DOI:
10.1039/D2RA07661F
(Paper)
RSC Adv., 2023,
13, 8736-8742
Electro-oxidation reconstitution of aluminium copper MOF-derived metal oxyhydroxides for a robust OER process
Received
1st December 2022
, Accepted 30th January 2023
First published on 15th March 2023
Abstract
It is common knowledge that the O2 evolution reaction (OER) is a crucial half-reaction in the electrolysis of water. However, it is currently difficult to create inexpensive OER electrode materials in a way that is efficient, simple, and environmentally friendly. In this research, metal oxy-hydroxides with numerous oxygen defects (M-OOHv) are created at surface of Cu foam (CF) using a unique, straightforward electro-oxidation reconstitution (ER) process. Different spectroscopic and microscopy methods are used to analyse the electrode characteristics of Al2Cu-MOF@M-OOHv-ER/CF; electrochemical measurements display a lower overpotential (η) of 366 mV @ 10 mA cm−2 and a Tafel slope of 95.2 mV dec−1 in 1.0 M KOH. X-Ray diffraction (XRD), scanning electron microscopy (SEM), and Raman studies confirm the phase transition of the metal–organic framework (MOF) to the M-OOH, which acts as the active site to boost the OER activity. Through spectroscopic and microscopic investigations, it is determined that the efficiency of bimetallic electrode materials and oxygen vacancies in the M-OOHv have an impact on the electron power density. The manufactured electrode material additionally showed good durability for 50 hours. As a result, the newly developed Al2Cu-MOF@M-OOHv-ER/CF nanomaterial has greater potential for both electrolysis of water and other energy storage equipment.
1 Introduction
The requirement for clean, nondepletable energy reservoirs is a hot topic because of the current energy crisis. Functional materials for distinctly efficient hydrogen evolution reactions (HER) and oxygen evolution reactions (OER) attract considerable attention.1–6 A highly active electrode material for the OER is urgently required because the OER has a sluggish kinetics as compared to the HER. Due to excellent oxygen evolution electrocatalytic performance, contemporary IrO2 and RuO2, etc. are valuable metals for electrocatalysts. However IrO2 and RuO2 are not frequently employed in actual commercial applications because of their small reserves, high cost, and unstable nature.7–11 There is a lot of interest in transition metal-based materials (TMMs) as alternatives to precious metal-based materials because they have enormous reserves and various electronic structures that make them appropriate for binding to intermediates.12–14 Metal oxides (MOs), metal hydroxides (MOHs), and metal oxy-hydroxides (MOOHs) are a few of the compounds that have a major impact on the success of the fundamental O2 evolution process. Directly produced TMMs are insufficient because the OER requires a large surface area and a certain shape to expose more active sites.15–19
Therefore, metal–organic frameworks (MOFs) have regarded as electrocatalysts for OER due to their improved specific surface area as well as periodic structure.20 It is tough to further boost the electrocatalytic performance of MOFs on account of their poor electrical conductivity and catalytic activity.20–22 Calcination at high temperatures can convert MOFs with high catalytic activity to the metal oxides. In addition, the presence of a carbon layer can improve the electrical conductivity.23–25 While high-temperature calcination can increase the conductivity and introduce more catalytically active sites, it is also very energy-intensive, waste-producing, and impure.26–28 This makes it necessary to find out an effective, uncomplicated, and ecologically friendly technique to create electrocatalysts that perform wonderfully, which is still an obstacle. However, it has been shown that M-OOHs exhibit outstanding OER electrocatalytic efficacy and developing the corresponding M-OOHv from MOFs with high specific surface area will considerably enhance OER efficiency.29,30 Furthermore, scientists studied the bimetallic MOF, such as Ling et al., who synthesized the Fe0.67Co0.33OOH on nickel foam for OER activity. The electrocatalytic result of Fe0.67Co0.33OOH displays 45.3 mV dec−1 and a Tafel slope of 244 mV @ 10 mA cm−2.31 Frang et al. developed the defect rich (Co, Fe)3O4 for the OER process. Current density at 10 mA cm−2 alongside Tafel slope around 41 mV dec−1, the electrochemical performance related to (Co, Fe)3O4 indicated a smaller overpotential of 286 mV. Due to the enhanced electrochemical result, the enrichment of active centers and defective species occurred.32
