DOI:
10.1039/D2RA07374A
(Paper)
RSC Adv., 2023,
13, 2561-2569
A novel supramolecular Zn(II)-metallogel: an efficient microelectronic semiconducting device application†
Received
20th November 2022
, Accepted 10th January 2023
First published on 17th January 2023
Abstract
A unique strategy for the synthesis of a supramolecular metallogel employing zinc ions and adipic acid in DMF medium has been established at room temperature. Rheological analysis was used to investigate the mechanical characteristics of the supramolecular Zn(II)-metallogel. Field emission scanning electron microscopy and transmission electron microscopy were used to analyse the hexagonal shape morphological features of the Zn(II)-metallogel. Interestingly, the electrical conductivity is observed in the electronic device with Zn(II)-metallogel based metal–semiconductor (MS) junctions. All aspects of the metallogel's electrical properties were investigated. The electrical conductivity of the metallogel-based thin film device was 7.38 × 10−5 S m−1. The synthesised Zn(II)-metallogel based device was investigated for its semi-conductive properties, such as its Schottky barrier diode nature.
1. Introduction
The study of supramolecular gels is one of the most exciting and promising areas of research in the field of supramolecular chemistry and materials science. Gels are solids with a high degree of viscoelasticity; they are made up of an elastic as well as cross-linked network and a solvent. The liquid is entrapped and adheres to the vast surface area of the three-dimensional solid matrix, giving the gel a solid-like appearance.1,2 Several non-covalent interactions such as hydrophobic contacts, van der Waals forces, cation⋯π, anion⋯π, π⋯π, hydrogen bonding, dipole–dipole, ion–ion, and dipole–dipole interactions etc. have been crucial to the generation of supramolecular networks.3,4 The direct use of supramolecular phenomena has facilitated the development of 3D soft gel scaffolds.5 To create a stable gel architecture with different gelators, a wide variety of polar type solvents, like water,6 methanol,7 ethanol,8 dimethyl sulfoxide,9 acetonitrile,10 N,N-dimethyl formamide,11 and nonpolar solvents, such as tetrahydrofuran,12 toluene,13 acetone,14 carbon tetrachloride,15 1,2 dichlorobenzene,16 dichloromethane,17 deuterated dichloromethane18 solvents are very effective.
As supramolecular type soft scaffolds, low-molecular-weight gelators (LMWGs) with a molecular weight of ≤3000 have been introduced into synthetic gel systems along with the polymeric gelator-based gel. Despite various low molecular weight gelators such as alkenes,19 saccharides,20 sugars,21 urea derivatives,22 modified amino acids,23 peptides,24 bile acids,25 carbohydrate,26 cholesterol,27 and amides28 are general in the chemistry of gelation, achieving the necessary and effective synthesis utilising LMWGs remains still difficult. Along with the demonstration of the supramolecular gelation process, the current direction in gel research29 is the introduction of metal ions along with the LMWGs for the formation of multifunctional supramolecular metallogels. The combination of diverse metal ions and organic components of low molecular weight combine to generate metallogels with distinct self-aggregation mechanisms and noncovalent characteristics, which lead to the development of more compelling and remarkable properties in the domain of science and technology. Supramolecular metallogels have significant applications in a wide range fields of materials science, including the food industry, cosmetics, electron emission, photophysics, logic gates, drug delivery, cell culturing, biomineralization, medical diagnostics, tissue engineering, lithography, optical activity, energy storage, charge transportation, catalysis, conductivity, actuators, magnetic materials, redox responsiveness, chemosensors, electrochemical and optoelectronic devices, nanoscience and nanoelectronics etc.30–49
Recent emphasis has been focused on supramolecular metallogel systems based on transition metals as smart functional materials owing to their affordability and availability. Multiple transition metal ion-based metallogels involving Cu, Ni, Fe, Mn, Co, etc. are documented in the scientific literature.50–55 Zn(II) is one of the most important transition metal ions for numerous successful applications in catalysis,56 cell imaging,57 and medicinal chemistry58 etc.
Functional LMWG based supramolecular gels may be synthesised using the concept that adipic acid has metallogel forming capacity in DMF solvent media. We have used a method called adipic acid-mediated gel formation in this endeavour. Here, we investigate the susceptibility of adipic acid to metallogelation using Zn(II) as a source in N,N-dimethylformamide under ambient conditions. A new supramolecular Zn(II)-metallogel (Zn-AA) is facilitated by the presence of adipic acid. Adipic acid may look promising as a LMWG towards a wide variety of metal ions since the adipate ion may serve as a chelating ligand as well as a bridging ligand.
Researchers in the field of materials science are always exploring for opportunities to create innovative forms of energy storage and conversion technology. The discovery of the functional material mediated by metallogels is a major advance in this field. The mechanical flexibility and soft chemical nature of metallogels opens up a wide range of possible applications.59
Herein, we have tested the feasibility of using Zn-AA metallogel in devices, since in the semiconducting area, this material provides a considerable optical band gap. Implementing these materials in real-world electrical devices is a technical challenge. We have successfully used Zn(II) metallogel material in the metal–semiconductor junction thin film devices to investigate the capability for transporting charge and adaptability of electronic device. Schottky behaviour with potential photosensing activity using readily accessible, low-cost transition metal ions has been shown in a synthetic material-based metal semiconductor (MS) junction device. Our constructed devices show a non-linear rectifying nature in their current–voltage characteristics graph, much like a Schottky barrier diode with a significant rectification ratio. As a result, we have evaluated the functionality of the Schottky diode we built using Zn-AA metallogel. Research into the material's photophysical and electronic charge transport properties demonstrates its potential to pique the interest of future scientists developing metallogel-based active electronic devices.
