DOI:
10.1039/D2RA07704C
(Paper)
RSC Adv., 2023,
13, 8863-8872
A ternary deep eutectic solvent-modified magnetic mixed iron hydroxide@MIL-101(Cr)-NH2 composite as a sorbent in magnetic solid phase extraction of organochlorine pesticides prior to GC-MS†
Received
3rd December 2022
, Accepted 11th March 2023
First published on 16th March 2023
Abstract
A green solvent of ternary deep eutectic solvent (menthol-thymol-dodecanoic acid) was prepared and used as a functional reagent to modify a magnetic mixed iron hydroxide@MIL-101(Cr)-NH2 composite. The proposed sorbent (MIH@MIL-101(Cr)-NH2-TDES) was applied in magnetic solid phase extraction (MSPE) for the enrichment of organochlorine pesticides. The analytes were quantitively analyzed by GC-MS. The relationships of experimental parameters for preparing the proposed sorbent and the MSPE method were studied through a Box–Behnken design and a central composite design, respectively. Their optimized conditions were investigated using response surface methodology. Application of the MIH@MIL-101(Cr)-NH2-TDES sorbent in MSPE successfully enhanced the sensitivity of GC-MS analysis, giving enrichment factors in the range of 56–168. The MSPE/GC-MS method was developed using MIH@MIL-101(Cr)-NH2-TDES as a sorbent and was successfully employed for the preconcentration/determination of organochlorine residues in honey and tea samples. The satisfactory detection limits were in the ranges of 0.07–0.80 ng g−1 and 0.7–8.5 ng g−1 for honey and tea samples, respectively. Acceptable recoveries were obtained in the ranges of 81.7–107.3% and 85.4–109.3% for the spiked honey and tea samples, respectively, with RSDs lower than 10.0%.
1. Introduction
Organochlorine pesticides (OCPs) have been widely applied as insecticides in agriculture to control or eliminate insects. These compounds are organic pollutants that have high persistence in the environment, low biodegradability and can damage animal and human health,1 producing acute symptoms such as dizziness, nausea, vomiting, myoclonic jerking, motor hyperexcitability, convulsive seizures, and generalized convulsions.2 Therefore, it is necessary to monitor the OCP concentration to control its residual levels in foods and beverages, e.g., honey,3,4 milk,5,6 and tea leaves.7–9 Gas chromatography (GC) equipped with sensitive detectors, such as electron capture detectors (ECDs)4–6 and mass spectrometry (MS),7–9 has been extensively applied for the determination of OCPs. However, sample preparation, preconcentration, isolation, and cleanup were required prior to performing the instrumental analysis.
Magnetic solid phase extraction (MSPE) is an extraction method that has remarkable advantages, such as high extraction efficiency and high adsorption capacity, facile isolation process of two phases, short time for phase isolation and extraction, and environmental friendliness. To improve the detection sensitivity for OCPs, various magnetic sorbents have been proposed for application in MSPE methods, including magnetic cobalt ferrite-filled carbon nanotubes,4 ZnFe2O4/carbon composites,10 and graphene-based magnetic nanocomposites.11 Mixed-iron hydroxide (MIH) has also recently drawn attention because of its small size and large surface area, simple preparation process, low cost, and environmental friendliness.12 The pure magnetic materials tend to self-agglomerate, which is the cause of the reduction in magnetic stability. Modification of the magnetic core avoided this problem and improved its efficiency. Various types of materials have been proposed for modifying magnetic particles, including inorganic materials (silicon dioxide,13 carbon-based materials,14 and metallic materials15), organic materials (polymer-based16 and nonpolymer materials17), and metal–organic frameworks (MOFs).18 MOFs, porous inorganic–organic materials that consist of metal ions and organic linkers, are interesting materials for combining with MIH particles due to their high specific surface area, tunability of surface function and pore sizes. MIL-101(Cr)-NH2, synthesized from chromium(III) ions (as metal centers) and 2-aminoterephthalic acid (as a linker) via a hydrothermal method,19 is one of the interesting MOFs due to its high surface area, rich hydrogen bonding, and lack of organic solvent consumption in its synthesis process.
In addition, modifying the magnetic surface with a functional reagent was significant in improving the efficiency of the magnetic material for preconcentration of the target compound. Deep eutectic solvents (DESs), formed by mixing hydrogen bond acceptors (HBAs) and hydrogen bond donors (HBDs) under heating, are classified as green solvents due to their lack of chemical reactions, lack of waste production, and low consumption of energy in the production process.20 DESs have many advantages, such as good functional ability, simple preparation, low cost, and lower toxicity (compared to traditional solvents). Recently, ternary deep eutectic solvents (TDESs) have been extensively used as functional reagents to enhance the performance of various sorbents. TDE-functionalized sorbents have been reported, such as magnetic-molecularly imprinted polymers (MMIP) using TDES of choline chloride (ChCl), oxalic acid and 1,2-propanediol as monomers for purification of baicalein,21 DES-MIP using caffeic acid–ChCl–formic acid TDES as monomers for purification of paclitaxel,22 and AgNO3–Al(NO3)3·H2O-methylacetamide TDES-based membranes for separation of ethylene and ethane.23 The TDESs showed lower viscosity than binary-DESs, which was the cause of more adhesion on the magnetic surface and led to a large specific surface area.21 Owing to these properties, TDESs were appropriate as a functional reagent to modify the magnetic sorbent for OCP extraction. To the best of our knowledge, no article has proposed the synthesis and application of magnetic MOF composites modified with TDES.
In this study, we prepared, for the first time, a menthol-thymol-dodecanoic acid TDES-modified mixed iron hydroxide-MIL-101(Cr)-NH2 composite (denoted MIH@MIL-101(Cr)-NH2-TDES) via a coprecipitation method. Menthol, thymol and dodecanoic acid are natural compounds that have low toxicity and are environmentally friendly. Modifying the MIH particles with MIL-101(Cr)-NH2 and TDES successfully improved the efficiency of the sorbent, which prevented the self-agglomeration of MIH and increased its active sites. Three subgroups of OCPs, including (i) dichlorodiphenylphenylethanes (dichlorodiphenyldichloroethane (DDD) and dichlorodiphenyl dichloroethylene (DDE)); (ii) chlorinated cyclodienes (aldrin, isobenzan, and heptachlor); and (iii) hexachlorocyclohexanes (HCH), were selected as target analytes. The experimental design methods of Box–Behnken design (BBD) and central composite design (CCD) were applied to study the relationship of the experimental factors for material preparation and MSPE, respectively. These studied conditions were investigated and optimized via response surface methodology (RSM). Finally, the MSPE/GC-MS developed using MIH@MIL-101(Cr)-NH2-TDES as a sorbent was suitable for the determination of OCP residues in honey and tea samples.