In the present study, an Al2Cu-MOF@M-OOHv-ER/CF nanomaterial is developed by an electro-oxidation reconstitution procedure from an Al/Cu bimetallic organic framework (Al2CuMOF) on copper foam, resulting in a metal hydroxide with copious oxygen vacancies (M-OOHv) on the interface of Al2Cu-MOF/CF. The substrate, which is copper foam, has a high porosity and improves the conductivity in addition to exposing a lot of active centers. Additionally, the efficacy of O2 evolution is greatly enhanced when oxygen defect engineering is used in conjunction with the M-OOH. Due to the formation of defects in the crystal structure, the way the electrons are organized can change. This improves the conductivity and accelerates the adsorption of water molecules. With a morphology similar to saw-toothed edges, Al2Cu-MOF@M-OOHv-ER/NF manifests huge electrochemically active surface area (ECSA), a BET surface area of 173 m2 g−1, and stable long-term operation without decay for 50 hours at 1.77 V. Its overpotential is just 366 mV measured @ 10 mA cm−1. The MOF to M-OOHv phase change seen by in situ Raman indicates oxygen defects. In addition, many analytical methods were used to quantify the structural modifications that prove oxygen defects in the MOOHv and the bimetal synergistic effect, which regulate the electron density of states, and are responsible for the enhanced OER catalytic activity. Furthermore, the newly developed Al2Cu-MOF@M-OOHv-ER/CF nanomaterial has vast application capability for complete water splitting and can be produced in an effective, straightforward, and environmentally friendly way and has significant oxygen evolution performance.
2 Experimental
2.1. Chemicals and reagents
Aluminum nitrate (Al(NO3)3·9H2O, ≥98%), copper(II) nitrate (Cu(NO3)2·3H2O, 99%), terephthalic acid (C8H6O4, H2BDC), N,N-dimethylformamide (C3H7NO, DMF), absolute ethanol (C2H6O, 95.0%), and kalium hydroxide (KOH, ≥85%), all were obtained from Sigma-Aldrich. There was no need to purify the aforementioned chemical reagents because they were all of analytical grade.
2.2. Synthesis of Al2Cu-MOF/CF
First, to get rid of any surface impurities, the CF (1 cm × 1 cm) was ultrasonically treated for 10 min in 1.0 M HCl, CH3CH2OH, and DI water. The precursors used for the fabrication of Al2Cu-MOF/CF were Cu(NO3)2·3H2O (160 mg, 0.5 mmol), terephthalic acid (265 mg, 1.65 mmol), and Al(NO3)3·9H2O (280 mg, 1.1 mmol). All these precursors were then disintegrated in mixture of 50 mL DMF, 5 mL of CH3CH2OH, along with 5 mL of DI H2O. Consequently, this uniform mixture was then shifted to a 100 mL hydrothermal reactor along with the already cleaned CF and then placed in the electric furnace for six hours at 160 °C. The autoclave was then allowed to cool at ambient temperature. Then the fabricated Al2Cu-MOF/CF was repeatedly washed with absolute ethanol and double distilled H2O to eliminate any remaining organic ligands after the hydrothermal process. At 60 °C for 6 hours, the newly synthesized bimetallic Al2Cu-MOF/CF was then dried, and stored for further characterization.
2.3. Synthesis of Al2Cu-MOF@M-OOHv-ER/CF
For the fabrication of Al2Cu-MOF@M-OOHv-ER/CF, the electrochemical method was used. For this purpose, the synthesized Al2Cu-MOF/CF was employed as the working electrode, while a platinum wire served as the counter electrode as well as Ag/AgCl served as standard reference electrode. The M-OOHv (oxygen defect metal hydroxide) was electrochemically deposited on the surface of Al2Cu-MOF using 10 CV cycles in the potential window (0 to 2 V) vs. Ag/AgCl inside 1 M aqueous solution of KOH at a sweeping speed of 5 mVs−1. The deposited electrocatalyst (Al2Cu-MOF@M-OOHv-ER/CF) was desiccated in electric oven at 60 °C.
2.4. Material characterization
The crystalline phases of different electrodes were investigated using an XRD (Advance D8 Bruker) with Cu Kα radiation and an accelerating potential of 40 kV in the 2θ range of 10–80°. Raman spectroscopy was used to analyze the material's phase change after it was manufactured (Alpha 300 R) under a He–Ne laser (excitation at 632 nm). The electrode surface morphologies were explored using SEM, and the elemental compositions of the electrodes were investigated using SEM-EDX (Quanta 200-FEG). FT-IR was recorded using a JASCO 6800 FTIR spectrometer with scans ranging from 4000 to 400 cm−1. A Quantachrome Nova2200e BET surface area analyzer was utilized at 80 K to evaluate the specific surface areas.