2. Experimental
2.1. Materials
Adipic acid and zinc(II) acetate dihydrate were bought from Sigma-Aldrich Company and they were utilised exactly as supplied. The whole experiment was executed using N,N-dimethyl formamide (DMF) solvent.
2.2. Characterizations
A SHIMADZU UV-3101PC spectrophotometer was utilized for the UV-vis absorption spectrum data.
Rheological studies: for rheology experiment of the gels, an Anton Paar 100 rheometer with a cone and plate geometry (CP 25-2) having an adjustable Peltier temperature controlling system was used. All the measurements were done fixing the gap distance between the cone and the plate at 0.05 mm. The gels were scooped on the plate of the rheometer. An oscillatory strain amplitude sweep experiment was performed at a constant oscillation frequency of 1 Hz for the applied strain range 0.001–10% at 20 °C. The software US-200 converted the torque measurements into either G′ (the storage modulus) and G′′ (the loss modulus) and represent G′ and G′′ with either strain or shear stress. Oscillatory frequency sweep experiments were performed in the linear viscoelastic region (strain 0.01%) to ensure that calculated parameters correspond to an intact network structures.
A Carl Zeiss SUPRA 55VP FESEM instrument was utilized to perform the field emission scanning electron microscope (FESEM).
Transmission electron microscopy (TEM) was performed using a ThermoFisher image corrected Titan Themis at an operating voltage of 300 kV. A Bruker SuperX detector was used for the energy-dispersive X-ray spectroscopy (EDX) study in scanning TEM mode (STEM). TEM sample was prepared by sonication in ethanol.
For the IR investigation, a Shimadzu FTIR-8400S FTIR spectrometer was used.
The Phillips PANalytical X'PERT PRO equipment was utilized to acquire the Powder X-Ray Diffraction (PXRD) data of the Zn-AA metallogel at room temperature.
A digital melting point measuring apparatus Aplab (model: MPA-01) was used to determine the Tgel of the Zn-AA metallogel.
Our synthesised metallogel material-based thin film device's current–voltage (I–V) characteristics were performed using a Keithley 2401 source meter.
2.3. Synthesis of Zn(II)-metallogel (Zn-AA)
At room temperature, 0.219 g of Zinc(II) acetate dihydrate (1 mmol) in ∼1 mL of DMF and 0.292 g of adipic acid (2 mmol) in ∼1 mL of DMF were combined. Therefore, a white, stable gel of Zn(II) (i.e. Zn-AA) was formed by sonicating the vial for 5 minutes at ambient temperature and air pressure (Fig. 1).
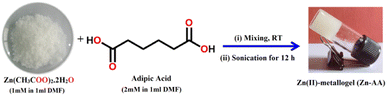 |
| Fig. 1 Representation of the gelation process of Zn(II)-metallogel. | |
3. Results and discussion
3.1. Evaluation of the gel-melting temperature (Tgel) and the minimum critical gelation concentration (MGC)
The assessment of the Zn-AA metallogel's minimum critical gelation concentration (MGC) is shown in Fig. 2. To assess the MGC of the Zn-AA metallogel, the concentrations of Zn(CH3COO)2·2H2O and adipic acid were altered within a range of concentration (i.e. 30 mg mL−1 to 512 mg mL−1). Here, [Zn(CH3COO)2·2H2O]
:
[adipic acid] = 1
:
2, (w/w), was retained as the constituent ratio for the Zn-AA metallogel. 512 mg mL−1 of Zn(II)-acetate salt and adipic acid in DMF solvent produced the stable and white Zn-AA metallogel (Fig. 2).
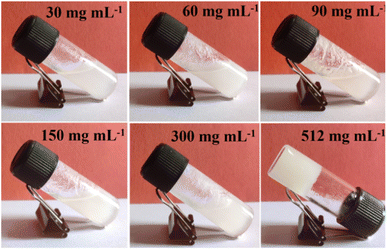 |
| Fig. 2 Photographic illustration of the Zn-AA metallogel gelating at the minimal critical concentration (MGC). MGC is 512 mg mL−1 when compared to the 1 : 2 (w/w) weight ratio of Zn(CH3COO)2·2H2O and adipic acid. | |
The gel melting temperature (Tgel) of Zn-AA metallogel was recorded as ∼120 °C ± 2 °C by digital melting point measuring apparatus.
3.2. Rheological analysis
Rheological measurements were used to determine the semi-solid and viscoelastic nature of Zn-AA metallogel (Fig. 3). The storage modulus and the loss modulus of the metallogel are denoted by G′ and G′′ respectively. They are represented by the equations
G′ = (σ0/γ0) cos(δ) |
G′′ = (σ0/γ0) sin(δ) |
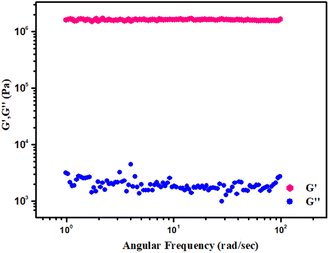 |
| Fig. 3 Variation of G′ and G′′ (Pa) of Zn-AA metallogel. | |
For any gel material the condition G′(ω) > G′′(ω) is valid [where, G′(ω) ≈ ω° and ω represents the angular frequency].
