DOI:
10.1039/D2RA07826K
(Paper)
RSC Adv., 2023,
13, 2451-2457
Computed ammonia affinity for evaluating Lewis acidity of organoboronates and organoboronamides†
Received
8th December 2022
, Accepted 6th January 2023
First published on 17th January 2023
Abstract
Lewis acidity of organoboronates [B(pin), B(neop), B(cat), B(eg), B(nad)] and organoboronamides [B(dan), B(aam), B(mdan)] has been found to be unifiedly evaluated by computed ammonia affinity (AA), while other methods [LUMO energies, global electrophilicity index (GEI), fluoride ion affinity (FIA)] were only partially applicable. The relationships between the AA values and such structural characters including the B–X bond lengths, the X–B–X angles, and the changes in the B–X bond lengths in the formation of the ammonia adducts were also described.
Introduction
Organoboronic acids [R–B(OH)2] and diol-derived cyclic boronates, e.g. pinacol [R–B(pin)] and neopentyl glycol boronates [R–B(neop)] have proven to be invaluable organometallic reagents in modern synthetic organic chemistry that are utilized for diverse carbon–carbon and carbon–heteroatom bond-forming reactions (Fig. 1A).1 Lewis acidity of their boron centers usually governs the reactivities; most of the organoboron-based transformations, regardless of catalytic/non-catalytic ones, proceed through donor–acceptor interaction between Lewis basic moieties and the Lewis acidic 2p empty orbital of the boron centers. The characteristics, on the other hand, have led to synthetic chemistry with such Lewis acidity-diminished, “protected” boronamides as B(dan)2–4 and B(aam)4–6 (Fig. 1B): the organoboronamides are generally reluctant to participate in transmetalation and thus can be used for the Suginome's boron-masking strategy, where Lewis acidic organoboronic acids/boronates chemoselectively undergo Suzuki–Miyaura coupling (SMC, Fig. 2A).2,6–8 The difference in boron-Lewis acidity of unsymmetrical diborons [(amide)B–B(pin)] also enables chemoselective σ-bond metathesis with transition metal complexes, resulting in various catalytic boronamide-installation reactions (Fig. 2B),3,4,9–13 and furthermore the Lewis acidity-diminishment with the boronamide moieties was demonstrated to improve significantly air- and/or water-resistant properties of the respective H–B,14–16 2-pyridyl–B10,11,17,18 and PhMe2Si–B19 compounds, being known to be unstabilized in their Lewis acidic B(pin)-forms (Fig. 2C). The stabilizing effect was also observed in hydrolysis of phenylboranes, and only Ph–B(dan) remained completely intact (Fig. 2D).20 It should also be noted that ease of the hydrolysis of phenylboronates is dependent on the diol structures that could affect their Lewis acidity.
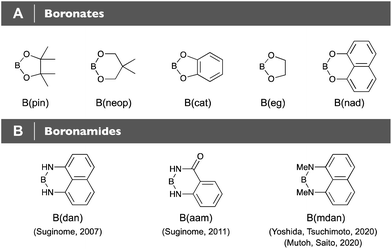 |
| Fig. 1 (A) Boronates. (B) Boronamides. | |
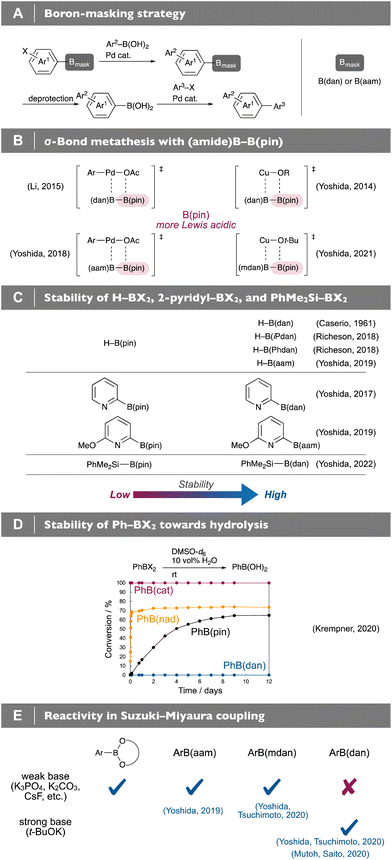 |
| Fig. 2 (A) Boron-masking strategy. (B) σ-Bond metathesis with (amide)B–B(pin). (C) Stability of H–BX2, 2-pyridyl–BX2, and PhMe2Si–BX2. (D) Stability of Ph–BX2 towards hydrolysis. (E) Reactivity in Suzuki–Miyaura coupling. | |
Despite the diminished Lewis acidity of the boronamide moieties, we found that Ar–B(aam)18 and Ar–B(mdan)21 smoothly underwent direct SMC under weak base conditions (Fig. 2E), and moreover the use of a strong base, t-BuOK, could activate even Ar–B(dan) toward direct SMC.21,22 All of the above experimental results on the reactivity and the stability instinctively indicate that the boron-Lewis acidity decreases in “B(diol) > B(aam) ≈ B(mdan) > B(dan)” order; however, theoretical approaches to quantitative evaluation of the Lewis acidity of these synthetically important organoboronic acid derivatives have been underdeveloped. We have recently reported on copper-catalyzed internal-selective boronamide-installation reactions to terminal alkynes using (amide)B–B(pin), in which the regioselectivity closely correlates with the degree