2.5. Electrochemical measurement
The electrode material that was created acted as the functioning electrode, the counter electrode was made of a Pt wire and standard electrode was made of silver/silver chloride. This was all done in a Pyrex electrolytic cell with 1 M KOH as the electrolyte. An alkaline environment is generally utilized to analyze the O2 evolution process because the O2 evolution reaction includes the creation of defects after the OH− loses electrons. Using the Nernst equation, the potential values in this reading were translated into the RHE with the following eqn (1):33 |
ERHE = EAg/AgCl + 0.059 pH + E0Ag/AgCl
| (1) |
In the non-faradaic region in range of −0.2 V alongside 0.01 V (vs. RHE), electrode ECSAs were measured by cyclic voltammetry (CV) in a sweeping range of 10–50 mV s−1 (vs. RHE). The double-layer capacitance (Cdl) and ECSA were measured using the given expressions (2) and (3):34,35
|
 | (2) |
|
 | (3) |
Herein,
Cdl,
Csp and ECSA are the double layer capacitance (mF), specific capacitance of the flat electrodes (NF, 0.04 mF cm
−2) and electrochemical active surface area (cm
2), respectively. By making a graph of the overpotential (η)
vs. the current density, then Tafel slope was found. This Tafel slope gave information about the kinetic mechanism of the OER for the manufactured materials. Linear sweep voltammetry (LSV) with a 95%
iR correction was used to get the OER polarization curves. The overpotential of the OER at 25 °C was computed with the given expression (3):
36
With the use of the EIS approach, the OER mechanism has been further examined at frequencies ranging between 100 Hz and 100 kHz and an alternating current (AC) potential voltage around 5 mV at the open circuit voltage. The durability of the fabricated material in long-term was measured with chronoamperometry at the cell constant of 0.75 V.
3 Results and discussion
3.1. Structural, morphological, and textural characteristics
To investigate the physical features of the catalyst, the XRD patterns of Al2Cu-MOF/CF and Al2Cu-MOF@M-OOHv-ER/CF were explored in the 2θ range of 10 to 80°. The distinctive diffraction peaks detected in Al2Cu-MOF/CF at 25.85°, 43.07°, and 53.16° correspond to the 002, 100, and 105 indexed planes, and other peaks located at 15.56° and 27.56° correspond to the 110 and 444 indexed planes, which were well consistent with the Al and Cu MOF as previously reported.37,38 The existence of a bimetallic peak in Al2Cu MOF indicates the successful fabrication of Al2Cu MOF. Fig. 1 illustrates that the slight shifting in the 2θ value and variation in the peak intensity suggested the change in MOF phase to the metal hydroxy phase during the electro-oxidation reconstitution (ER). The absence of peaks at 60.29° and 62.82° in Al2Co-MOF@MOOHv-ER/CF also confirms the phase transformation. Furthermore, phase transition and structure of the fabricated material were assessed with Raman spectroscopy as shown in Fig. 1(b). The presence of the characteristics peaks at 714.24, 925.42, 1181.21, 1452.23, and 1630.75 cm−1 is attributed to Al2Cu-MOF/CF. The material displays the characteristic peak of MOOH at 608 cm−1.47 The variation in peak intensity at 608 cm−1 and the production of MOOHv on the interface of Al2Cu-MOF confirm the phase transition and oxygen defects that are present in the Al2Cu MOF@M-OOHv-ER/CF.
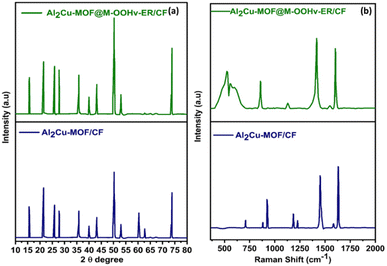 |
| Fig. 1 (a) XRD diffractogram and (b) Raman spectroscopy of Al2CuMOF/CF and Al2Cu-MOF@M-OOHv-ER/CF. | |
Additionally, the molecular structural changes of Al2Cu-MOF/CF and Al2CuMOF@M-OOHv-ER/NF were determined with FT-IR as seen in Fig. 2(a). The stretching bands in Al2Cu-MOF/NF and Al2CuMOF@M-OOHv-ER/NF at 1610 cm−1 and 1390 cm−1 are due to vibrations that concerns carboxyl group from the ligand H2BDC.39,40 Additionally, the benzene ring's C–H bonding stretching from H2BDC appeared at 780 cm−1, and the other peaks located below 700 cm−1 are ascribed due to metal oxide (M–O) vibration modes in Al2CuMOF/CF and Al2Cu-MOF@M-OOHv-ER/CF.41 The presence of FT-IR peaks demonstrates that Al2CuMOF/CF, along with H2BDC, was successfully developed.