2. Materials and methods
2.1 Chemicals and reagents
All the chemicals were of at least analytical grade. The stock standard solutions of each OCP were prepared at 1000 mg L−1 in methanol and stored at 4 °C. The OCPs, including isomers of hexachlorocyclohexane (α-HCH, β-HCH, γ-HCH, and δ-HCH), dichlorodiphenyl dichloroethylene (o,p'-DDE and p,p'-DDE), and dichlorodiphenyl-dichloroethane (o,p'-DDD and p,p'-DDD), were purchased from CPAchem (France). Heptachlor, aldrin and isobenzan were obtained from Dr Ehrenstorfer GmbH (Germany). Iron(II) ammonium sulfate hexahydrate was purchased from Carlo Erba (Italy). Anhydrous iron(III) chloride was purchased from Riedel-de Hean (Germany). (−)-Menthol, thymol (≥98.5%), dodecanoic acid (≥99%) and 2-aminoterephthalic acid (99%) were obtained from Sigma-Aldrich (Germany). Chromium(III) nitrate nonahydrate was purchased from HiMedia Laboratories (India). Hydrochloric acid was purchased from QRëC (New Zealand). Sodium hydroxide and absolute ethanol were purchased from Merck Chemicals (Germany). Methanol (HPLC grade), sodium chloride and ethyl acetate were purchased from Fisher Scientific (Belgium). Acetonitrile (HPLC grade) and acetone were obtained from RCI Labscan (Thailand). The water used in this work was Type I deionized water (18.2 MΩ cm) and was prepared through an RiOs Simplicity 185 ultrapure water system (Millipore, USA).
2.2 Instrumentation
The structure and morphology of the materials were examined using an FEI Helios NanoLab G3 CX DualBeam scanning electron microscope with a focused ion beam (FIB-SEM; Hillsboro, USA) and an FEI Tecnai G2 20 transmission electron microscope (TEM; Hillsboro, USA). A PerkinElmer spectrum two FTIR spectrometer was used to record the Fourier transform infrared spectra (FTIR; 4000–400 cm−1). An X-ray powder diffractometer (XRD; D8 Advance, Bruker, USA) using monochromatic Cu Kα radiation (λ = 0.15406 nm, 2θ = 5°–70°) was used for crystal structure characterization. The magnetic properties of the materials were investigated by a Lake Shore vibrating sample magnetometer (VSM 7403, USA) with an applied magnetic field (H) of ±10
000 Oersted (Oe). A PE 2400 CHNS analyzer (PerkinElmer, USA) was used for elemental analysis. A thermogravimetric analyzer (TGA; Hitachi, STA7200, Japan) was conduced to investigate the thermal stability of the prepared materials at the temperature range of 50–700 °C with heating rate of 10 °C min−1 under N2 atmosphere.
Separation and quantification of OCPs were carried out using an Agilent 8890 gas chromatograph equipped with an HP-5MS ultra inert fused silica capillary column (30 m × 250 μm × 0.25 μm film thickness), a 5977B mass spectrometer and a 7693A automatic liquid sampler (Agilent Technology, USA). Quantitative data acquisition and processing were performed using MassHunter Software (Agilent Technology, USA). Helium (99.999%) was used as the carrier gas at a flow rate of 1.0 mL min−1. The injection volume was 1 μL with split mode at a ratio of 10
:
1. The column temperature program was set as follows: initial temperature, 160 °C; raised to 200 °C at a heating rate of 20 °C min−1; slowly increased to 250 °C at 5 °C min−1; and finally heated to 270 °C at 20 °C min −1 and held for 1 min. The transfer line, electron ionization source and quadrupole temperatures were maintained at 270 °C, 230 °C and 150 °C, respectively. Selective ion monitoring (SIM) was used for the quantitative analyses of the OCPs, as listed in Table 1.
Table 1 Method validation of the proposed MSPE/GC-MS using MIH@MIL-101(Cr)-NH2 as a sorbent and the selected ion for monitoring organochlorine pesticides
Analyte |
m/z |
Linear range (ng L−1) |
R2 |
LODs (ng L−1) |
LOQs (ng L−1) |
RSDsa |
EFs |
Intraday (n = 5) |
Interday (n = 5 × 3) |
Investigated at 1000 ng L−1 of each organochlorine pesticide. |
α-HCH |
183, 181, 219 |
7.5–50,000 |
0.9983 |
5.0 |
7.5 |
2.8 |
4.3 |
71 |
β-HCH |
109, 181, 219 |
2.5–50,000 |
0.9985 |
1.5 |
2.5 |
2.4 |
5.9 |
75 |
γ-HCH |
109, 181, 219 |
10.0–50,000 |
0.9986 |
2.5 |
10.0 |
2.7 |
5.3 |
58 |
δ-HCH |
109, 183, 219 |
25.0–50,000 |
0.9969 |
10.0 |
25.0 |
3.4 |
7.7 |
56 |
Heptachlor |
100, 272, 274 |
7.5–50,000 |
0.9957 |
5.0 |
7.5 |
2.8 |
7.5 |
128 |
Aldrin |
66, 241, 263 |
2.5–50,000 |
0.9990 |
1.0 |
2.5 |
2.8 |
4.4 |
153 |
Isobenzan |
103, 263, 311 |
7.5–50,000 |
0.9985 |
5.0 |
7.5 |
3.8 |
4.0 |
168 |
o,p'-DDE |
246, 248, 318 |
1.0–50,000 |
0.9993 |
0.4 |
1.0 |
4.7 |
4.8 |
166 |
p,p'-DDE |
246, 248, 318 |
1.0–50,000 |
0.9988 |
0.4 |
1.0 |
3.3 |
6.3 |
168 |
o,p'-DDD |
165, 235, 237 |
1.0–50,000 |
0.9971 |
0.8 |
1.0 |
3.9 |
8.0 |
149 |
p,p'-DDD |
165, 235, 237 |
25.0–50,000 |
0.9963 |
15.0 |
25.0 |
5.7 |
5.8 |
133 |
2.3 Synthesis of MIH@MIL-101(Cr)-NH2-TDES
To prepare TDES, menthol, thymol and dodecanoic acid were mixed with different molar ratios, as listed in Table S1,† under heating at 40–60 °C until a clear and homogeneous liquid was formed.