of the Lewis acidity-diminishment that can be determined by theoretical calculation-based fluoride ion affinity (FIA).12 Although the calculated Lewis acidity order of the boronamide moieties [B(aam) > B(mdan) > B(dan)] is in good agreement with the above experimental results, the computed FIA turned out to be inapplicable to comparison of the boron-Lewis acidity without structural similarity; the FIA value of an experimentally more Lewis acidic B(pin) is much lower than those of the boronamide moieties. Herein, we disclose that calculation-based ammonia affinity (AA) provides a unified method for evaluating Lewis acidity of organoboronates and organoboronamides, which leads to a useful way of estimating such experimental behaviors as reactivity, selectivity, and stability of diverse organoboron compounds (Fig. 3).23,24
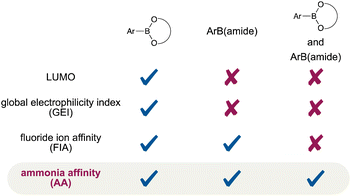 |
| Fig. 3 Various methods for evaluating Lewis acidity of organoboronic acid derivatives. | |
Computational methods
Geometry optimizations and frequency calculations were conducted by using M06-2X25 with def2-SVP25–27 basis set on Gaussian 16 Rev. A.03 program.28 We chose the M06-2X functional, which has been well established to show accurate results in the models including Lewis donor–acceptor interactions.29–31 The def2-SVP basis set was chosen because calculations can be conducted with less computational resources as compared with other basis sets such as def2-TZVP. Additionally, the def2-SVP basis set is also applicable to other main group Lewis acids such as organostannanes.32 All optimized structures were confirmed to be local minima by verifying the absence of imaginary frequencies. Energies of the orbitals, EHOMO and ELUMO, and enthalpies, H, were obtained from geometry optimizations at this level of theory.
Intrinsic Lewis acidity (iLA), which denotes Lewis acidity of free Lewis acids without influence of deformation energy arising from formation of Lewis acid–base adducts,33 is usually evaluated by energies of LUMO or global electrophilicity index (GEI).33–36 GEI values, ω, were calculated from the chemical potential, μ, and chemical hardness, η, as shown in eqn (1); these values were obtained from energies of the HOMO and LUMO orbitals (eqn (2) and (3)).37,38
|
μ = 1/2(EHOMO + ELUMO)
| (2) |
Although LUMO energies and GEI values have frequently been used for estimating Lewis acidity of main group Lewis acids and are rapidly accessible with less computational resources, their use for comparing Lewis acids without structural similarity sometimes results in inaccurate estimation.36 Therefore, our attention was focused on global Lewis acidity (gLA) of organoboronic acid derivatives; gLA is evaluated by various affinity scales including fluoride ion affinity (FIA) and ammonia affinity (AA).33,39 Owing to difficulties in calculating accurate energies of a “naked” fluoride ion, FIA values were obtained by using the experimental FIA value of COF2 (209 kJ mol−1) as an anchor point in a (pseudo-)isodesmic reaction at the same level of theory according to eqn (4)–(6).31,40
|
LA + COF3− → [LA–F]− + COF2: ΔH1
| (4) |
|
COF3− → COF2 + F−: ΔH2 = 209 kJ mol−1
| (5) |
|
LA + F− → [LA–F]−: FIA = ΔH1 − ΔH2
| (6) |
On the other hand, AA values were obtained by non-isodesmic methods according to eqn (7).39
|
AA = HLewis adduct − (HLewis acid + HNH3)
| (7) |
Although various affinity values usually become negative as exothermic reactions, these values are often expressed in positive numbers by convention.33 FIA values calculated at the M06-2X/def2-SVP level of theory were used in this study for their comparison with AA because diffuse functions were reported to be neglectable for larger anions in FIA calculations.31 Indeed, FIA values calculated at various levels of theory were found to show nearly unchanged Lewis acidity order of phenylboronic acid derivatives used in this study (Fig. S2†).