Keeping the Zn(OAc)2·2H2O concentration constant (i.e. [Zn(II)] = 512 mg mL−1), rheological studies demonstrated that the value of storage modulus of Zn-AA metallogel is much higher than the value of loss modulus, i.e. (G′ > G′′) (Fig. 3). The Zn-AA metallogel is found to have a significantly high storage modulus (G′ > 106 Pa) that is much greater than its loss modulus (G′′), which is reconcilable with the material maintaining its gel structural characteristic, semisolid-like behaviour, and large tolerance limit (Fig. 3). Fig. 4 displays the results of a strain-sweep measurement of Zn-AA metallogel at a fixed 6.283 rad s−1 frequency.
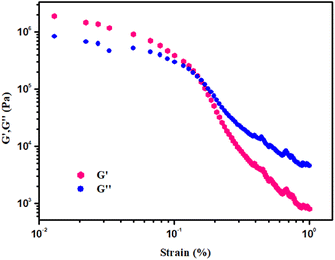 |
| Fig. 4 Strain-sweep measurements of Zn-AA metallogel. | |
3.3 Microstructural study
The field emission scanning electron microscopic (FESEM) pattern of the Zn-AA metallogel showed the agglomerated hexagonal like hierarchical network of the metallogel (Fig. 5a and b).
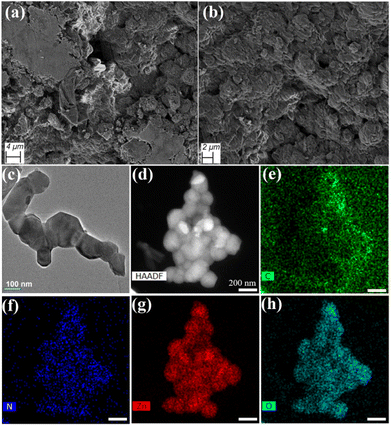 |
| Fig. 5 Zn-AA metallogel: (a and b) the FESEM microstructural pattern of Zn-AA metallogel, (c) bright field TEM image of Zn-AA metallogel. (e–h) Elemental composition of C, N, Zn and O are mapped by STEM EDX. The corresponding region is shown in STEM image (d). | |
The structural concreteness in microstructural network of Zn-AA metallogel might be due to the predominant supramolecular interactions. Bright field TEM image reveals a mixed hexagonal and pentagonal shaped structural morphology of Zn-AA metallogel in Fig. 5c. The average range in length and width is measured around 200–400 nm and 120–320 nm for hexagonal and 160–220 nm and 200 nm in pentagonal structure, respectively. Along with hexagonal morphology, a rod shape feature is found in minor quantity. Please see Fig. S1 in ESI† for the rod shape morphological pattern along with its chemical composition mapping by STEM EDX. Elemental composition mapping by STEM EDX in Fig. 5d–h confirms presence of Zn, N, C and O elements of Zn(OAc)2·2H2O, adipic acid and DMF molecules, which are used in preparation of Zn-AA metallogel (Fig. 5d).
3.4 FT-IR and PXRD analysis of Zn-AA metallogel
The Fourier transform infrared (FT-IR) spectra of Zn-AA metallogel in its xerogel form discloses the major absorption peaks are located at 2950, 2875, 1690, 1530, 1446, 1401, 1341, 1273, 1221, 1045, 906, 740, 690 and 520 cm−1 etc. (Fig. 6). The high intensity peak at 1690 cm−1 is obtained because of the asymmetric stretching vibration of carbonyl group (C
O) of acetate. The O–H stretching and the C–H stretching at 2950 cm−1 and 2875 cm−1, are both excellent indicators of the existence of adipic acid. Fig. 6 displays the FT-IR spectra of Zn-AA metallogel. The (O–C
O) vibration mode and C
O symmetric stretching are attributed to the peak at 1530 cm−1. The C–H bond vibration and C–O stretching modes are responsible for the numerous absorption peaks seen at 1446 cm−1, 1401 cm−1, 1341 cm−1, 1273 cm−1 and 1221 cm−1. The C–C stretching modes of vibration are responsible for a sequence of FTIR bands at 906 cm−1 and 1045 cm−1. The band at 740 cm−1, confirms the OH out of plane bending. The absorption frequency at 690 cm−1 is associated with the COO bending mode. The spectrum depicts a peak at 520 cm−1 that is obtained because of the presence of Zn–O bond and it confirms the formation of the metallogel. Fourier transform infrared (FT-IR) spectral data displays the supramolecular interactions in Zn-AA metallogel among the metallogel forming chemical components.
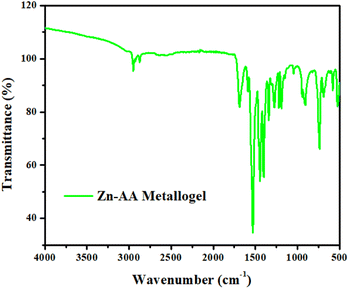 |
| Fig. 6 FT-IR spectra of the xerogel form of Zn-AA metallogel. | |
The powder X-ray diffraction is utilized to find out the structural nature of Zn-AA metallogel (Fig. 7). The crystalline character of the Zn(II)-metallogel is indicated by the PXRD pattern of sharp and narrow peaks. As shown in Fig. 7, the sharp XRD peaks appearing at 2θ = 25.2, 27.0, 29.1°, 39.0°, 49.0°, and 60.2° confirms the crystalline nature of Zn-AA metallogel gel. Further the XRD patter is compared with the standard data (JCPDS card # 372718) of zinc dicarboxylate, and is indexed accordingly. All these results confirm the crystalline nature of Zn-AA metallogel gel due to presence of zinc dicarboxylate.