MIL-101(Cr)-NH2 was synthesized through a hydrothermal method as described in the literature.19 Briefly, 2-aminoterephthalic acid (0.72 g), Cr(NO3)3·9H2O (1.6 g) and NaOH (0.4 g) were dissolved in 30 mL of water. The mixture was placed in an autoclave and heated at 150 °C for 12 h. The green particles were subsequently obtained and washed with water and ethanol. The product was dried at 80 °C overnight.
For MIH@MIL-101(Cr)-NH2-TDES preparation (illustrated in Fig. 1), the dried MIL-101(Cr)-NH2 particles were dispersed in 40 mL of water via ultrasonication. The prepared TDES was added and stirred. Then, an aqueous solution of Fe(NH4)2(SO4)2·6H2O and FeCl3 anhydrous (40 mL; 1.95 g and 0.4 g, respectively) was added and continuously stirred for 10 min. After that, NaOH solution (10 mL, 3 mol L−1) was immediately added to adjust the pH to 10. The precipitation of MIH particles grafted onto MIL-101(Cr)-NH2 was obtained. The magnetic sorbent was collected by an external magnet after stirring for 30 min, washed several times with water and dried at 80 °C overnight.24 In addition, MIH@MIL-101(Cr)-NH2 and MIH–TDES were prepared in the same manner as MIH@MIL-101(Cr)-NH2-TDES without adding TDES and MIL-101(Cr)-NH2, respectively.
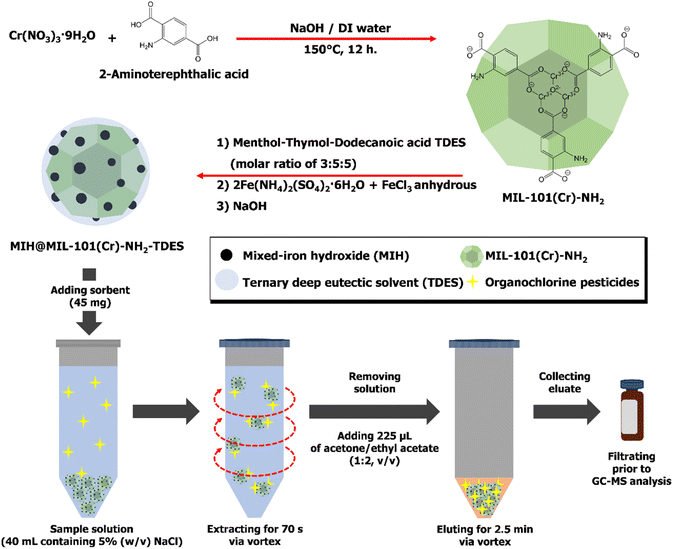 |
| Fig. 1 Schematic diagram for the synthesis of MIH@MIL-101(Cr)-NH2-TDES and the magnetic solid phase extraction procedure for organochlorine pesticides. | |
2.4 Experimental design
The synthesis conditions of the MIH@MIL-101(Cr)-NH2-TDES sorbent, including the amount of MIL-101(Cr)-NH2, volume of TDES and interaction time, were studied via BBD. The important parameters of MSPE were investigated via CCD. These studied conditions were optimized using RSM through Design-Expert 13 software. The experimental requirements of BBD and CCD were calculated according to the Equations N = 2k(k − 1) + cp and N = k2 + 2k + cp, respectively, where k is the number of factors and cp is the number of the central point.25 Each experiment was carried out in triplicate (n = 3).
2.5 MSPE procedure
An illustration of the MSPE procedure is shown in Fig. 1. Briefly, the MIH@MIL-101(Cr)-NH2-TDES sorbent (45 mg) was added to a 50 mL centrifuge tube containing sample solution (40 mL) and sodium chloride (2 g in 40 mL, 5% (w/v)). The target analytes were extracted onto the sorbent within 70 s by vortexing, and then the solution was discarded. After that, the mixed solvent of acetone/ethyl acetate (225 μL; 1
:
2 (v/v)) was added to elute the adsorbed analytes from the sorbent for 2.5 min. Finally, the eluate was filtered through a 0.22 μm Nylon membrane filter before being subjected to GC-MS.
2.6 Pretreatment of real samples
Honey samples (5 g), purchased from local markets (Khon Kaen, Thailand), were diluted with water (100 mL). The solution was filtered through Whatman (No. 1) filter paper and kept at 4 °C until analysis.
Three types of tea samples, including Wulong, Biluochun and Longjing tea leaves, were obtained from local markets (Khon Kaen, Thailand). After drying at 40 °C overnight, the dried tea leaves were pounded to obtain tea powder. Ethyl acetate (10 mL) was added to an Erlenmeyer flask containing 2 g of the tea powder and then sonicated for 30 min. The suspension was filtered through Whatman (No. 1) filter paper. The supernatant was dried under N2 gas and then redissolved with 10 mL of acetonitrile. Finally, the solution was diluted to 100 mL with water and kept at 4 °C until analysis.
3. Results and discussion
3.1 Choice of magnetic sorbent
To obtain a highly efficient sorbent for the enrichment of OCPs, various types of magnetic sorbents modified with MIL-101(Cr)-NH2 and TDES were studied under the MSPE conditions as follows: sorbent, 30 mg; extraction/desorption times, 30 s; desorption solvent, 500 μL of ethyl acetate; and sample volume, 10 mL containing 50 μg L−1 of each OCP. In this study, the preconcentration efficiency was reported in terms of the enrichment factor (EF), and calculated according to the equation EF = Cf/Ci, where Cf is the concentration of analytes in a final eluate and Ci is the initial analyte concentration in the sample.