Results and discussion
Evaluation of Lewis acidity of organoboronic acid derivatives by theoretical calculation
We first calculated gLA (FIA) as well as iLA (LUMO energies and GEI) of phenyl-boronamides [B(dan), B(aam), and B(mdan)] and -boronates [B(pin), B(neop), B(cat), B(eg), and B(nad)20] (Table 1 or Fig. 4). All the calculated Lewis acidity of phenylboronates showed good correlation with the Lewis acidity obtained by Gutmann–Beckett (GB) method20,41,42 and the above experimental results (Fig. 2); the calculated values of B(cat) and B(nad) of strong Lewis acidity among the boronates fluctuated depending upon the methods. Although the FIA values were found to be reasonable also in the case of phenylboronamides, their ELUMO and GEI values estimated that B(mdan) is the least Lewis acidic in the order of B(aam) > B(dan) > B(mdan), being inconsistent with the experimental results. On the other hand, some fluctuations were observed in the FIA-based Lewis acidity order of B(aam) and B(mdan) at various levels of theory (Fig. S2†). The above results revealed that the FIA would be more useful for evaluating the Lewis acidity of a broad range of phenylboronic acid derivatives with structural similarity; however, it faced serious limitations in comparing boronamides with boronates: the FIA values of the aliphatic diol-derived boronates [B(pin), B(neop), and B(eg)] became lower than those of the boronamides. Because the FIA calculation uses closely contact Lewis acid–fluoride ion adducts, this method may be inappropriate for the evaluation of the Lewis acidity of the “protected” boronamides that are inherently difficult to form the contact adducts,21 resulting in the overestimation of the Lewis acidity. Hence, our attention was focused on computed gLA by employing loose Lewis acid–base adducts, ammonia affinity (AA).39 As depicted in Fig. 4, the calculated AA turned out to unifiedly evaluate the Lewis acidity of the boronamides and boronates; the Lewis acidity order [B(aromatic diol) > B(aliphatic diol) > B(mdan) ≈ B(aam) > B(dan)] is in good agreement with the experimental results.43 Considering the presence of πPh to pB electron donation in a Ph–B bond,24b it might be conceivable that the higher Lewis acidity of PhB(mdan) than that of PhB(dan) arises from the Ph moiety almost perpendicular to the plane of the B(mdan) (dihedral angle of CorthoCipso–BN = 75.3°). However, AA values of methylboronamides showed a similar trend [MeB(dan): 10.1 < MeB(aam): 16.7 < MeB(mdan): 18.3 < MeB(pin): 28.8, see ESI† for details], and thus we concluded that the Lewis acidity is governed by the boronamide moieties.
Table 1 Calculated ELUMO (eV), GEI (eV), FIA (kJ mol−1), and AA (kJ mol−1) of phenylboronic acid derivatives
PhBX2 |
ELUMO |
GEI |
FIA |
AA |
B(dan) |
−0.003 |
0.766 |
277.4 |
12.1 |
B(aam) |
−0.283 |
1.056 |
294.0 |
18.6 |
B(mdan) |
0.264 |
0.665 |
301.8 |
21.5 |
B(neop) |
0.330 |
0.889 |
252.8 |
23.7 |
B(pin) |
0.219 |
0.943 |
265.6 |
36.2 |
B(eg) |
0.174 |
0.965 |
258.4 |
36.3 |
B(cat) |
−0.275 |
1.073 |
322.8 |
54.7 |
B(nad) |
−0.280 |
0.981 |
334.7 |
64.8 |
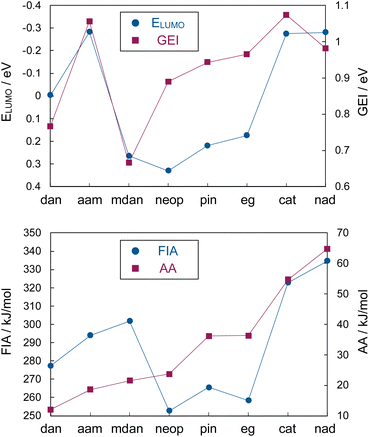 |
| Fig. 4 Evaluation of Lewis acidity of phenylboronic acid derivatives by theoretical calculation. | |
Correlation between AA and B–X bond lengths of phenylboronic acid derivatives
The validity of the AA for evaluating the Lewis acidity of the phenylboronic acid derivatives was also confirmed by collating them with the B–X bond lengths:44 the stronger the AA-based Lewis acidity is, the longer the B–X bond lengths become (Table 2 or Fig. 5). In general, shorter B–X bond lengths reflect weaker boron-Lewis acidity, because these clearly show effective electron-donation from a lone pair on X to a vacant p orbital of B.45–47 Although there might be non-covalent interactions between ammonia and the coronene moiety in the ammonia–PhB(cordan) adduct,48 the good correlation (AA values vs. B–X bond lengths) clearly suggests that non-covalent interactions would not affect the AA values.
