DOI:
10.1039/D2RA07319F
(Paper)
RSC Adv., 2023,
13, 320-334
Sulfamic acid grafted to cross-linked chitosan by dendritic units: a bio-based, highly efficient and heterogeneous organocatalyst for green synthesis of 2,3-dihydroquinazoline derivatives†
Received
17th November 2022
, Accepted 15th December 2022
First published on 21st December 2022
Abstract
In this work, novel cross-linked chitosan by the G1 dendrimer from condensation of melamine and toluene-2,4-diisocyante terminated by sulfamic acid groups (CS-TDI-Me-TDI-NHSO3H), as a bio-based and heterogeneous acidic organocatalyst, was designed and prepared. Also, the structure of the prepared organocatalyst was characterized by Fourier transform infrared spectroscopy (FT-IR), field emission scanning electron microscopy (FESEM), energy-dispersive X-ray spectroscopy (EDS), X-ray diffraction (XRD) and thermogravimetric analysis/derivative thermogravimetry (TGA/DTA). Subsequently, the catalytic performance of the biobased and dendritic CS-TDI-Me-TDI-NHSO3H, as a multifunctional solid acid, was evaluated for the preparation of 2,3-dihydroquinazoline derivatives through a three-component reaction by following green chemistry principles. Some of the advantages of this new protocol include high to excellent yields and short reaction times as well as easy preparation and remarkable catalyst stability of the introduced acidic organocatalyst. The CS-TDI-Me-TDI-SO3H catalyst can be used for up to five cycles for the preparation of quinazoline derivatives with a slight decrease in its catalytic activity.
Introduction
Polysaccharide-based scaffolds have been widely used in different fields such as drug delivery, vaccines, wound dressing materials, cosmetics, food additives and packaging, environmental applications including water treatment, and heterogeneous organocatalysts due to their bioactivity, biodegradability and biocompatibility in recent decades.1–14 Hence, the use of renewable biopolymers such as cellulose, chitin, sodium alginate, and especially chitosan for the design and preparation of efficient biodegradable and heterogeneous organocatalytic systems would be very desirable.11,15–39 Among these scaffolds, chitosan (CS) is one of the most unique and widely used biopolymers, a natural and active cationic amino polysaccharide obtained from the alkaline N-deacetylation of chitin.8,24,40–47 Indeed, chitosan has numerous applications in various fields such as preparation of new bio-based materials,48–52 heterogeneous catalytic systems,38,53–55 water purification, metal extraction,56–59 electrolyte-based fuel cells,60–62 sensors,6,63 corrosion protection,64 etc. However, many research attempts are being made to provide functional chitosan derivatives with chemical modifications. Some of the advantages of using chitosan in various applications include low cost, chemical stability, desirable hydrophilicity, having proper functional groups for chelation of metals, non-toxicity, and environmental friendliness. Compared to homogeneous catalytic systems, heterogeneous ones are much more efficient for multiple and continous use in the chemical synthesis.65–67 Indeed, heterogeneous catalyst systems benefit from easy removal, recovery and recycling of the catalyst compared to homogeneous catalysts.68 Therefore, chitosan application as a new support material for heterogeneous catalysis is increasing.69,70 Also, the use of ligands containing multi-amine groups in a chain or dendrimer increases the catalytic efficiency by increasing the number of active sites.
Melamine-based dendrimer amines (MDAs) are ideal dendrimer ligands, first reported in 2000 by Simanek and Zhang. MDAs have received a great deal of attention due to the strong binding of amine sites and increasing of the surface hydrophilicity.71–75 On the other hand, sulfamic acid (H2NSO3H) is a common sulfur-containing amino acid with mild acidity, which has been used to replace conventional Lewis and Brønsted acid catalysts.76–78 Noteworthy, sulfamic acid also exists as H3N+SO3− zwitterionic units insoluble in non-polar organic solvents. Hence, its catalytic properties arise from its zwitterionic nature and shows excellent activity in acid-catalyzed organic transformations. Thus, it has been widely used, as an acidic catalyst, in reactions such as Michael addition reaction,71 Pechmann reaction,79 Beckmann rearrangement reaction,80 imino Diels–Alder reaction,81 and functional group protection82 and deprotection83 reactions.