3.1.1 TDES compositions. Various molar ratios of menthol, thymol and dodecanoic acid were studied to prepare different TDESs (as listed in Table S1†) and modified on the magnetic materials. The results (Fig. S1†) show that increasing the mole fraction of menthol or thymol (TDES2, TDES3, TDES4 and TDES5) led to the enhancement of the extraction efficiency of less than that of TDES1. On the other hand, increasing the fatty acid (TDES6) can effectively improve the extraction efficiency of the sorbent. The mole fractions of TDESs are shown in Fig. S2.† In general, the polarity of DESs depends on the properties of their individual components, meaning that DESs consisting of hydrophobic compounds will be low-polarity solvents. The hydrophobicity/hydrophilicity of TDESs was roughly considered using the log[P] values of their compositions, as listed in Table S1.† Due to the higher hydrophobicity of TDES6 resulting from the larger mole fraction of dodecanoic acid, the TDES6-modified magnetic sorbent can increasingly interact with the analytes via hydrophobic interactions. However, an excess mole fraction of the fatty acid in TDES causes an unstable solvent at room temperature, which was observed using molar ratios of menthol
:
thymol
:
dodecanoic acid of 1
:
1
:
2, 1
:
1
:
3 and 1
:
3
:
5, respectively (data not shown). Therefore, the MIH@MIL-101(Cr)-NH2-TDES6 sorbent, prepared from menthol
:
thymol
:
dodecanoic acid at a molar ratio of 3
:
5
:
5 (denoted TDES in other sections), gave the highest preconcentration performance for OCPs and was selected as the MSPE sorbent in this study.
3.1.2 Box–Behnken design. The synthesis conditions of the MIH@MIL-101(Cr)-NH2-TDES sorbent, including the amount of MIL-101(Cr)-NH2 (x1), volume of TDES (x2) and interaction time (x3), were optimized using BBD and RSM. Three independent factors were assigned at 3 levels (−1, 0, 1), as shown in Table S2.† For the three-factor design, 15 experimental runs were required (cp = 3). The experimental conditions and their responses are summarized in Table S3.† The relationship of the three independent parameters is shown in eqn (1). |
EF = 25.50 + 1.08 x1 + 9.29 x2 + 1.41 x3 + 0.2025 x1 x2 − 0.3 x1 x3 − 0.1675 x2 x3 − 5.21 x12 − 7.83 x22 − 0.665 x32
| (1) |
In this study, analysis of variance (ANOVA) of the Box–Behnken design based on a quadratic model was investigated, and the results are summarized in Table S4.† The lack of fit (LOF) was 0.0710, indicating that it was not significant relative to the pure error. Terms of the quadratic model, including x2, x3, x12, and x22, were important terms due to their P values being less than 0.05. The R2 and adjusted R2 were 0.9929 and 0.9802, respectively.
The synthesis conditions of the MIH@MIL-101(Cr)-NH2-TDES sorbent were optimized using the Design-Expert 13 program. The relationship of the three parameters is shown in Fig. 2. Increasing the amount of MOF improved the extraction efficiency (Fig. 2A). However, the extraction performance was reduced using MOF over 650 mg due to the possible loss of MOF particles from the magnetic surface in solution during the extraction process. The extraction efficiency of the sorbent increased when the hydrophobic DES on the sorbent was raised owing to the stronger interactions between the sorbent and the analytes. However, the excessive volume of TDES on the sorbent surface (over 0.820 mL) may reduce the dispersibility of the sorbent in aqueous solution, which results in a decrease in extraction efficiency. The result in Fig. 2B shows the effect of time, in which the extraction efficiency increased with increasing interaction time from 30 to 70 min, and then the efficiency remained almost constant. Therefore, the proper conditions for synthesis of the sorbent included 650 mg of MIL-101(Cr)-NH2 particles, 820 μL of TDES and 70 min for interaction time.
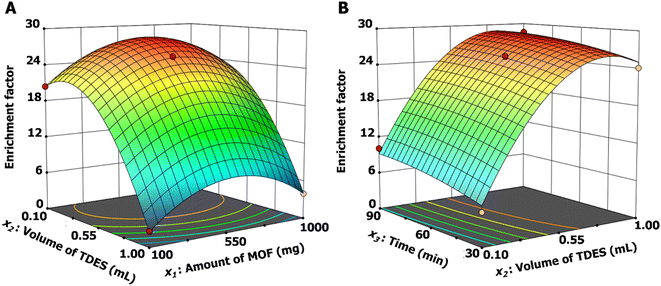 |
| Fig. 2 Three-dimensional response surface plots of the relationship between (A) amount of MIL-101(Cr)-NH2 and TDES volume and (B) TDES volume and stir time. The magnetic solid phase extraction conditions were as follows: sorbent, 30 mg; extraction/desorption time, 30 s; desorption solvent, 0.5 mL of ethyl acetate; and sample volume, 10 mL containing 50 μg L−1 of each organochlorine pesticide. | |
3.2 Characterization
The morphologies of the prepared materials, including MIL-101(Cr)-NH2, MIH, MIH@MIL-101(Cr)-NH2 and MIH@MIL-101(Cr)-NH2-TDES, were investigated, as shown in Fig. 3. MIH@MIL-101(Cr)-NH2 and MIH@MIL-101(Cr)-NH2-TDES have magnetic particles trapped on the spheroidal shape of MIL-101(Cr)-NH2. Moreover, the surface of MIH@MIL-101(Cr)-NH2-TDES was rougher than that of bare MIL-101(Cr)-NH2 and MIH@MIL-101(Cr)-NH2, indicating that the magnetic material was successfully modified with TDES.