Table 2 Calculated AA (kJ mol−1), B–X bond (Å), X–B–X angle (°), and ΔB–XNH3 bond (Å) of phenylboronic acid derivatives
PhBX2 |
AA |
B–X bond |
X–B–X angle |
ΔB–XNH3 bond |
B(dan) |
12.1 |
1.423 |
116.1 |
0.06 |
B(aam) |
18.6 |
1.428 |
116.0 |
0.063 |
B(mdan) |
21.5 |
1.431 |
118.8 |
0.067 |
B(iPdan) |
21.6 |
1.434 |
120.4 |
0.072 |
B(Cydan) |
22.1 |
1.433 |
120.4 |
0.075 |
B(Phdan) |
29.1 |
1.435 |
117.8 |
0.079 |
B(cordan) |
29.7 |
1.436 |
117.6 |
0.079 |
B(eedan) |
49.2 |
1.440 |
117.7 |
0.089 |
B(eydan) |
71.4 |
1.445 |
116.6 |
0.091 |
B(neop) |
23.7 |
1.366 |
121.9 |
0.072 |
B(pin) |
36.2 |
1.369 |
112.6 |
0.069 |
B(eg) |
36.3 |
1.368 |
112.8 |
0.067 |
B(cat) |
54.7 |
1.386 |
110.7 |
0.068 |
B(nad) |
64.8 |
1.374 |
120.6 |
0.067 |
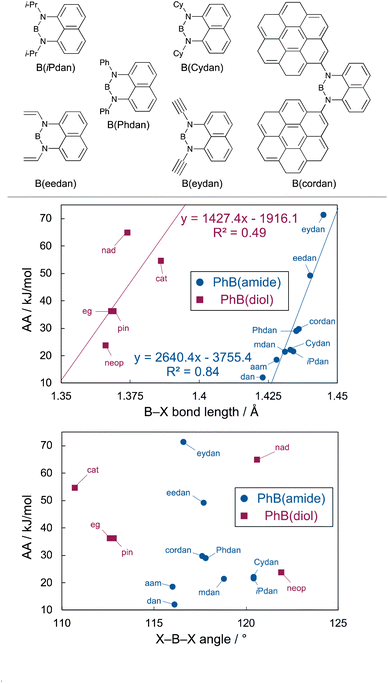 |
| Fig. 5 Correlation between AA and B–X bond length or X–B–X angle of phenylboronic acid derivatives. | |
Correlation between AA and X–B–X angles of phenylboronic acid derivatives
We next investigated correlation between the AA values and X–B–X angles; Sporzyński reported that the geometric parameter (O–B–O angle) was a major factor for the Lewis acidity of diol-derived organoboronates that was experimentally evaluated by the GB method, and that those having O–B–O angle close to 120° exhibited the weaker Lewis acidity because of energetically unfavorable planar (sp2)-to-tetrahedral (sp3) interconversion.41 Besides, they concluded that the differences in the Lewis acidity were not caused by any electronic effect originating from the diol moieties. A similar correlation was also observed between the AA values and the O–B–O angles of the phenylboronates, except for the B(nad) with 120.6° O–B–O angle that showed the highest AA value. In stark contrast, there was almost no correlation between the AA values and the N–B–N angles of the phenylboronamides, suggesting that X–B–X bond angles are not always a major factor affecting boron-Lewis acidity.