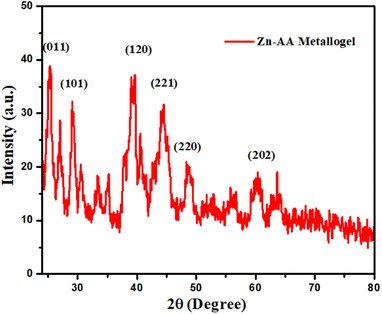 |
| Fig. 7 Powder X-ray diffraction (PXRD) pattern of Zn-AA metallogel in xerogel form. | |
3.5 Device fabrication
To make metallogel-based thin film devices (MBDs), glass substrates with indium-doped tin oxide (ITO) coatings were subjected to standard wet cleaning techniques utilising an ultrasonic cleaner in a soap solution, deionized water followed by isopropanol and acetone for 15 minutes each, before finally being annealed in 70 °C in the presence of N2 for 15 minutes. For performing the electrical properties measurement multiple metal–semiconductor (MS) junction devices having the configuration like sandwich i.e. ITO/Zn-AA metallogel/Au were developed. The thin film of as-synthesized compound was pasted on the pre cleaned ITO-coated glass substrate by doctor blading method. Here the gold (Au) was used as the metal electrode, which was deposited on a precleaned plain glass slide by using a thermal evaporator unit under the base pressure of 10−6 Torr.
Electrical parameters were analysed by measuring the device's current–voltage (I–V) characteristics using a Keithley Sourcemeter (model no. 2401) and the two-probe method. All of the devices were built and tested under ambient circumstances at room temperature (28 °C).
3.6 Optical characterization
The optical band gap (Eg) of our Zn-AA metallogel was determined by using Tauc's equation (eqn (1)).60where ν, h, Eg and α stand for frequency of light, Planck's constant, optical band gap and absorption coefficient respectively. The exponent ‘n’ is the constant which depends on the process of electron transition. For ideal case the value of ‘A’ is considered as 1. The direct optical band gap has been computed using the aforementioned equation with an appropriate exponent value n = ½.60 The values of optical direct band gap (Eg) have been computed as 3.52 eV for our synthesized Zn-AA metallogel by extrapolating the straight line portion of (αhν)2 vs. hν graph (Fig. A) to α = 0 absorption.
The analysis of the optical properties of Zn-AA metallogel was executed using UV-Vis spectroscopy. From the obtained UV-Vis absorption spectra (inset of Fig. 8), the band gap (optical) (Eg) of the metallogel was determined. From Tauc's equation (eqn (1)),60 the Eg was computed as 3.52 eV (Fig. 8). The obtained spectrum determined that the band gap of compound (1) is a direct type.
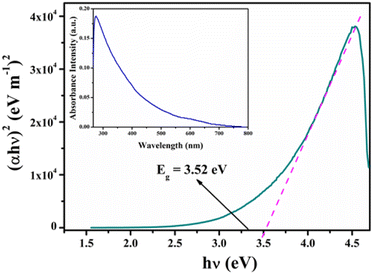 |
| Fig. 8 Optical direct band gap (Eg) and UV-Vis absorption spectra (inset) of the metallogel. | |
3.7 Electrical characterization
The just computed band gap value suggested our synthesized metallogel from the semiconductor family. Therefore, to study its electrical properties, Metal (Al)–Semiconductor (metallogel) (MS) junction thin-film devices were fabricated followed by the procedure described in device fabrication part. The current–voltage (I–V) graph of metallogel-based devices (MBDs) was performed with bias voltage applied in the span of ±2 V at room temperature (28 °C).
Fig. 9 shows the I–V graph of our fabricated MBDs from where the conductivity (σ) was computed as 7.38 × 10−5 S m−1, which signifies our Zn-AA metallogel as a typical semiconductor. The I–V graph of MBD (Fig. 9) represents nonlinear rectifying behavior which depicts the development of the Schottky barrier diode at the Al/metallogel boundary. So, in this article, we showed our attention to studying the properties of transporting charge of MBD and further discussed them elaborately. The on/off (rectification) ratio of our device (Ion/Ioff) at ±2 V was calculated from the I–V graph (Fig. 9) as 49.63. The obtained I–V graph of MBD was analyzed in detail utilizing thermionic emission theory. Hence, Cheung's method was implemented to compute some important parameters of diode.60–63
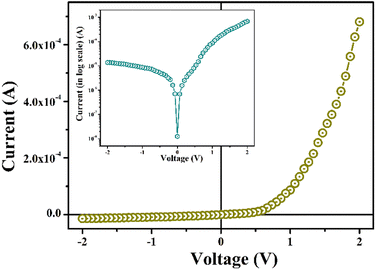 |
| Fig. 9 I–V characteristics graph of MBD. | |
By using thermionic emission theory, we were able to get a deeper understanding of the I–V characteristic of metallogel based device (MBD). Important diode parameters were also extracted using Cheung's method.60 Accordingly, we performed a quantitative analysis of the acquired I–V curve using the following reference eqn (2) and (3):61,62
|
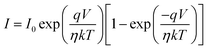 | (2) |
|
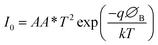 | (3) |
where,
I0 stands for saturation current,
A* is effective Richardson constant,
η is ideality factor,
A denotes effective diode area,
V represents forward bias voltage,
T is the absolute temperature in Kelvin,
q is the electronic charge and
k is the Boltzmann constant. The functional Richardson constant was taken into account for all the devices as 32 A K
−2 cm
−2 and the active diode area was determined to be 7.065 × 10
−2 cm
2.