2,3-Dihydroquinazolines are a group of heterocyclic compounds that have a pyrimidine nucleus in their structure.84–88 Also, they have received increased attention due to their wide range of biological properties such as anesthetic,89 anti-cancer,90 muscle relaxant91 and sedative properties.92 Therefore, synthesis of 2,3-dihydroquinazoline derivatives has attracted the attention of organic and pharmaceutical chemists, leading to various methods for the preparation of 2,3-dihydroquinazoline derivatives in order to achieve higher reaction efficiency.70,93–105 Most of these reported methods have disadvantages such as multi-stage preparation methods, long reaction time, low efficiency, hard reaction conditions, and the use of precious metals or toxic reagents. Thus, using new methods for the synthesis of 2,3-dihydroquinazolines under desired reaction conditions is important.
In the present work, sulfamic acid grafted to cross-linked chitosan by dendritic units (CS-TDI-Me-TDI-NHSO3H, 1) organocatalyst was designed, prepared and characterized. The CS-TDI-Me-TDI-NHSO3H was used as a heterogeneous and green nanocatalyst for the synthesis of 2,3-dihydroquinazoline derivatives (Scheme 1).
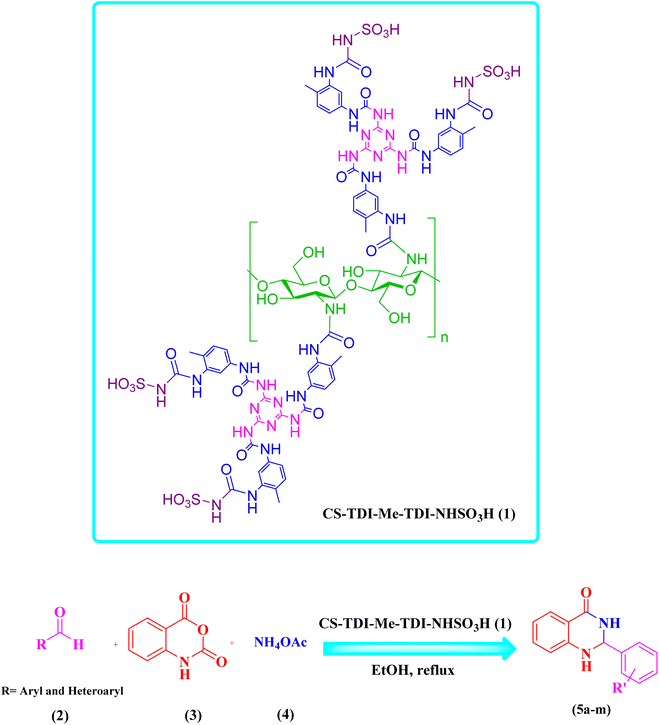 |
| Scheme 1 CS-TDI-Me-TDI-NHSO3H (1), as solid acid catalyst, for green synthesis of 2,3-dihydroquinazoline derivatives by using aldehyde derivatives (2), isatoic anhydride (3) and ammonium acetate (4) in EtOH under reflux conditions. | |
Results and discussion
The prepared CS-TDI-Me-TDI-HNSO3H organocatalyst (1) was characterized using various suitable techniques including FT-IR, FESEM, XRD, TGA and EDS.