Optimized structures of ammonia/fluoride adducts of phenylboronic acid derivatives
The optimized structures of the ammonia–phenylboronamide adducts were found to undergo less planar-to-tetrahedral interconversion than those of the fluoride adducts (Fig. 6B vs. Fig. 6C), whereas the planar-to-tetrahedral interconversion in forming the ammonia/fluoride adducts was almost similar in the cases of the Lewis acidic phenylboronates (PhB(pin)F−, 140.3°; PhB(pin)NH3, 138.9°, see ESI† for details).49 The interconversion generally causes increase in the B–X lengths;50 we next investigated relationship between the FIA or AA values and the changes in the B–X bond lengths (ΔB–XF− or ΔB–XNH3) in the formation of the adducts. There was almost no correlation between the FIA values and ΔB–XF− [ΔB–XF−: phenylboronates, 0.111 Å–0.119 Å; B(dan) (0.106 Å) ≈ B(aam) (0.106 Å) > B(mdan) (0.102 Å), see ESI† for details]. On the other hand, good correlation could be found between the AA values and ΔB–XNH3 of the phenylboronamides, although this was not applied to the phenylboronates (Table 2 or Fig. 7). These results may be ascribable to the relatively planar optimized structures of the ammonia–phenylboronamide adducts, in which the electron-donation influence from the N atoms to the B center was properly reflected.51
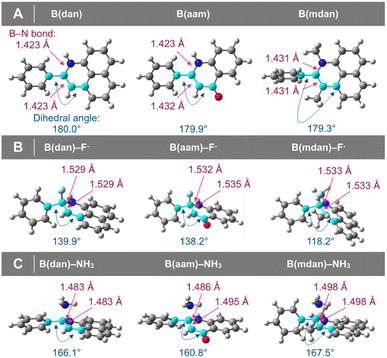 |
| Fig. 6 (A) Optimized structures of phenylboronamides. (B) Optimized structures of fluoride–phenylboronamide adducts. (C) Optimized structures of ammonia–phenylboronamide adducts. | |
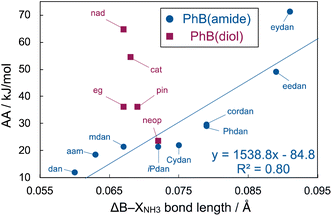 |
| Fig. 7 Correlation between AA and ΔB–XNH3 of phenylboronic acid derivatives. | |
Experimental demonstration of the predicted Lewis acidity of the phenylboronamides
According to the AA analysis, B(aam) is the second least Lewis acidic, and this has been well supported by its chemoselectivity (Fig. 2B) and stabilizing effect (Fig. 2C); however, PhB(aam) was reported to be readily hydrolyzed as compared with more Lewis acidic PhB(pin) (Fig. 8A).5 Assuming that the hydrolysis of the B(aam) moiety exceptionally proceeds not through direct attack of water to the boron center that is markedly affected by boron-Lewis acidity but through protonation of the amide carbonyl group, we calculated the AA of PhB(aam)H+ to observe considerable increase in its value (Fig. 8B, 79.3 kJ mol−1) that could result in the fast hydrolysis. Since amide carbonyl groups have been well established to be easily protonated52 and hydrogen bonding of the B(aam) moiety was reported,53 we concluded that the behavior of the B(aam) moiety in the presence of water is totally different from that under non-aqueous conditions. On the other hand, the Lewis acidity-diminished B(mdan) moiety having no carbonyl group, whose AA value is like that of the B(aam), was demonstrated to show excellent stability toward hydrolysis (Fig. 8C). In addition to the fact that PhB(mdan) can undergo direct SMC under weak base conditions (Fig. 8D),21 its high water-resistant property that can be estimated by the AA analysis would be of high synthetic practicality.
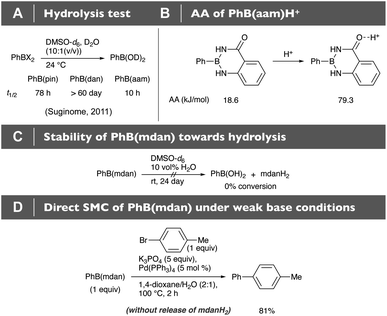 |
| Fig. 8 (A) and (C) Hydrolysis tests. (B) AA of PhB(aam)H+. (D) Direct SMC of PhB(mdan) under weak base conditions. | |
Conclusions
We have demonstrated that Lewis acidity of various organoboronates and organoboronamides can be accurately evaluated by theoretical calculation-based ammonia affinity (AA) in a unified manner. In particular, the AA is superior to the FIA in evaluating the boronamide-Lewis acidity, because electron-donation influence from the N atoms to the B center can properly be reflected in the optimized ammonia–phenylboronamide adducts. The predicted Lewis acidity diminishment of the B(mdan) moiety by the AA was reproduced experimentally by its high stability towards hydrolysis, and furthermore its slightly Lewis acidic character (AA = 21.5 kJ mol−1) compared with the B(dan) moiety (AA = 12.1 kJ mol−1) leads to the direct SMC under weak base conditions.54
Author contributions
H. T. and H. Y. conceived the concept and wrote the manuscript. H. T. conducted all theoretical calculations and experiments. M. N. provided advice for theoretical calculations. H. Y. directed the project.