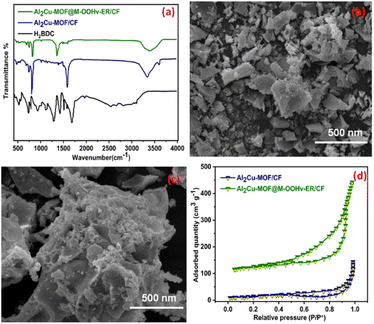 |
| Fig. 2 (a) FTIR, (b and c) SEM micrograph and (d) textural properties of Al2Cu-MOF/CF and Al2Cu-MOF@M-OOHv-ER/CF. | |
Scanning electron microscopy (SEM) micrographs of Al2CuMOF/CF and Al2Cu-MOF@M-OOHv-ER/CF are displayed at 500 nm in Fig. 2(b and c). The Al2CuMOF/CF, which is composed of two-dimensional smooth irregular nanosheets, develops uniformly over the CF. Fig. 2(c) demonstrates that electro-oxidation reconstitution of the material results in a more abrasive and rougher Al2Cu MOF surface, exposing more active edge sites for improving catalytic activity.41,42 In addition, a rough surface implies the production of new compounds, which might reduce the effectiveness of catalysis. Fig. 2(d) illustrates the textural properties of the fabricated material measured under a nitrogen atmosphere at a comparative pressure (P/P0) of 0 to 1.0. The BET isotherm displays the surface areas of Al2CuMOF/CF and Al2Cu-MOF@M-OOHv-ER/CF to be 68 and 173 m2 g−1, respectively.44–46 The BET result suggests that the high surface area of the bimetallic MOF increases the electrocatalytic efficiency of the material toward the OER activity.
3.2. Electrocatalytic OER performance
All OER activities regarding Al2Cu-MOF/CF and Al2Cu-MOF@M-OOHv-ER/CF were investigated in 1.0 M aqueous solution (KOH) configured with a three electrode system whose potential ranging from 0 to 2.0 V. Fig. 3(a) depicts about CV polarization curve of Al2Cu-MOF@M-OOHv-ER/CF exhibited a higher current density than those of Al2Cu-MOF/CF, RuO2 and Cu foam.
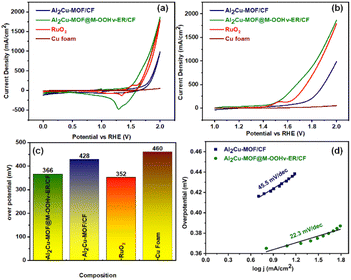 |
| Fig. 3 (a) Cyclic voltammogram at various scanning rates, (b) LS voltammetry, (c) overpotential of Al2Cu-MOF/CF, Al2Cu MOF@M-OOHv-ER/CF, RuO2 and Cu foam @ 10 mA cm−2 and (d) Tafel slope of Al2Cu-MOF/CF and Al2Cu-MOF@M-OOHv-ER/CF@ 10 mA cm−2. | |
Electro-oxidation reconstitution (Fig. 3(b)) showed the higher current density than Al2Cu-MOF/CF, RuO2 and Cu foam. Additionally, the Al2Cu-MOF@M-OOHv-ER/CF nanostructured arrangements with a saw-tooth morphology may assist the uniformly dispersed M-OOHv in attaining the adsorption of OH ions at surface of the electrocatalyst that intensifies conductance of the material and exhibited OER's potent electrochemical performance.43 LSV curves were used to calculate the Tafel slopes for each electrode, so that the whole electrode's OER reaction dynamics could be investigated more closely. Furthermore, the Al2Cu-MOF@M-OOHv-ER/CF (366 mV) delivers a better overpotential than Al2Cu-MOF/CF (428 mV), RuO2 (352 mV) and Cu foam (460 mV) as shown in Fig. 3(c). This may be the result of the M-OOHv adsorption at surface of Al2Cu-MOF/CF.44 For the kinetic mechanism the Tafel slope of Al2Cu-MOF@M-OOHv-ER/CF had the lowest value (22.3 mV dec−1) and delivered the fastest OER reaction kinetics than the other fabricated electrode as demonstrated in Fig. 3(d).