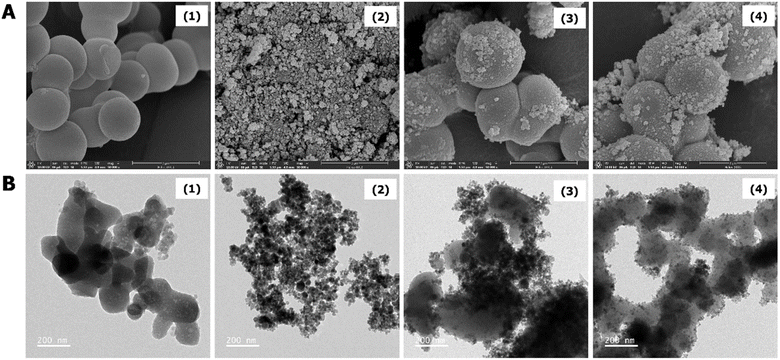 |
| Fig. 3 (A) SEM and (B) TEM images of (1) MIL-101(Cr)-NH2, (2) MIH, (3) MIH@MIL-101(Cr)-NH2, and (4) MIH@MIL-101(Cr)-NH2-TDES. | |
Fig. 4A shows the FTIR spectra of the as-prepared sorbents and TDES. The broad peak in the range of 3670–3230 cm−1 was –OH stretching from MIL-101(Cr)-NH2, MIH and TDES, which can be observed in the FTIR spectra of the proposed magnetic sorbent (as shown in Fig. 4A(4)). The peaks at 1620 cm−1 and 1258 cm−1 corresponded to the N–H bending vibration of amino groups and the C–O stretching vibration from MIL-101(Cr)-NH2, respectively.19 The peaks of C
C stretching and the C–H bending vibration of the aromatic ring appeared at 1561 cm−1 and 768 cm−1, respectively. The peaks of the O–H bending vibration and C–C stretching vibration from MIL-101(Cr)-NH2 were observed at 1428 cm−1 and 1386 cm−1, respectively. The Fe–O stretching vibration appeared at 635 cm−1 and 582 cm−1 from MIH particles.24 The peaks at 2923 cm−1 and 2854 cm−1 were –CH2 asymmetrical stretching and –CH3 stretching of menthol-thymol-dodecanoic acid TDES. The peak at 1705 cm−1 corresponded to the C
O stretching vibration from TDES. All these peaks were observed in the FTIR spectra of the sorbent, as shown in Fig. 4A(4). Thus, the MIH@MIL-101(Cr)-NH2-TDES sorbent was successfully prepared.
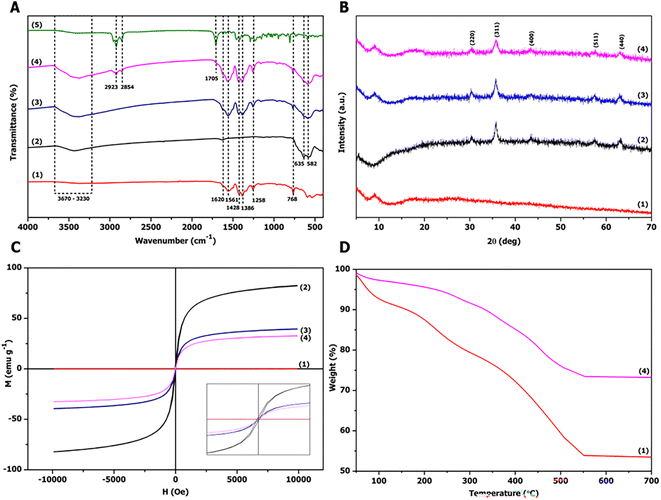 |
| Fig. 4 (A) FTIR spectra, (B) XRD patterns, (C) magnetic properties, and (D) Thermogravimetric analysis of (1) MIL-101(Cr)-NH2, (2) MIH, (3) MIH@MIL-101(Cr)-NH2, (4) MIH@MIL-101(Cr)-NH2-TDES and (5) TDES. | |
Fig. 4B shows the XRD patterns of the as-prepared sorbent. The diffraction peaks of MIH at 2θ values of 63.22° (440), 57.53° (511), 43.41° (400), 35.72° (311), and 30.40° (220) corresponded to Fe3O4 (JCPDS 26-1136) and Fe2O3 (JCPDS 39-1346). The broad peak at approximately 8.90° of MIL-101(Cr)-NH2 appeared in the XRD pattern of the magnetic sorbent (Fig. 4B(4)), indicating that the MIH@MIL-101(Cr)-NH2 composite was successfully prepared. The crystalline structure of MIH remained unchanged after modification.
The magnetic properties of MIL-101(Cr)-NH2, MIH, MIH@MIL-101(Cr)-NH2, and MIH@MIL-101(Cr)-NH2-TDES are shown in Fig. 4C. The magnetic materials exhibited ferromagnetic properties due to their small hysteresis loop, as shown in the inset. MIL-101(Cr)-NH2 showed no magnetism, and its saturation magnetization (Ms) increased to 39.52 emu g −1 after modification with magnetic MIH particles. The saturation magnetization of MIH was reduced from 81.25 emu g−1 to 32.85 emu g−1 due to modification with nonmagnetic MIL-101(Cr)-NH2 and TDES. However, the as-prepared sorbent has strong magnetic properties and can be easily isolated under an external magnet from the liquid phase in the MSPE procedure.
The elemental compositions of carbon, hydrogen, and nitrogen in MIL-101(Cr)-NH2, MIH@MIL-101(Cr)-NH2 and MIH@MIL-101(Cr)-NH2-TDES were investigated by CHN analysis. The percentages of C
:
H
:
N in MIL-101(Cr)-NH2, MIH@MIL-101(Cr)-NH2 and MIH@MIL-101(Cr)-NH2-TDES were found to be 27.22
:
3.81
:
4.71, 7.18
:
1.67
:
1.74 and 19.62
:
2.37
:
1.70, respectively. The results indicated that the MIH@MIL-101(Cr)-NH2-TDES sorbent was successfully synthesized.
The thermal stability of the prepared materials was studied using TGA method, as the results shown in Fig. 4D. At the temperature range of 50–100 °C, MIL-101(Cr)-NH2 and MIH@ MIL-101(Cr)-NH2-TDES show the first weight loss of 7.3% and 2.7%, respectively, due to their moisture.30 The materials show continuous mass loss in the temperature range up to 550 °C. The final weights of MIL-101(Cr)-NH2 and MIH@ MIL-101(Cr)-NH2-TDES were 53.9% and 73.5%, respectively. These results indicated that the MIH@MIL-101(Cr)-NH2-TDES sorbent has higher thermal stability than the MIL-101(Cr)-NH2, owing to high thermal stability of Fe3O4 and Fe2O3 in MIH particles.31,32
3.3 Optimization of the MSPE procedure
3.3.1 Extraction process. In the MSPE process, five parameters (sorbent amount, pH of the sample, salt addition, extraction time and sample volume) were studied to screen their effect on extraction efficiency via a one-factor-at-a-time method, as shown in Fig. S3.† The study found that all parameters influenced the extraction efficiency except the pH of the sample. The results in Fig. S3B† demonstrated either no positive results or no effect on the extraction efficiency using acidic/basic sample solutions. Therefore, the pH of the sample solution did not need to be adjusted prior to extraction.All the important parameters, including sorbent amount (10–70 mg), salt addition (0–10% w/v), extraction time (10–90 s), and sample volume (10–50 mL), were optimized, and their relationships were studied via experimental design using CCD coupled to RSM. Four variables were studied at 5 levels (−2, −1, 0, +1, +2), as shown in Table S2.† A total of 30 runs were required (cp = 6). The response results of all the experiments are shown in Table S3.† The relationship of the four variables is shown in eqn (2). ANOVA of the CCD fitted with the quadratic model is shown in Table S4.† The LOF was 0.1070, indicating that there was no significant difference relative to the pure error. Terms of the quadratic model, including X1, X2, X3, X4, X1X3, X1X4, X2X3, X2X4, X3X4, X12, X22, and X42, were important terms (p < 0.05). The R2 and adjusted R2 were 0.9863 and 0.9734, respectively.