60
Eqn (4)–(6), derived from Cheung's concept, were also used to calculate the series resistance (RS), ideality factor (η) and potential barrier height (∅B).62,63
|
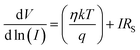 | (4) |
|
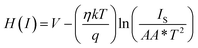 | (5) |
The ideality factor (η) for MBD was calculated as 2.16, from the intercept of the dV/d
ln
I vs. I graph (Fig. 10). From the slope of the same plotting, the series resistance (RS) of MBD was also calculated as 3.79 KΩ. A fluctuation from the ideal value (∼1) for the computed η is shown for MBD. This deviation symbolizes the barrier height inhomogeneities with the presence of juncture states and series resistance at the MS junction.64,65
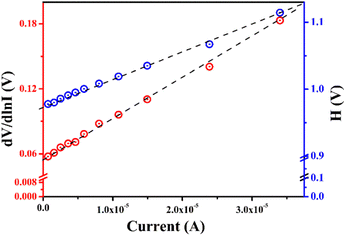 |
| Fig. 10 dV/d ln I vs. I and H vs. I graph of MBD. | |
The barrier height (∅B) of MBD was estimated as 0.45 eV from the intercept of H vs. current graph (Fig. 10) and utilizing the just computed η [eqn (6)]. The RS of MBD was also computed from the slope of this graph and it was calculated as 4.01 KΩ. Table 1 presents all the values of the computed parameters of MBD. The obtained values of RS from the different two graphs are very near about.
Table 1 Various parameters of Schottky device (Zn-AA based)
Device |
On/off |
Conductivity (S m−1) |
Ideality factor (η) |
Barrier height (∅B) (eV) |
Series resistance (RS) |
From dV/d ln I vs. I (KΩ) |
From H vs. I (KΩ) |
Zn-AA metallogel based device |
49.63 |
7.38 × 10−5 |
2.16 |
0.45 |
3.79 |
4.01 |
For an in-depth explanation of the moving of the charge carriers at the MS boundary, we plotted the I–V curves on a logarithmic scale. In the ln(I) vs. ln(V) graph of MBD, two distinctive slopes are seen (Fig. 11). These two distinctive slope regions were represented as region-I and region-II. In region-I, the value of the slope is ∼1, indicating the ohmic regime. In this region, the generated current follows the I ∝ V relation. On the other hand, the value of slope in region-II is ≥ 2 and the generated current follows the I ∝ V2 relation (Fig. 11). Hence region-II is contemplated as a space charge limited current (SCLC) region that is devoid of trap.60,66 If the inherent charge transporters are less than the inserted charge transporters, a space charge field is produced due to the expanding of the inserted charge transporters. This generated charge field governs the produced currents so it is called space charge limited current or SCLC.60,66 To estimate the performance of MBD, in this article we employed this theory. Hence to compute the effective carrier mobility from region-II, the equation of Mott–Gurney was considered and I vs. V2 graph (Fig. 12) has been drawn:60,63,66
|
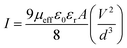 | (7) |
where,
ε0,
εr,
μeff and
I symbolize their usual meaning. The relative dielectric constants value of the metallogel was considered as 2.25.
67
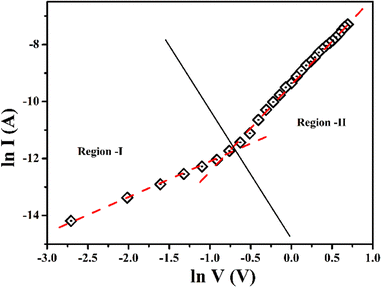 |
| Fig. 11 ln I vs. ln V graph of MBD. | |
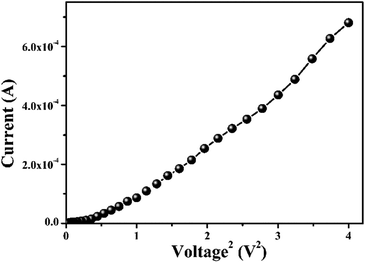 |
| Fig. 12 I vs. V2 graph of MBD. | |
The diffusion length (LD) and transit time (τ) were also computed to study the transportation phenomenon of the charge carrier across the junction. The transit time (τ) value has been computed from eqn (8).60
|
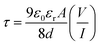 | (8) |
|
 | (9) |
|
 | (10) |
Here,
D represents the diffusion coefficient and was computed using the Einstein–Smoluchowski equation (
eqn (9)).
60 LD of charge carriers acts a crucial role in device performance when an MS junction is developed and was computed from the
eqn (10). All the calculated parameters are shown in
Table 2.
Table 2 Various charge conducting parameters of MBD
Device |
εr |
μeff (m2 V−1 s−1) |
τ (sec) |
μeffτ (m2 V−1) |
D (m2 s−1) |
LD (m) |
Zn-AA metallogel based device |
2.25 |
1.08 × 10−6 |
3.21 × 10−9 |
3.46 × 10−13 |
2.78 × 10−8 |
1.34 × 10−7 |
We have compared the parameters of our fabricated semiconductor device with other reported various semiconductor devices and presented in Table 3.