Fig. 1 shows the FT-IR spectra of melamine–toluene 2,4-diisocyanate intermediate (I, a) and CS-TDI-Me-TDI-HNSO3H (1, b). According to Fig. 1a, the absorption bands at 3468–3334 cm−1 are attributed to the stretching vibration of N–H bonds of amin groups. Also, absorption band at 2926 cm−1 belongs to the stretching vibration of C–H aliphatic bonds. In addition, the adsorption bands at 2276 cm−1 and 1652 cm−1 correspond to the vibration of N
C
O and C
O bonds of the amide. As shown in Fig. 1b, the absorption bands at 3470–3336 cm−1 are attributed to the stretching vibration of N–H bonds of the amine groups. Also, the absorption band at 2976 cm−1 belongs to the stretching vibration of C–H aliphatic bonds. Whereas, the adsorption band at 1654 cm−1 is related to the stretching vibration of C
O bond of amide groups. Also, the characteristic bands at 1208 cm−1 and 1026 cm−1 correspond to the asymmetric and symmetric S
O stretching vibration in the SO3H group, respectively.
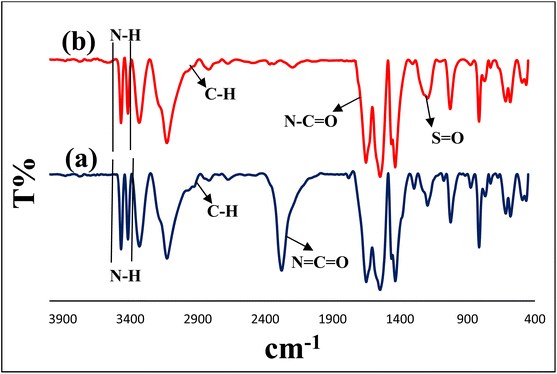 |
| Fig. 1 FTIR spectra of melamine–toluene 2,4-diisocyanate intermediate (I, a) and CS-TDI-Me-TDI-HNSO3H bio-based material (1, b). | |
Fig. 2 shows the XRD pattern of CS-TDI-Me-TDI-HNSO3H (1). There are symmetrical reflections at 2θ of 17.56°, 21.52°, 26.08°, 28.71°, and 29.72° which are characteristic of the CS-TDI-Me-TDI-HNSO3H (1) structure according to the standard XRD patterns of melamine (JCPDS card no. 00-039-1950), chitosan (JCPDS card no. 00-040-1518), and H2NSO3H (JCPDS card no. 01-070-0060). As can be seen, the results obtained from the XRD pattern of CS-TDI-Me-TDI-HNSO3H (1) confirm the successful preparation of the desired nanomaterial.
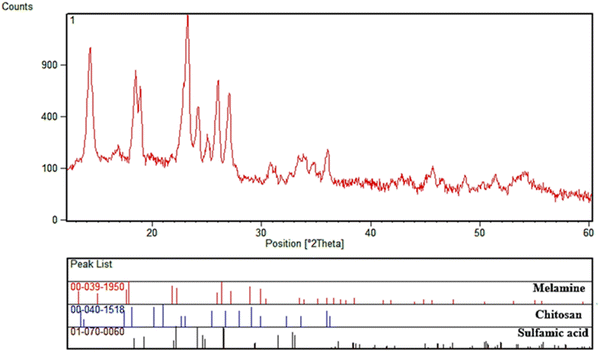 |
| Fig. 2 Wide-angle XRD pattern of CS-TDI-Me-TDI-HNSO3H nanomaterial (1). | |
Fig. 3 shows the EDS analysis related to the CS-TDI-Me-TDI-HNSO3H organocatlyst (1), which confirms the presence of C, O, N, and S elements in its structure. Therefore, the presence of S element indicates the grafting of H2NSO3H on the chitosan backbone. Also, EDS mapping analysis shows uniform particle distribution of the structure.