|
EF = 47.21 + 1.81X1 + 0.96X2 + 1.66X3 + 6.94X4 + 0.57X1X2 + 2.14X1X3 + 1.90X1X4 + 2.72X2X3 − 2.38X2X4 + 1.03X3X4 − 3.25X12 − 4.67X22 + 0.14X32 − 3.49X42
| (2) |
Three-dimensional response surface and contour plots (Fig. 5) indicated the interaction of each factor. The extraction efficiency increased with increasing sorbent mass and then remained almost constant after using over 45 mg of sorbent. The EF increased with increasing sample volume from 10 to 40 mL due to the high content of the analytes. Owing to the limitation of the adsorption capacity of the sorbent, the EF value remained constant after extracting a volume larger than 40 mL (Fig. 5(A and B)). Generally, the addition of salt is the cause of the reduced solubility of analytes in aqueous solution, called the “salting-out effect.” The results in Fig. 5(C and D) present the effect of ionic strength on the extraction efficiency. Adding NaCl salt from 0–5% enhanced the extraction efficiency due to higher analyte extracted to the sorbent. After the addition of salt over 5%, the EF value decreased because the higher viscosity of the solution hindered analyte contact with the sorbent. Moreover, increasing the extraction time improved the extraction efficiency, and the highest EF was obtained with an extraction time of 70 s. Therefore, the optimized MSPE conditions included 45 mg of sorbent, 5% (w/v) NaCl, 70 s of extraction time and 40 mL of sample solution. The other MSPE conditions were kept as follows: desorption time, 30 s; and desorption solvent, 0.5 mL of ethyl acetate.
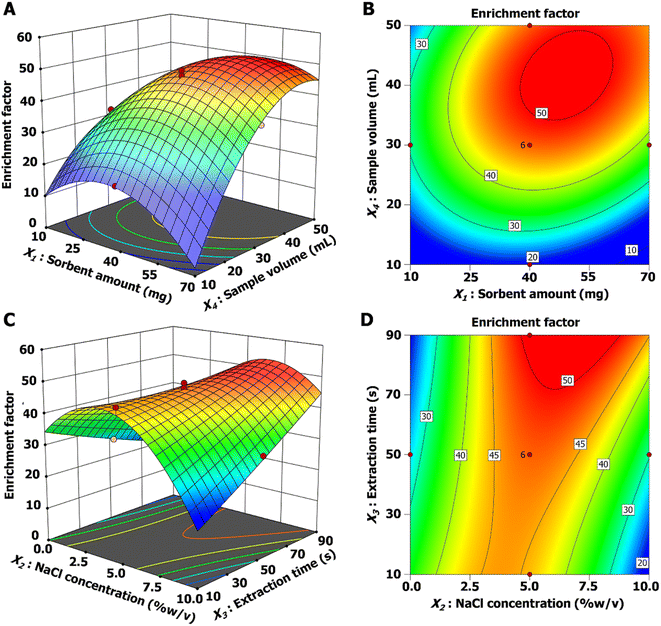 |
| Fig. 5 Three-dimensional response surface and contour plots of the relationship between (A) sorbent amount and sample volume and (B) salt addition and extraction time. The desorption conditions were as follows: desorption time, 30 s; desorption solvent, 0.5 mL of ethyl acetate. | |
3.3.2 Desorption process. Selection of a proper solvent is important in the MSPE method. The solvents tested in this study included acetonitrile, methanol, acetone, ethyl acetate and mixed solvent (acetonitrile/ethyl acetate, 1
:
1 (v/v); methanol/ethyl acetate, 1
:
1 (v/v); and acetone/ethyl acetate, 1
:
1, 1
:
2, and 1
:
3 (v/v)). These solvents (0.5 mL) were tested for desorption of OCPs (2 min) under the optimized extraction conditions. The results in Fig. S4A† indicated that the mixed solvent of acetone/ethyl acetate (1
:
2, v/v) was a suitable desorption solvent that was used in the subsequent experiments. The volume of the solvent was one of the major factors such that a sufficient volume was able to elute all the analytes from the sorbent surface. However, the excess volume of desorption solvent influenced the dilution of the analytes and reduced the preconcentration performance of the extraction method. A volume in the range of 200–400 μL was studied (Fig. S4B†). At least 200 μL of acetone/ethyl acetate (1
:
2, v/v) can be used for eluting the analytes within 2 min. EFs of most OCPs reached the highest value using 225 μL of the mixed solvent, which was selected for this study. Furthermore, the desorption time was investigated in the range of 1–3 min (Fig. S4C†). The EF values increased with increasing desorption time and remained constant after 2.5 min. Thus, the optimized conditions of the desorption process were 225 μL of acetone/ethyl acetate (1
:
2 (v/v)) as eluent and 2.5 min for desorption of OCPs.