OER competence may also be investigated by calculating the electrochemical active surface area (ECSA) via using value of double layer capacitance (Cdl) with the help of eqn (2) and (3). Al2Cu-MOF@M-OOHv-ER/CF exhibited the most exposed dynamic areas compared to the other fabricated electrode. The ECSA can be calculated using cyclic voltammograms at various scan rates as represented in Fig. 4(a and c). From these curves the Cdl values were calculated by plotting a graph between the change in current density and scan rate. All obtained Cdl results of Al2Cu-MOF/CF and Al2Cu-MOF@M-OOHv-ER/CF were 7.5 mF and 30.2 mF, respectively, as shown in Fig. 4(b and d). The ECSA results for Al2Cu-MOF/CF and Al2Cu-MOF@M-OOHv-ER/CF are 187.5 and 755.0 cm2, respectively.
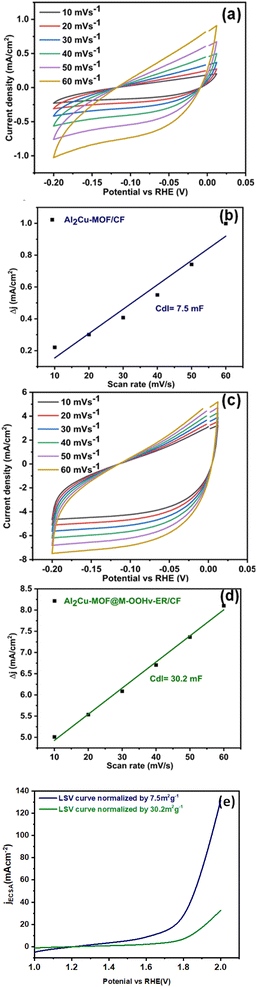 |
| Fig. 4 (a and c) Cyclic voltammogram, (b and d) Cdl plot profile, and (e) normalized plot profile of Al2Cu-MOF/CF and Al2Cu-MOF@M-OOHv-ER/CF. | |
It is probable that the produced saw-tooth type of morphology of Al2Cu-MOF@M-OOHv-ER/CF will exhibit the greatest ECSAs that will boost its OER electrochemical performance.45
For intrinsic activity, the ECSA normalized LSV curve is another way to evidence the remarkable OER activity of all prepared samples. The ECSA-normalized LSV curve is shown in Fig. 4(e). The obtained LSV curve for prepared samples has been normalized by ESCA 7.5 m2 g−1 and 30.2 m2 g−1 for Al2Cu-MOF/CF and Al2Cu-MOF@M-OOHv-ER/CF. The given graph exhibits a higher current density normalized by ECSA in the case of Al2Cu-MOF@M-OOHv-ER/CF as compared to Al2Cu-MOF/CF; therefore, the prepared OER electrocatalyst can be used for commercial and business applications.
To find out more about the OER kinetics, EIS of the fabricated electrode was conducted. The EIS was evaluated in 1.0 M KOH and its frequency ranges through 100 Hz to 100 KHz. Utilizing the Nyquist curves, we were able to determine the resistance values of all electrocatalysts. Compared to the Al2Cu-MOF/CF (14 Ω), the Al2Cu-MOF@M-OOHv-ER/CF electrode depicts extraordinarily low Rct value of (5.7 Ω), which corresponds to the higher oxygen evolution efficiency and lower Tafel slope results. As a consequence, the Al2Cu-MOF@M-OOHv-ER/CF exhibits strong catalytic activity, and good electron transfer capacity.46–50
In addition to electrocatalytic properties, the practical application of Al2Cu-MOF@M-OOHv-ER/CF was determined through chronoamperometry. The Al2Cu-MOF@M-OOHv-ER/CF operates for 50 h without experiencing considerable attenuation and has better stability than RuO2 (Fig. 5(b)).
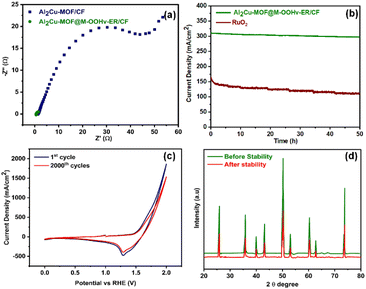 |
| Fig. 5 (a) EIS of Al2Cu-MOF/CF and Al2Cu MOF@M-OOHv-ER/CF, (b) chronoamperometry of Al2Cu MOF@M-OOHv-ER/CF and RuO2, (c) XRD stability and (d) CV stability cycles of Al2Cu-MOF@M-OOHv-ER/CF. | |
The structural durability of the Al2Cu-MOF@M-OOHv-ER/CF was also determined with XRD analysis. The XRD structure stability result suggests that the electrocatalyst is stable over 50 hours, as shown in Fig. 5(c). Additionally, there was no discernible change between the CV curves before and after the durability test, demonstrating exceptional endurance of Al2Cu MOF@M-OOHv-ER/CF, as shown in Fig. 5(c).