Conflicts of interest
There are no conflicts to declare.
Acknowledgements
This work was financially supported by Fukuoka Naohiko Memorial Foundation and Nagase Science and Technology Foundation. H. T. acknowledges JSPS fellowship for young scientists, JSPS KAKENHI Grant Number JP20J22816 for financial support. The computation was performed using Research Center for Computational Science, Okazaki, Japan (Project: 21-IMS-C254 and 22-IMS-C244). We deeply thank Dr Ryo Tanaka (Hiroshima University) for helpful advice on the FIA calculation.
Notes and references
-
(a) Boronic Acids: Preparation and Applications in Organic Synthesis Medicine and Materials, ed. D. G. Hall, Wiley-VCH, Weinheim, 2nd edn, 2011 Search PubMed;
(b) E. C. Neeve, S. J. Geier, I. A. I. Mkhalid, S. A. Westcott and T. B. Marder, Chem. Rev., 2016, 116, 9091–9161 CrossRef CAS PubMed;
(c) Science of Synthesis Reference Library, Advances in Organoboron Chemistry toward Organic Synthesis, ed. E. Fernández, Thieme, Stuttgart, 2020 Search PubMed.
- H. Noguchi, K. Hojo and M. Suginome, J. Am. Chem. Soc., 2007, 129, 758–759 CrossRef CAS PubMed.
- J. Li and H. Yoshida, Heterocycles, 2021, 102, 1478–1516 CrossRef CAS.
- S. Kamio and H. Yoshida, Adv. Synth. Catal., 2021, 363, 2310–2324 CrossRef CAS.
- H. Ihara, M. Koyanagi and M. Suginome, Org. Lett., 2011, 13, 2662–2665 CrossRef CAS PubMed.
- M. Koyanagi, N. Eichenauer, H. Ihara, T. Yamamoto and M. Suginome, Chem. Lett., 2013, 42, 541–543 CrossRef CAS.
- H. Noguchi, T. Shioda, C.-M. Chou and M. Suginome, Org. Lett., 2008, 10, 377–380 CrossRef CAS PubMed.
- P. Dobrounig, M. Trobe and R. Breinbauer, Monatsh. Chem., 2017, 148, 3–35 CrossRef CAS PubMed.
- H. Yoshida, Y. Takemoto and K. Takaki, Chem. Commun., 2014, 50, 8299–8302 RSC.
- L. Xu and P. Li, Chem. Commun., 2015, 51, 5656–5659 RSC.
- S. Kamio, I. Kageyuki, I. Osaka, S. Hatano, M. Abe and H. Yoshida, Chem. Commun., 2018, 54, 9290–9293 RSC.
- T. Tsushima, H. Tanaka, K. Nakanishi, M. Nakamoto and H. Yoshida, ACS Catal., 2021, 11, 14381–14387 CrossRef CAS.
- Theoretical calculations on selective addition of a base to (pin)B–B(dan) were reported. See:
(a) J. Cid, J. J. Carbó and E. Fernández, Chem.–Eur. J., 2014, 20, 3616–3620 CrossRef CAS PubMed;
(b) S. H. Doan, N. N. H. Ton, B. K. Mai and T. V. Nguyen, ACS Catal., 2022, 12, 12409–12418 CrossRef CAS.
- F. Caserio Jr, J. Cavallo and R. Wagner, J. Org. Chem., 1961, 26, 2157–2158 CrossRef.
- H. Yoshida, M. Kimura, H. Tanaka, Y. Murashige, I. Kageyuki and I. Osaka, Chem. Commun., 2019, 55, 5420–5422 RSC.
- S. Lee, N. Almalki, B. Gabidullin and D. Richeson, Eur. J. Inorg. Chem., 2018, 2702–2708 CrossRef CAS.
- H. Yoshida, Y. Takemoto, S. Kamio, I. Osaka and K. Takaki, Org. Chem. Front., 2017, 4, 1215–1219 RSC.
- S. Kamio, I. Kageyuki, I. Osaka and H. Yoshida, Chem. Commun., 2019, 55, 2624–2627 RSC.