3.4 Extraction ability of MIH@MIL-101(Cr)-NH2-TDES
The goals of modifying the MIH particles with MIL-101(Cr)-NH2 and TDES were to prevent self-agglomeration of the magnetic materials and to improve the extraction efficiency for OCPs. To confirm the achievement of these purposes, the as-prepared materials MIH, MIH@MIL-101(Cr)-NH2, MIH-TDES, and MIH@MIL-101(Cr)-NH2-TDES were tested under the optimized MSPE conditions. The results in Fig. S5† demonstrated that MIH@MIL-101(Cr)-NH2-TDES gave higher efficiency than the others. This indicated that modifying magnetic particles with MIL-101(Cr)-NH2 and TDES can improve the sorbent property for enrichment of OCPs due to increasing active sites or interactions between the sorbent and OCPs (i.e., hydrophobic interactions, hydrogen bonding, π–π interactions and π–σ interactions).18
3.5 Method validation
The validation parameters of the proposed MSPE/GC-MS method were studied, including linear range, coefficient of determination (R2), limits of detection (LODs), limits of quantitation (LOQs), and precision (intra- and interday). The results shown in Table 1 demonstrated that good analytical performance of the proposed method was obtained with wide linear ranges of 1.0–50,000 ng L−1 and R2 greater than 0.995. The LODs and LOQs, which were calculated based on signal-to-noise ratios (S/N) of 3 and 10, respectively, were in the range of 0.4–15.0 ng L−1 and 1.0–5.0 ng L−1, respectively. Relative standard deviations (RSDs) indicated that the precision of the method was lower than 5.7% and 8.0% for intra- (n = 5) and interday (n = 5 × 3), respectively. High EFs in the range of 56–168 were reached.
The analytical performance of the proposed method for the determination of OCPs in honey and tea samples is listed in Table S5.† The calibration curves were linear in the ranges of 0.10–1000 ng g−1 and 1.0–2500 ng g−1 with R2 greater than 0.994 for honey and tea samples, respectively. LODs were observed in the ranges of 0.07–0.80 ng g−1 and 0.7–8.5 ng g−1 for honey and tea samples, respectively. LOQs in the range of 0.10–1.00 ng g−1 for honeys and 1.0–10.0 ng g −1 for tea leaves were obtained. For honey samples, the intra- and interday precision in terms of RSDs was below 7.6% and 7.9%, respectively. For tea leaves, RSDs below 7.7% and 8.2% for intra- and interday, respectively, were obtained.
3.6 Application to real samples
The MSPE/GC-MS method developed using MIH@MIL-101(Cr)-NH2-TDES as a sorbent was applied to determine OCP residues in honey and tea samples. To investigate the matrix effects from the real samples, matrix-matched calibration curves were studied. Matrix effects (MEs) of each sample were calculated according to previous studies26 and are summarized in Table S6.† For honey samples No. 1–3, the ME values were in the ranges of −7.8% to −67.8%, −11.2% to −82.5% and −6.5% to −71.0%, respectively. The ME values in the ranges of −0.4% to −50.8%, −3.7% to −39.9% and −5.6% to −38.3% were obtained for Wulong, Biluochun and Longjing tea leaves, respectively. These results indicated that the honey and tea samples showed no matrix effect (not more than −20%) for analyzing some OCPs and showed medium (−20% to −50%) and strong (below −50%) matrix effects for analyzing most OCPs.27 For the determination of OCPs in the samples, all blank honey and tea sample solutions had no contamination of organochlorine residues. To study the accuracy of the proposed method, honey samples spiked at 1.00, 2.00 and 4.00 ng g−1 and tea samples spiked at 10.0, 15.0 and 20.0 ng g−1 were investigated. The acceptable recoveries of OCPs in the spiked honey and tea samples were obtained in the ranges of 81.7–107.3% and 85.4–109.3% (with RSDs less than 10.0%), respectively, as shown in Table S7 and S8.†
3.7 Reusability of the sorbent
To study the reusability of the MIH@MIL-101(Cr)-NH2-TDES sorbent for the enrichment of OCPs, the sorbent used was washed with a mixture of acetone/ethyl acetate (1
:
2, v/v; 0.5 mL × 3) and water (1 mL × 3) before extraction in the new cycle. The results in Fig. S6† indicated that the sorbent cloud could be reused at least seven times without significant loss of the recoveries (RSDs < 10%). These results confirmed the good stability and reusability of the sorbent.
3.8 Comparison of the proposed MSPE/GC-MS with other methods
The proposed MSPE/GC-MS method using MIH@MIL-101(Cr)-NH2-TDES as a sorbent was compared with other reported methods for analyzing OCP residues in honeys, tea leaves and other samples (Table S9†). The proposed MSPE method required a significantly shorter extraction time than the dispersive-SPE using CD-MOF/TiO2 sorbent,3 the MSPE using magnetic cobalt ferrite-CNTs sorbent,4 the ultrasound-assisted extraction using porous membrane8 and the other MSPE methods using magnetic-MOF sorbents (including Fe3O4-NH2@MIL-101(Cr),18 M-M-ZIF-67,28 and Fe3O4@PDA@Zr-SO3H29). Moreover, the proposed method has better analytical performance (such as a wider linear range, lower LODs, and higher accuracy) than the previously mentioned studies. Compared to other methods that use magnetic-MOF as a sorbent,7,18,28,29 the proposed method can simultaneously determine different subgroups of OCPs. Consequently, the developed MSPE/GC-MS using MIH@MIL-101(Cr)-NH2-TDES as a sorbent is a facile, highly sensitive and effective method.
4. Conclusion
MIH@MIL-101(Cr)-NH2 composites modified with menthol-thymol-dodecanoic acid TDES were successfully synthesized and applied to the enrichment of OCPs. The new TDES was prepared from a mixture of three natural compounds, menthol, thymol and dodecanoic acid, at a molar ratio of 3
:
5
:
5. Experimental design based on Box–Behnken design was used to study the relationships of each factor for preparation of the sorbent. Moreover, the optimized MSPE conditions were investigated via central composite design and response surface methodology. The MSPE/GC-MS method developed shows good analytical performance. It can be applied to determine the OCPs in honey and tea leaves. The MIH@MIL-101(Cr)-NH2-TDES sorbent was employed as a promising sorbent for the enrichment/extraction of organochlorine residues in various matrices.
Author contributions
Preeyaporn Phosiri: conceptualization, methodology, investigation, writing – original draft. Prachathipat Pongpinyo: resources. Yanawath Santaladchaiyakit: investigation, visualization. Rodjana Burakham: conceptualization, supervision, funding acquisition, project administration, resources, writing – review and editing.
Conflicts of interest
The authors declare that there are no conflicts of interest.