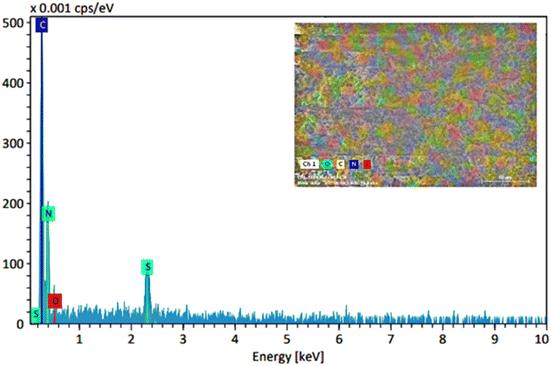 |
| Fig. 3 EDS spectra of the CS-TDI-Me-TDI-HNSO3H organocatalyst (1). | |
FESEM images of CS-TDI-Me-TDI-HNSO3H (1) nanomaterial (1) are shown in Fig. 4. FESEM images of structure of CS-TDI-Me-TDI-HNSO3H shows that the morphology of chitosan has changed from sheets to irregular particles, which confirms the formation of the desired structure. Also, these particles have a uniform dispersion and average particle size of 25–44 nm.
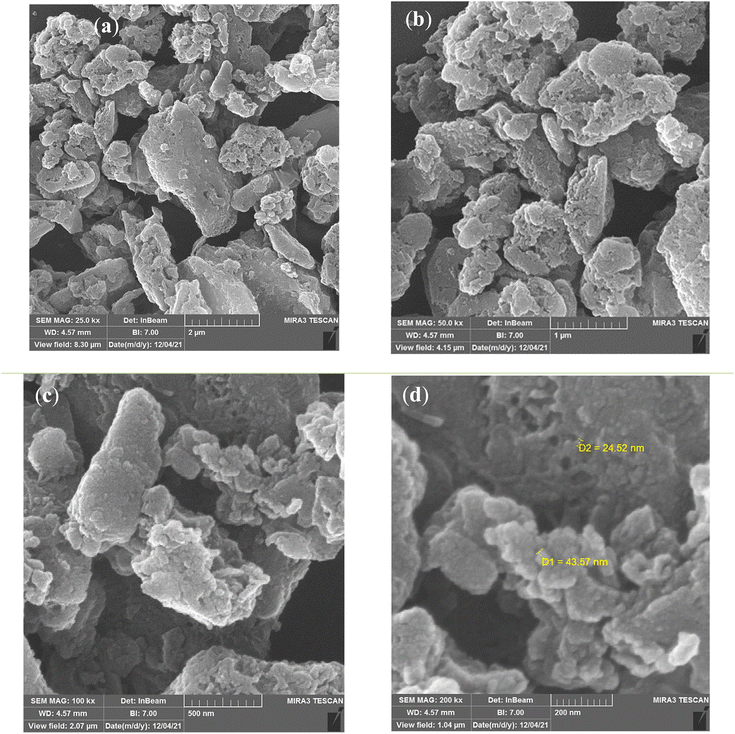 |
| Fig. 4 FESEM images of CS-TDI-Me-TDI-HNSO3H nanomaterial (1). | |
Using thermogravimetric analysis (TGA), the thermal stability of the prepared catalyst (1) was investigated in the temperature range of 50–500 °C. As shown in Fig. 5, two weight loss steps were observed between 270 and 400 °C, Since the pristine chitosan is degraded at 200–220°,106 this degradation at the temperature range of 270–400 °C indicates that the organic units on the surface of chitosan have been linked by toluene diisocyanate, which affects the thermal stability of chitosan and degradation takes place at a higher temperature.