Table 3 Comparison table of electrical parameters of Zn-AA metallogel based device with other reported results
Device based on |
On/off |
Ideality factor |
Barrier height (eV) |
Series resistance (KΩ) |
Ref. |
[Zn(INH)(succ)]n |
176 |
1.43 |
0.36 |
— |
68 |
ZnCdS |
29 |
2.22 |
— |
17.3 |
69 |
[Cd(4bpd)(SCN)2]n |
46.55 |
5.52 |
0.36 |
— |
70 |
C40H34Cu2N6O18 |
8.46 |
2.78 |
0.47 |
— |
71 |
[Cd4L2(NCO)6]n |
12.44 |
3.47 |
0.526 |
5.31 |
72 |
Zn-AA metallogel |
49.63 |
2.16 |
0.45 |
3.79 |
Present work |
From the Table 3 it is clear that the ideal factor of our fabricated semiconductor device is more ideal (∼1) than the other reported semiconductor devices. Moreover, the barrier height of our device is less than 0.5 eV, which means this device can work under very small applied voltage. Even, the series resistance is lower for this device compared to other devices. This proves that the performance of our fabricated device is better than the other devices.
4. Conclusions
In conclusion, rapid mixing of zinc acetate and a low molecular weight gelator adipic acid in DMF solvent medium followed by sonication yielded a new supramolecular Zn(II)-metallogel at room temperature. Zn-AA metallogel is created by a variety of non-covalent interactions that allow it to be stable at ambient conditions. Zn-AA metallogel's hierarchical structure, which resembles flakes, was investigated using the field emission scanning electron microscopy (FESEM) as well as the transmission electron microscopy (TEM) instruments. The rheological tests have analysed the mechanical strength of the Zn-AA metallogel. The measurements of optical band gap of our generated Zn-AA metallogel are consistent with a semiconducting state. Also, we used semiconducting Zn-AA metallogel and Au metal to create a metal–semiconductor junction thin film device. Fabrication of a Schottky diode was verified by the current–voltage (I–V) characteristic graph, which demonstrated the device's nonlinear charge transport. Therefore, the current investigation of a sandwich-like configuration of the indium tin oxide (ITO), zinc acetate-adipic acid (Zn-AA) metallogel, and gold (Au) hints to the potential for developing supramolecular Zn2+ metallogel-based electrical devices for cutting-edge technology in the future. In actuality, the adipic acid and zinc(II) source based Zn-AA metallogel study is a pioneering strategy and symbolise metallogel for the fabrication of the semiconducting device.
Conflicts of interest
The authors declare no competing financial interests.
Acknowledgements
K. K. is grateful to the University Grant Commission, New Delhi, for awarding him Junior Research Fellowship (Award letter number: 63/(CSIR-UGC NET June 2019)). S. D. is thankful to the UGC, New Delhi, for awarding him Dr D. S. Kothari Postdoctoral Fellowship (Award letter number: No. F.4-2/2006 (BSR)/CH/19-20/0224). S. B. thankfully acknowledges DST Inspire Faculty Research Grant (Faculty Registration No.: IFA18-CH304; DST/INSPIRE/04/2018/000329).
Notes and references
- N. M. Sangeetha and U. Maitra, Chem. Soc. Rev., 2005, 34, 821–836 RSC.
- P. Dastidar, Chem. Soc. Rev., 2008, 37, 2699–2715 RSC.
- M. D. Ward, Chem. Soc. Rev., 1997, 26, 365–375 RSC.
- R. Madueno, M. T. Räisänen, C. Silien and M. Buck, Nature, 2008, 454, 618–621 CrossRef CAS PubMed.
- P. Terech and R. G. Weiss, Chem. Rev., 1997, 97, 3133–3159 CrossRef CAS PubMed.
- S. Dhibar, A. Dey, A. Dey, S. Majumdar, A. Mandal, P. P. Ray and B. Dey, New J. Chem., 2019, 43, 15691–15699 RSC.
- C. K. Karan and M. Bhattacharjee, ACS Appl. Mater. Interfaces, 2016, 8, 5526–5535 CrossRef CAS PubMed.
- Q. Lin, Q. P. Yang, B. Sun, Y. P. Fu, X. Zhu, T. B. Weia and Y. M. Zhang, Soft Matter, 2014, 10, 8427–8432 RSC.
- X. Ma, S. Liu, Z. Zhang, Y. Niu and J. Wu, Soft Matter, 2017, 13, 8882–8885 RSC.
- C. Po, Z. Ke, A. Y. Y. Tam, H. F. Chow and V. W. W. Yam, Chem.–Eur. J., 2013, 19, 15735–15744 CrossRef CAS PubMed.
- S. Dhibar, A. Dey, D. Ghosh, S. Majumdar, A. Dey, P. Mukherjee, A. Mandal, P. P. Ray and B. Dey, ChemistrySelect, 2019, 4, 1535–1541 CrossRef CAS.
- R. M. Ortuño, Gels, 2021, 7, 54 CrossRef PubMed.
- C. A. Offiler, C. D. Jones and J. W. Steed, Chem. Commun., 2017, 53, 2024–2027 RSC.