4 Conclusions
In conclusion, a novel technique has been created to transform Al2Cu-MOF/CF into Al2Cu-MOF@M-OOHv-ER/CF via electro-oxidation reconstitution that has many oxygen defects on the surface. When analysed with a three-electrode system under 1.0 M aqueous solution, and a current density of around 10 mA cm−2 Al2Cu-MOF@M-OOHv-ER/CF showed lesser overpotential around 224 mV. The Al2Cu-MOF@M-OOHv-ER/CF also displays minimal Tafel slope of 95.2 mV dec−1 having larger ECSA with a Cdl of 30.2 mF. In situ Raman spectroscopy discloses active sites for the OER when the MOF phase transforms into the MOOHv phase. Various analytical techniques of the structure determination helped in betterment of catalytic activity were responsible for the bimetal synergistic effect & oxygen defects in MOOHv, which affects electron density of states. The newly developed Al2Cu-MOF@M-OOHv-ER/CF, which resembles a rough surface, can be produced using a quick, easy, and environmentally friendly process and has remarkable potential for O2 evolution. The in situ electrooxidation reconstitution process proposed in this project offers a unique way to transform MOF materials into derivatives that are catalytically active.
Author contributions
All authors have equal contribution.
Conflicts of interest
There are no conflicts to declare.
Acknowledgements
The authors express their gratitude to Princess Nourah bint Abdulrahman University Researchers Supporting Project (Grant No. PNURSP2023R70), Princess Nourah bint Abdulrahman University, Riyadh, Saudi Arabia.
References
- M. Hassan, Y. Slimani, M. A. Gondal, M. J. Mohamed, S. Güner, M. A. Almessiere and A. Trukhanov, Ceram. Int., 2022, 48, 24866–24876 CrossRef CAS.
- D. A. Vinnik, V. V. Kokovkin, V. V. Volchek, V. E. Zhivulin, P. A. Abramov, N. A. Cherkasova, Z. Sun, M. I. Sayyed, D. I. Tishkevich and A. V. Trukhanov, Mater. Chem. Phys., 2021, 270, 124818 CrossRef CAS.
- A. V. Trukhanov, V. O. Turchenko, I. A. Bobrikov, S. V. Trukhanov, I. S. Kazakevich and A. M. Balagurov, J. Magn. Magn. Mater., 2015, 393, 253–259 CrossRef CAS.
- M. Zdorovets, A. Kozlovskiy, D. Tishkevich, T. Zubar and A. Trukhanov, J. Mater. Sci.: Mater. Electron., 2020, 31, 21142–21153 CrossRef CAS.
- S. Manzoor, M. Sadaqat, S. J. H. hah, S. Gouadria, F. Hussain, K. S. Joya and M. N. Ashiq, Fuel, 2022, 319, 123797 CrossRef CAS.
- X. Xu, R. Wang, S. Chen, A. Trukhanov, Y. Wu, L. Huang and Z. Sun, Inorg. Chem. Front., 2022, 9, 5507–5516 RSC.
- S. Cao and J. Yu, Chem. Lett., 2014, 5, 2101–2107 CAS.
- O. Polat and N. Sahiner, Int. J. Energy Res., 2022, 46, 14587–14608 CrossRef CAS.
- S. J. Mun and S. J. Park, Catalysts, 2019, 9(10), 805 CrossRef CAS.
- M. S. Reza, N. B. H. Ahmad, S. Afroze, J. Taweekun, M. Sharifpur and A. K. Azad, Chem. Eng. Technol., 2022, 46(3), 420–434 CrossRef.
- A. Naseri, A. M. Samadi, A. Pourjavadi, A. Z. Moshfegh and S. Ramakrishna, J. Mater. Chem. A, 2017, 5, 23406–23433 RSC.
- Z. Chen, L. Guo, L. Pan, T. Yan, Z. He, Y. Li, C. Shi, Z. Huang, X. Zhang and J. Zou, Adv. Energy Mater., 2022, 12, 2103670 CrossRef CAS.
