluo and F. Nouri, Iran. J. Chem. Chem. Eng., 2018, 37, 149–157 CAS.
- M. Taheri and R. Mohebat, Green Chem. Lett. Rev., 2020, 13, 1–14 CrossRef.
- A. N. Komogortsev, V. G. Melekhina, B. V. Lichitsky and M. E. Minyaev, Tetrahedron Lett., 2020, 61, 152384 CrossRef CAS.
- A. Berjis, B. Mirza and H. Anaraki-ardakani, J. Serb. Chem. Soc., 2021, 86, 547–553 CrossRef CAS.
- B. Karami, S. Khodabakhshi and K. Eskandari, Synlett, 2013, 24, 998–1000 CrossRef CAS.
- Z. Chen, P. Zeng, S. Zhang and X. Huang, ChemistrySelect, 2021, 6, 4539–4543 CrossRef CAS.
- B. V Lichitsky, A. N. Komogortsev and V. G. Melekhina, 2021, 2–6.
- B. V. Lichitsky, V. G. Melekhina, A. N. Komogortsev, V. A. Migulin, Y. V. Nelyubina, A. N. Fakhrutdinov, E. D. Daeva and A. A. Dudinov, Tetrahedron, 2021, 83, 131980 CrossRef CAS.
- X. Chang, X. Zhang and Z. Chen, Org. Biomol. Chem., 2018, 16, 4279–4287 RSC.
- X. Chang, P. Zeng and Z. Chen, Eur. J. Org. Chem., 2019, 2019, 6478–6485 CrossRef CAS.
- A. N. Komogortsev, B. V. Lichitsky, A. D. Tretyakov, A. A. Dudinov and M. M. Krayushkin, Chem. Heterocycl. Compd., 2019, 55, 818–822 CrossRef CAS.
- B. V. Lichitsky, V. G. Melekhina, A. N. Komogortsev and M. E. Minyaev, Tetrahedron Lett., 2020, 61, 152602 CrossRef CAS.
- H. B. Jalani and J. Jeong, J. Heterocycl. Chem., 2022, 59, 1266–1271 CrossRef CAS.
- A. N. Komogortsev, B. V. Lichitsky, T. T. Karibov and V. G. Melekhina, Tetrahedron, 2022, 117–118, 132836 CrossRef CAS.
- N. Pogaku, P. R. Krishna and Y. L. Prapurna, Synth. Commun., 2018, 48, 1986–1993 CrossRef CAS.
- V. N. Babu, A. Murugan, N. Katta, S. Devatha and D. S. Sharada, J. Org. Chem., 2019, 84, 6631–6641 CrossRef CAS PubMed.
- M. García-Valverde, S. Macho, S. Marcaccini, T. Rodríguez, J. Rojo and T. Torroba, Synlett, 2008, 2, 0033–0036 Search PubMed.
- S. Kolita, P. Borah, P. S. Naidu and P. J. Bhuyan, Tetrahedron, 2016, 72, 532–538 CrossRef CAS.
- W. J. Xue, Q. Li, Y. P. Zhu, J. G. Wang and A. X. Wu, Chem. Commun., 2012, 48, 3485–3487 RSC.
- P. Zhao, X. Wu, X. Geng, C. Wang, Y. D. Wu and A. X. Wu, Tetrahedron, 2018, 74, 4323–4330 CrossRef CAS.
- Y. Yang, M. Gao, L. M. Wu, C. Deng, D. X. Zhang, Y. Gao, Y. P. Zhu and A. X. Wu, Tetrahedron, 2011, 67, 5142–5149 CrossRef CAS.
- Q.-H. Gao, Tetrahedron, 2013, 69(1), 22–28 CrossRef CAS.
|
This journal is © The Royal Society of Chemistry 2023 |
Click here to see how this site uses Cookies. View our privacy policy here.