- B. Jiang, J. Zhang, W. Zheng, L. J. Chen, G. Q. Yin, Y. X. Wang, B. Sun, X. Li and H. B. Yang, Chem.–Eur. J., 2016, 22, 14664–14671 CrossRef CAS PubMed.
- H. Bunzen, Nonappa, E. Kalenius, S. Hietala and E. Kolehmainen, Chem.–Eur. J., 2013, 19, 12978–12981 CrossRef CAS PubMed.
- K. Mitsumoto, J. M. Cameron, R. J. Wei, H. Nishikawa, T. Shiga, M. Nihei, G. N. Newton and H. Oshio, Chem.–Eur. J., 2017, 23, 1502–1506 CrossRef CAS PubMed.
- F. Gou, J. Cheng, X. Zhang, G. Shen, X. Zhou and H. Xiang, Eur. J. Inorg. Chem., 2016, 2016, 4862–4866 CrossRef CAS.
- X. Q. Wang, W. Wang, G. Q. Yin, Y. X. Wang, C. W. Zhang, J. M. Shi, Y. Yu and H. B. Yang, Chem. Commun., 2015, 51, 16813–16816 RSC.
- S. J. Wezenberg, C. M. Croisetu, M. C. A. Stuart and B. L. Feringa, Chem. Sci., 2016, 7, 4341–4346 RSC.
- R. Luboradzki and Z. Pakulski, Tetrahedron, 2004, 60, 4613–4616 CrossRef CAS.
- J. H. Jung, G. John, M. Masuda, K. Yoshida, S. Shinkai and T. Shimizu, Langmuir, 2001, 17, 7229–7232 CrossRef CAS.
- M. George, G. Tan, V. T. John and R. G. Weiss, Chem.–Eur. J., 2005, 11, 3243–3254 CrossRef CAS PubMed.
- S. Samai, J. Dey and K. Biradha, Soft Matter, 2011, 7, 2121–2126 RSC.
- C. Tomasini and N. Castellucci, Chem. Soc. Rev., 2013, 42, 156–172 RSC.
- P. Babu, N. M. Sangeetha and U. Maitra, Macromol. Symp., 2006, 241, 60–67 CrossRef CAS.
- C. Narayana, R. K. Upadhyay, R. Chaturvedia and R. Sagar, New J. Chem., 2017, 41, 2261–2267 RSC.
- J. Yan, J. Liu, P. Jing, C. Xu, J. Wu, D. Gao and Y. Fang, Soft Matter, 2012, 8, 11697–11703 RSC.
- C. C. Tsou and S. S. Sun, Org. Lett., 2006, 8, 387–390 CrossRef CAS PubMed.
- Q. Lin, T. T. Lu, X. Zhu, B. Sun, Q. P. Yang, T. B. Weia and Y. M. Zhang, Chem. Commun., 2015, 51, 1635–1638 RSC.
- X. Ji, Y. Yao, J. Li, X. Yan and F. Huang, J. Am. Chem. Soc., 2013, 135, 74–77 CrossRef CAS PubMed.
- S. M. Mehta, T. Jin, I. Stanciulescu and K. J. Grande-Allen, Acta Biomater., 2018, 75, 52–62 CrossRef CAS PubMed.
- B. Escuder, F. Rodríguez-Llansola and J. F. Miravet, New J. Chem., 2010, 34, 1044–1054 RSC.
- X. Cheng, J. Pan, Y. Zhao, M. Liao and H. Peng, Adv. Energy Mater., 2018, 8, 1702184–1702199 CrossRef.
- X.-Q. Dou and C.-L. Feng, Adv. Mater., 2017, 29, 1604062–1604082 CrossRef PubMed.
- N. Shi, G. Yin, M. Han and Z. Xu, Colloids Surf., B, 2008, 66, 84–89 CrossRef CAS PubMed.
- A. R. Hirst, B. Escuder, J. F. Miravet and D. K. Smith, Angew. Chem., Int. Ed., 2008, 47, 8002–8018 CrossRef CAS PubMed.
- J. Wang, Z. Wang, J. Gao, L. Wang, Z. Yang, D. Kong and Z. Yang, J. Mater. Chem., 2009, 19, 7892–7896 RSC.
- J. Boekhoven and S. I. Stupp, Adv. Mater., 2014, 26, 1642–1659 CrossRef CAS PubMed.
- X. Jiaa and K. L. Kiick, Macromol. Biosci., 2009, 9, 140–156 CrossRef PubMed.
- P. R. A. Chivers and D. K. Smith, Nat. Rev. Mater., 2019, 4, 463–478 CrossRef CAS.
- O. Roubeau, A. Colin, V. Schmitt and R. Clérac, Angew. Chem., Int. Ed., 2004, 43, 3283–3286 CrossRef CAS PubMed.
- V. K. Pandey, M. K. Dixit, S. Manneville, C. Bucherc and M. Dubey, J. Mater. Chem. A, 2017, 5, 6211–6218 RSC.
- Y.-X. Ye, W.-L. Liu and B.-H. Ye, Catal. Commun., 2017, 89, 100–105 CrossRef CAS.
- W. Miao, L. Zhang, X. Wang, H. Cao, Q. Jin and M. Liu, Chem.–Eur. J., 2013, 19, 3029–3036 CrossRef CAS PubMed.
- S. Dhibar, A. Dey, S. Majumdar, D. Ghosh, A. Mandal, P. P. Ray and B. Dey, Dalton Trans., 2018, 47, 17412–17420 RSC.