- Y. Gao, D. Kong, F. Cao, S. Teng, T. Liang, B. Luo, B. Wang, Q.-H. Yang and L. Zhi, Nano Res., 2022, 15, 7959–7967 CrossRef CAS.
- N. Prabu, R. S. A. Saravanan, T. Kesavan, G. Maduraiveeran and M. Sasidhara, Carbon, 2019, 152, 188–197 CrossRef CAS.
- Y. Zhu, T. R. Kuo, Y. H. Li, M. Y. Qi, G. Chen, J. Wang, Y. J. Xu and H. M. Chen, Energy Environ. Sci., 2021, 14, 1928–1958 RSC.
- A. V. Trukhanov, V. G. Kostishyn, L. V. Panina, V. V. Korovushkin, V. A. Turchenko, P. Thakur, A. Thakur, Y. Yang, D. A. Vinnik, E. S. Yakovenko, L. Y. Matzui, E. L. Trukhanova and S. V. Trukhanov, J. Alloys Compd., 2018, 754, 247–256 CrossRef CAS.
- S. V. Trukhanov, A. V. Trukhanov, V. A. Turchenko, E. L. Trukhanova, D. I. Tishkevich, V. M. Ivanov, T. I. Zubar, M. Salem, V. G. Kostishyn, L. V. Panina, D. A. Vinnik and S. A. Gudkova, Ceram. Int., 2018, 44, 290–300 CrossRef CAS.
- M. A. Almessiere, A. V. Trukhanov, Y. Slimani, K. Y. You, S. V. Trukhanov, E. L. Trukhanova, F. Esa, A. Sadaqati, K. Chaudhary, M. Zdorovets and A. Baykal, Nanomaterials, 2019, 9, 202, DOI:10.3390/nano9020202.
- J. Zhu, B. Zeng, L. Mo, F. Jin, M. Deng and Q. Zhang, Appl. Clay Sci., 2021, 206, 106070 CrossRef CAS.
- S. Fu, C. Zhu, J. Song, D. Du and Y. Lin, Adv. Energy Mater., 2017, 7, 1700363 CrossRef.
- Q. Qian, Y. Li, Y. Liu, L. Yu and G. Zhang, Adv. Mater., 2019, 31, 1901139 CrossRef PubMed.
- Y. Yang, Y. Yang, Y. Liu, S. Zhao and Z. Tang, Small Sci., 2021, 1, 2100015 CrossRef CAS.
- N. Cheng, L. Ren, X. Xu, Y. Du and S. X. Dou, Adv. Energy Mater., 2018, 8, 1801257 CrossRef.
- D. Wang, D. Y. Jana and Y. Zhao, Acc. Chem. Res., 2020, 53, 1389–1400 CrossRef CAS PubMed.
- A. Indra, T. Song and U. Paik, Adv. Mater., 2018, 30, 1705146 CrossRef PubMed.
- T. Montini, M. Melchionna, M. P. Monai and P. Fornasiero, Chem. Rev., 2016, 116, 5987–6041 CrossRef CAS PubMed.
- Y. Li, Z. Y. Fu and B. L. Su, Adv. Funct. Mater., 2012, 22, 4634–4667 CrossRef CAS.
- A. Trovarelli, Catal. Rev. - Sci. Eng., 1996, 38, 439–520 CrossRef CAS.
- Z. Gao, Z. W. Yu, F. Q. Liu, C. Yang, Y. H. Yuan and Yu F. Luo, ChemSusChem, 2019, 12, 4623–4628 CrossRef CAS PubMed.
- S. Niu, C. Li, J. Huo, W. Dong, S. El Hankari, Y. Liang and Q. Li, ACS Omega, 2021, 6, 13946–13952 CrossRef CAS PubMed.
- X. Ling, F. Du, Y. Zhang, Y. Shen, W. Gao, B. Zhou, Z. Wang, G. Li, T. Li, Q. Shen, Y. Xiong, X. Wang, Y. Zhou and Z. Zou, J. Mater. Chem. A, 2021, 9, 13271–13278 RSC.
- F. Liu, W. Jin, Y. Li, L. Zheng, Y. Hu, X. Xu, Y. Xue, C. Tang, H. Liu and J. Zhang, Appl. Surf. Sci., 2020, 529, 147125 CrossRef CAS.
- D. Chu and J. Li, ECS Trans., 2020, 97, 575–580 CrossRef CAS.