Acknowledgements
The authors gratefully acknowledge the financial support from the Royal Golden Jubilee (RGJ) PhD program (Grant No. NRCT5-RGJ63003-060). R. Burakham thanks the National Research Council of Thailand (NRCT) and Khon Kaen University for the Mid-Career Research Grant (NRCT5-RSA63003-05). The Materials Chemistry Research Center, Research and Graduate Studies, Khon Kaen University, is also acknowledged.
References
- FAO/WHO, Food and Agriculture Organization/World Health Organization, Food standards program. Codex Alimentarius Commission, Twenty-seventh Session, 28 June-03 July 2004, Geneva, Switzerland, 2004 Search PubMed.
- R. K. Gupta and R. C. Gupta, Chapter 68 – Placental Toxicity, Reproductive and Developmental Toxicology, 2017, pp. 1301–1325 Search PubMed.
- X. Sun, Z. Fu, T. Jiang, F. Ning, Y. Cheng, T. Fu, M. Zhu, H. Zhang, M. Zhang and P. Hu, J. Chromatogr. A, 2022, 1663, 462750 CrossRef CAS PubMed.
- Z. Du, M. Liu and G. Li, J. Sep. Sci., 2013, 36, 3387–3394 CrossRef CAS PubMed.
- M. Wu, G. Chen, P. Liu, W. Zhou and Q. Jia, Analyst, 2016, 141, 243–250 RSC.
- A. Lobato, V. C. Fernandes, J. G. Pacheco, C. Delerue-Matos and L. M. Gonçalves, J. Chromatogr. A, 2021, 1636, 461797 CrossRef CAS PubMed.
- M. Sajid and K. Alhooshanl, Microchem. J., 2020, 152, 104464 CrossRef CAS.
- Y. Zhou, J. Zhu, J. Yang, Y. Lv, Y. Zhu, W. Bi, X. Yang and D. D. Y. Chen, Anal. Chim. Acta, 2019, 1066, 49–57 CrossRef CAS PubMed.
- B. Y. Durak, D. S. Chormey, M. Firat and S. Bakirdere, Food Chem., 2020, 305, 125487 CrossRef CAS PubMed.
- S. Xia, Z. Cai, J. Dong, S. Wang, Y. Wang, H. Kang and X. Chen, J. Chromatogr. A, 2018, 1567, 73–80 CrossRef CAS PubMed.
- T. Sun, J. Yang, L. Li, X. Wang, X. Li and Y. Jin, Chromatographia, 2016, 79, 345–353 CrossRef CAS.
- H. B. Li, Y. Q. Gao and G. W. Yang, RSC Adv., 2015, 5, 45359–45367 RSC.
- Z. Lu, J. Dai, X. Song, G. Wang and W. Yang, Colloids Surf., A, 2008, 317, 450–456 CrossRef CAS.
- Y. Yao, S. Miao, S. Liu, L. P. Ma, H. Sun and S. Wang, Chem. Eng. J., 2012, 184, 326–332 CrossRef CAS.
- C. L. Zhu, M. L. Zhang, Y. J. Qiao, G. Xiao, F. Zhang and Y. J. Chen, J. Phys. Chem. C, 2010, 114, 16229–16235 CrossRef CAS.
- B. Liu, W. Zhang, F. Yang, H. Feng and X. Yang, J. Phys. Chem. C, 2011, 115, 15875–15884 CrossRef CAS.
- X. Zhao, Y. Cai, F. Wu, Y. Pan, H. Liao and B. Xu, Microchem. J., 2011, 98, 207–214 CrossRef CAS.
- X. He, Y. Zhou, W. Yang, S. Li, T. Liu, T. Wang and X. Hou, Talanta, 2019, 196, 572–578 CrossRef CAS PubMed.
- S. Y. Chong, T. T. Wang, L. C. Cheng, H. Y. Lv and M. Ji, Langmuir, 2019, 35, 495–503 CrossRef CAS PubMed.
- F. M. Fuad, M. M. Nadzir and A. H. Kamaruddin, J. Mol. Liq., 2021, 339, 116923 CrossRef.
- D. Wang, X. Luo, Y. Huang, M. Wang and Z. Xia, Microchem. J., 2020, 157, 105109 CrossRef CAS.
- L. Tan, L. D. Zhou, Z. F. Jiang, R. R. Ma, J. Y. He, Z. N. Xia, Q. H. Zhang, C. Z. Wang and C. S. Yuan, J. Pharm. Biomed. Anal., 2021, 192, 113661 CrossRef CAS PubMed.
- B. Jiang, J. Zhou, M. Xu, H. Dou, H. Zhang, N. Yang and L. Zhang, J. Membr. Sci., 2020, 610, 118243 CrossRef CAS.
- P. Phosiri and R. Burakham, J. Sep. Sci., 2021, 44, 3636–3645 CrossRef CAS PubMed.
- M. A. Bezerra, R. E. Santelli, E. P. Oliveira, L. S. Villar and L. A. Escaleira, Talanta, 2008, 76, 965–977 CrossRef CAS PubMed.
- P. Phosiri, Y. Santaladchaiyakit and R. Burakham, J. Chromatogr. A, 2022, 1673, 463111 CrossRef CAS PubMed.
- A. M. Domínguez, F. Placencia, F. Cereceda, X. Fadic and W. Quiroz, J. Agric. Res., 2014, 74, 148–156 Search PubMed.
- X. Huang, G. Liu, D. Xu, X. Xu, L. Li, S. Zheng, H. Lin and H. Gao, Appl. Sci., 2018, 8, 959 CrossRef.
- Y. Wang, Q. Ye, M. Yu, X. Zhang and C. Deng, Chin. Chem. Lett., 2020, 31, 1843–1846 CrossRef CAS.
- N. Tian, Q. M. Jia, H. Y. Su, Y. F. Zhi, A. Ma, J. Wu and S. Y. Shan, J. Porous Mater., 2016, 23, 1269–1278 CrossRef CAS.
- G. Y. Li, Y. R. Jiang, K. L. Huang, P. Ding and J. Chen, J. Alloys Compd., 2008, 466, 451–456 CrossRef CAS.
- A. Lassoued, B. Dkhil, A. Gadri and S. Ammar, Results Phys., 2017, 7, 3007–3015 CrossRef.
|
This journal is © The Royal Society of Chemistry 2023 |