- H. Yoshida, Y. Izumi, Y. Hiraoka, K. Nakanishi, M. Nakamoto, S. Hatano and M. Abe, Dalton Trans., 2022, 51, 6543–6546 RSC.
- C. P. Manankandayalage, D. K. Unruh and C. Krempner, Dalton Trans., 2020, 49, 4834–4842 RSC.
- H. Yoshida, M. Seki, S. Kamio, H. Tanaka, Y. Izumi, J. Li, I. Osaka, M. Abe, H. Andoh, T. Yajima, T. Tani and T. Tsuchimoto, ACS Catal., 2020, 10, 346–351 CrossRef CAS.
- Y. Mutoh, K. Yamamoto and S. Saito, ACS Catal., 2020, 10, 352–357 CrossRef CAS.
- Theoretical evaluation of Lewis acidity is quite important; for some uncertainty in the representative experimental evaluation, Gutmann–Beckett method, see: P. Erdmann and L. Greb, Angew. Chem., Int. Ed., 2022, 61, e202114550 CrossRef CAS PubMed.
- Although FIA has been mainly used for estimating Lewis acidity, estimation of Lewis acidity of some organoboron compounds based on AA has also been reported. See:
(a) L. A. Mück, A. Y. Timoshkin and G. Frenking, Inorg. Chem., 2012, 51, 640–646 CrossRef PubMed;
(b) M. Stojanović and M. Baranac-Stojanović, RSC Adv., 2015, 5, 75895–75910 RSC;
(c) A. b. Saida, A. Chardon, A. Osi, N. Tumanov, J. Wouters, A. I. Adjieufack, B. Champagne and G. Berionni, Angew. Chem., Int. Ed., 2019, 58, 16889–16893 CrossRef PubMed.
- Y. Zhao and D. G. Truhlar, Theor. Chem. Acc., 2008, 120, 215–241 Search PubMed.
- F. Weigend, Phys. Chem. Chem. Phys., 2006, 8, 1057–1065 RSC.
- F. Weigend and R. Ahlrichs, Phys. Chem. Chem. Phys., 2005, 7, 3297–3305 RSC.
- M. J. Frisch, G. W. Trucks, H. B. Schlegel, G. E. Scuseria, M. A. Robb, J. R. Cheeseman, G. Scalmani, V. Barone, G. A. Petersson, H. Nakatsuji, X. Li, M. Caricato, A. V. Marenich, J. Bloino, B. G. Janesko, R. Gomperts, B. Mennucci, H. P. Hratchian, J. V. Ortiz, A. F. Izmaylov, J. L. Sonnenberg, D. Williams-Young, F. Ding, F. Lipparini, F. Egidi, J. Goings, B. Peng, A. Petrone, T. Henderson, D. Ranasinghe, V. G. Zakrzewski, J. Gao, N. Rega, G. Zheng, W. Liang, M. Hada, M. Ehara, K. Toyota, R. Fukuda, J. Hasegawa, M. Ishida, T. Nakajima, Y. Honda, O. Kitao, H. Nakai, T. Vreven, K. Throssell, J. A. Montgomery Jr, J. E. Peralta, F. Ogliaro, M. J. Bearpark, J. J. Heyd, E. N. Brothers, K. N. Kudin, V. N. Staroverov, T. A. Keith, R. Kobayashi, J. Normand, K. Raghavachari, A. P. Rendell, J. C. Burant, S. S. Iyengar, J. Tomasi, M. Cossi, J. M. Millam, M. Klene, C. Adamo, R. Cammi, J. W. Ochterski, R. L. Martin, K. Morokuma, O. Farkas, J. B. Foresman and D. J. Fox, Gaussian 16, Revision A.03, Gaussian, Inc., Wallingford CT, 2016 Search PubMed.
- J. A. Plumley and J. D. Evanseck, J. Phys. Chem. A, 2009, 113, 5985–5992 CrossRef CAS PubMed.
- J. A. Plumley and J. D. Evanseck, J. Chem. Theory Comput., 2008, 4, 1249–1253 CrossRef CAS PubMed.
- P. Erdmann, J. Leitner, J. Schwarz and L. Greb, ChemPhysChem, 2020, 21, 987–994 CrossRef CAS PubMed.
- H. Tanaka, M. Nakamoto and H. Yoshida, ChemRxiv, 2022, preprint, DOI:10.26434/chemrxiv-2021-dvx7s-v2.