- L. Zhang, H. Jang, H. Liu, M. G. Kim, D. Yang, X. Liu, S. Liu and J. Cho, Angew. Chem., Int. Ed., 2021, 60, 18821–18829 CrossRef CAS PubMed.
- W. Cheng, Z. P. Wu, D. Luan, S. Q. Zang and X. W. Lou, Angew. Chem., Int. Ed., 2021, 60, 26397–26402 CrossRef CAS PubMed.
- J. Seong Kim, I. Park, E.-S. Jeong, K. Jin, W. Mo Seong, G. Yoon, H. Kim, B. Kim, K. Tae Nam, K. Kang, J. S. Kim, I. Park, E. Jeong, K. Jin, W. M. Seong, G. Yoon, H. Kim, B. Kim, K. T. Nam and K. Kang, Adv. Mater., 2017, 29, 1606893 CrossRef PubMed.
- R. Nivetha, A. Sajeev, A. Mary Paul, K. Gothandapani, S. Gnanasekar, G. Jacob, R. Sellappan, V. Raghavan, N. Krishna Chandar, S. Pitchaimuthu, S. K. Jeong and A. Nirmala Grace, Mater. Res. Express, 2020, 7, 114001 CrossRef CAS.
- X. Zeng, J. Wenwu, H. Li, I. Saikumar and Q. Zhang, Nanotechnology, 2022, 31, 1–22 Search PubMed.
- H. Ren, T. Y. Song, J. N. Xu, S. B. Jing, Y. Yu, P. Zhang and L. R. Zhang, Cryst. Growth Des., 2009, 9, 105–112 CrossRef CAS.
- M. M. Jia, X. Y. Zhang, Q. L. Yang, D. Q. Xiong, P. K. Fu, M. M. Jiao, X. L. Wang and X. Y. Dong, J. Coord. Chem., 2021, 74, 2641–2656 CrossRef CAS.
- H. P. Nguyen Thi, H. D. Ninh, C. Van Tran, B. T. Le, S. V. Bhosale and D. D. La, ChemistrySelect, 2019, 4, 2333–2338 CrossRef CAS.
- C. H. Choi, S. H. Park and S. I. Woo, ACS Nano, 2012, 6, 7084–7091 CrossRef CAS PubMed.
- Y. Zhang, J. Wang, L. Ye, M. Zhang and Y. Gong, Dalton Trans., 2021, 50, 4720–4726 RSC.
- M. Vandichel, K. Laasonen and I. Kondov, Top. Catal., 2020, 63, 833–845 CrossRef CAS.
- Y. Chen, Z. Yu, R. Jiang, J. Huang, Y. Hou, J. Chen, Y. Zhang, H. Zhu, B. Wang and M. Wang, Small, 2021, 17, 2101003 CrossRef CAS PubMed.
- H. He, Y. Wang, J. Li, S. Jiang, S. Sidra, W. Gong, Y. Tang, Y. Hu, R. Wei, D. Yang, X. Li and Z. Zhao, Chem. Eng. J., 2022, 427, 131962 CrossRef CAS.
- N. Ma, J. Xu, Z. Bian, Y. Yang, L. Zhang and H. Wang, Chem. Eng. J., 2020, 389, 123426 CrossRef CAS.
- T. I. Zubar, V. M. Fedosyuk, A. V. Trukhanov, N. N. Kovaleva, K. A. Astapovich, D. A. Vinnik, E. L. Trukhanova, A. L. Kozlovskiy, M. V. Zdorovets, A. A. Solobai, D. I. Tishkevich and S. V. Trukhanov, J. Electrochem. Soc., 2019, 166, D173–D180 CrossRef CAS.
- T. Zubar, V. Fedosyuk, D. Tishkevich, O. Kanafyev, K. Astapovich, A. Kozlovskiy, M. Zdorovets, D. Vinnik, S. Gudkova, E. Kaniukov, A. S. B. Sombra, D. Zhou, R. B. Jotania, C. Singh, S. Trukhanov and A. Trukhanov, Nanomaterials, 2020, 10, 1–14 CrossRef PubMed.
- M. Chen, G. C. Wang, W. Q. Yang, Z. Y. Yuan, X. Qian, J. Q. Xu, Z. Y. Huang and A. X. Ding, ACS Appl. Mater. Interfaces, 2019, 11, 42156–42171 CrossRef CAS PubMed.
|
This journal is © The Royal Society of Chemistry 2023 |
Click here to see how this site uses Cookies. View our privacy policy here.