- J. Gómez-Herrero and F. Zamora, Adv. Mater., 2011, 23, 5311–5317 CrossRef PubMed.
- H. Su, S. Zhu, M. Qu, R. Liu, G. Song and H. Zhu, J. Phys. Chem. C, 2019, 123, 15685–15692 CrossRef CAS.
- P. Sutar and T. K. Maji, Chem. Commun., 2016, 52, 8055–8074 RSC.
- S. Dhibar, A. Dey, S. Majumdar, A. Dey, P. P. Ray and B. Dey, Ind. Eng. Chem. Res., 2020, 59, 5466–5473 CrossRef CAS.
- S. Dhibar, A. Dey, A. Dey, S. Majumdar, D. Ghosh, P. P. Ray and B. Dey, ACS Appl. Electron. Mater., 2019, 19, 1899–1908 CrossRef.
- S. Dhibar, R. Jana, P. P. Ray and B. Dey, J. Mol. Liq., 2019, 289, 111126 CrossRef CAS.
- N. Malviya, C. Sonkar, R. Ganguly and S. Mukhopadhyay, Inorg. Chem., 2019, 58, 7324–7334 CrossRef CAS PubMed.
- S. Dey, D. Datta, K. Chakraborty, S. Nandi, A. Anoop and T. Pathak, RSC Adv., 2013, 3, 9163–9166 RSC.
- S. Saha, E.-M. Schön, C. Cativiela, D. D. Díaz and R. Banerjee, Chem.–Eur. J., 2013, 19, 9562–9568 CrossRef CAS PubMed.
- S. Dhibar, S. K. Ojha, A. Mohan, S. P. C. Prabhakaran, S. Bhattacharjee, K. Karmakar, P. Karmakar, P. Predeep, A. K. Ojha and B. Saha, New J. Chem., 2022, 46, 17189–17200 RSC.
- S. Enthaler, ACS Catal., 2013, 3, 150–158 CrossRef CAS.
- A. Biswas, S. Mukhopadhyay, R. S. Singh, A. Kumar, N. K. Rana, B. Koch and D. S. Pandey, ACS Omega, 2018, 3, 5417–5425 CrossRef CAS PubMed.
- R. Leuci, L. Brunetti, A. Laghezza, F. Loiodice, P. Tortorella and L. Piemontese, Appl. Sci., 2020, 10, 4118 CrossRef CAS.
- H. Wu, J. Zheng, A. L. Kjøniksen, W. Wang, Y. Zhang and J. Ma, Adv. Mater., 2019, 31, 1806204 CrossRef PubMed.
- A. Dey, S. Middya, R. Jana, M. Das, J. Datta, A. Layek and P. P. Ray, J. Mater. Sci.: Mater. Electron., 2016, 27, 6325–6335 CrossRef CAS.
- E. H. Rhoderick, Metal Semiconductors Contacts, Oxford University Press, Oxford, 1978 Search PubMed.
- S. K. Cheung and N. W. Cheung, Appl. Phys. Lett., 1986, 49, 85–87 CrossRef CAS.
- A. Dey, A. Layek, A. Roychowdhury, M. Das, J. Datta, S. Middya, D. Das and P. P. Ray, RSC Adv., 2015, 5, 36560–36567 RSC.
- R. K. Gupta and F. Yakuphanoglu, Sol. Energy, 2012, 86, 1539–1545 CrossRef CAS.
- X. Miao, S. Tongay, M. K. Petterson, K. Berke, A. G. Rinzler, B. R. Appleton and A. F. Hebard, Nano Lett., 2012, 12, 2745–2750 CrossRef CAS PubMed.
- P. W. M. Blom, M. J. M. de Jong and M. G. van Munster, Phys. Rev. B: Condens. Matter Mater. Phys., 1997, 55, R656–R659 CrossRef CAS.
- S. Dhibar, A. Dey, S. Majumdar, P. P. Ray and B. Dey, Int. J. Energy Res., 2020, 45, 5486–5499 CrossRef.
- K. Naskar, A. Dey, B. Dutta, F. Ahmed, C. Sen, M. H. Mir, P. P. Ray and C. Sinha, Cryst. Growth Des., 2017, 17, 3267–3276 CrossRef CAS.
- M. Das, J. Datta, R. Jana, S. Sil, S. Halder and P. P. Ray, New J. Chem., 2017, 41, 5476–5486 RSC.
- S. Halder, A. Dey, A. Bhattacharjee, J. Ortega-Castro, A. Frontera, P. P. Ray and P. Roy, Dalton Trans., 2017, 46, 11239–11249 RSC.
- A. Hossain, A. Dey, S. K. Seth, P. P. Ray, P. Ballester, R. G. Pritchard, J. Ortega-Castro, A. Frontera and S. Mukhopadhyay, ACS Omega, 2018, 3, 9160–9171 CrossRef CAS PubMed.
- P. Ghorai, A. Dey, P. Brandão, J. Ortega-Castro, A. Bauza, A. Frontera, P. P. Ray and A. Saha, Dalton Trans., 2017, 46, 13531–13543 RSC.
Footnotes |
† Electronic supplementary information (ESI) available: TEM image of Zn-AA metallogel. See DOI: https://doi.org/10.1039/d2ra07374a |
‡ K. K. and A. D. should be treated as joint first authors. |
|
This journal is © The Royal Society of Chemistry 2023 |
Click here to see how this site uses Cookies. View our privacy policy here.