- L. Greb, Chem.–Eur. J., 2018, 24, 17881–17896 CrossRef CAS PubMed.
- A. R. Jupp, T. C. Johnstone and D. W. Stephan, Dalton Trans., 2018, 47, 7029–7035 RSC.
- A. R. Jupp, T. C. Johnstone and D. W. Stephan, Inorg. Chem., 2018, 57, 14764–14771 CrossRef CAS PubMed.
- R. J. Mayer, N. Hampel and A. R. Ofial, Chem.–Eur. J., 2021, 27, 4070–4080 CrossRef CAS PubMed.
- R. G. Parr, L. v. Szentpály and S. Liu, J. Am. Chem. Soc., 1999, 121, 1922–1924 CrossRef CAS.
- P. K. Chattaraj, U. Sarkar and D. R. Roy, Chem. Rev., 2006, 106, 2065–2091 CrossRef CAS PubMed.
- P. Erdmann and L. Greb, ChemPhysChem, 2021, 22, 935–943 CrossRef CAS PubMed.
- H. Böhrer, N. Trapp, D. Himmel, M. Schleep and I. Krossing, Dalton Trans., 2015, 44, 7489–7499 RSC.
- A. Adamczyk-Woźniak, M. Jakubczyk, P. Jankowski, A. Sporzyński and P. M. Urbański, J. Phys. Org. Chem., 2013, 26, 415–419 CrossRef.
- A. Adamczyk-Woźniak, M. Jakubczyk, A. Sporzyński and G. Żukowska, Inorg. Chem. Commun., 2011, 14, 1753–1755 CrossRef.
- Although we also calculated water affinity (WA) of the phenylboronic acid derivatives, stable water adducts could not be obtained through geometry optimizations (see ESI† for details).
- The average of two B–X bonds was used for investigating relationship between B–X bonds and various calculated values.
- O to B π-donation in boronate moieties has been well established. See: X. Guo, T. Yang, F. K. Sheong and Z. Lin, ACS Catal., 2021, 11, 5061–5068 CrossRef CAS.
- For relationship between N to B π-donation and B–N bond lengths of organoboronamides, see: ref. 11 and 16.
- For Wiberg bond indices of chemical bonds around boron in organoboronic acid derivatives, see: H. Suzuki, T. Nishikawa, H. Makino and M. Ouchi, Chem. Sci., 2022, 13, 12703–12712 RSC.
-
(a) H. Ghalla, N. Issaoui, F. Bardak and A. Atac, Comput. Mater. Sci., 2018, 149, 291–300 CrossRef CAS;
(b) A. Sagaama, N. Issaoui, O. Al-Dossary, A. S. Kazachenko and M. J. Wojcik, J. King Saud Univ., Sci., 2021, 33, 101606 CrossRef;
(c) A. Ramalingam, S. Sambandam, M. Medimagh, O. Al-Dossary, N. Issaoui and M. J. Wojcik, J. King Saud Univ., Sci., 2021, 33, 101632 CrossRef;
(d) I. Jomaa, N. Issaoui, T. Roisnel and H. Marouani, J. Mol. Struct., 2021, 1242, 130730 CrossRef CAS.
- Geometry optimizations of ammonia adducts were conducted from starting geometries based on fluoride adducts optimized at the M06-2X/def2-SVP level of theory.
- E. I. Davydova, T. N. Sevastianova and A. Y. Timoshkin, Coord. Chem. Rev., 2015, 297–298, 91–126 CrossRef CAS.
- It was reported that the amount of energy required to deform the fragments plays a vital role for determining AA of boron trihalides. See: D. Rodrigues Silva, L. de Azevedo Santos, M. P. Freitas, C. F. Guerra and T. A. Hamlin, Chem.–Asian J., 2020, 15, 4043–4054 CrossRef CAS PubMed.
-
(a) Z. Wu, F. Ban and R. J. Boyd, J. Am. Chem. Soc., 2003, 125, 6994–7000 CrossRef CAS PubMed;
(b) J. Morgan, A. Greenberg and J. F. Liebman, Struct. Chem., 2012, 23, 197–199 CrossRef CAS.
- For hydrogen bonding of the B(aam) moiety, see: ref. 47.
- A prior version of the present article was deposited as a preprint on ChemRxiv. See: H. Tanaka, M. Nakamoto and H. Yoshida, ChemRxiv, 2022, preprint, DOI:10.26434/chemrxiv-2022-tlps8.
|
This journal is © The Royal Society of Chemistry 2023 |