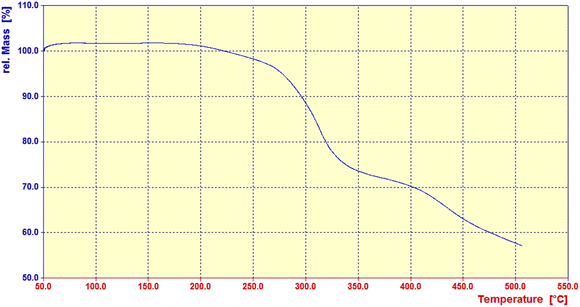 |
| Fig. 5 TGA curves of CS-TDI-Me-TDI-HNSO3H organocatalyst (1). | |
Optimization of conditions for the synthesis of 2,3-dihydroquinazoline derivatives in the presence of CS-TDI-Me-TDI-HNSO3H organocatalyst (1)
In this section, the efficacy of CS-TDI-Me-TDI-HNSO3H nanomaterial (1) in the model reaction for the synthesis of 2, 3-dihydroquinazoline derivatives was investigated. Therefore, different parameters including solvent, catalyst loading, temperature, and reaction time were investigated to determine the optimal reaction conditions (Table 1). The model reaction was investigated in the presence of 4-chloroaldehyde (2a, 0.5 mmol), isatoic anhydride (3, 0.5 mmol) and ammonium acetate (4, 1.5 mmol) for the synthesis of 2,3-dihydroquinazoline derivatives in various conditions. First, the model reaction was run without catalyst using various solvents at different temperatures (Table 1, entries 1–4). As shown in Table 1, without catalyst, the model reaction did not proceed to afford the desired product after 1 h. However, in the presence of 15 mg of CS-TDI-Me-TDI-HNSO3H organocatalyst (1), the desired product 5a was prepared in medium to excellent yields (57 to 97%, Table 1, entries 5–8). The progress of the model reaction to afford the desired product 5a in EtOH was investigated at temperatures rather than reflux conditions (Table 1, entries 9 and 10). Based on the obtained results, EtOH under reflux conditions can be considered as the desirable solvent. Afterward, to determine the desirable amount of catalyst, the model reaction was carried out in EtOH under reflux conditions in the presence of 10, 15 and 20 mg of CS-TDI-Me-TDI-HNSO3H organocatalyst (1) (Table 1, entries 10–12). Consequently, 15 mg of CS-TDI-Me-TDI-HNSO3H organocatalyst (1) loading in EtOH under reflux conditions were selected as the optimal reaction conditions.
Table 1 Screening of optimized conditions for three component reaction of 4-chloroaldehyde (2a), isatoic anhydride (3) and ammonium acetate (4) to afford 2,3-dihydroquinazoline derivative 5a in the presence of CS-TDI-Me-TDI-HNSO3H solid acid (1)a
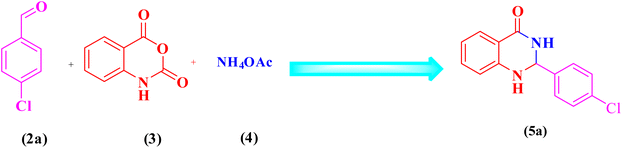
|
Entry |
Catalyst |
Conditions |
Catalyst loading (mg) |
Time (min) |
Yield (%) |
Reaction conditions: 4-chloroaldehyde (2a, 0.5 mmol), isatoic anhydride (3, 0.5 mmol) and ammonium acetate (4, 1.5 mmol) in the presence of CS-TDI-Me-TDI-HNSO3H (1) unless otherwise noted. |
1 |
— |
EtOH/RT |
— |
60 |
Trace |
2 |
— |
CH2Cl2/RT |
— |
60 |
— |
3 |
— |
H2O/RT |
— |
60 |
— |
4 |
— |
DMF/RT |
— |
60 |
Trace |
5 |
CS-TDI-Me-TDI-HNSO3H (1) |
EtOH/reflux |
15 |
15 |
97 |
6 |
CS-TDI-Me-TDI-HNSO3H (1) |
H2O/reflux |
15 |
60 |
57 |
7 |
CS-TDI-Me-TDI-HNSO3H (1) |
CH2Cl2/reflux |
15 |
60 |
40 |
8 |
CS-TDI-Me-TDI-HNSO3H (1) |
DMF/reflux |
15 |
60 |
62 |
9 |
CS-TDI-Me-TDI-HNSO3H (1) |
EtOH/RT |
15 |
40 |
50 |
10 |
CS-TDI-Me-TDI-HNSO3H (1) |
EtOH/50 °C |
15 |
20 |
87 |
11 |
CS-TDI-Me-TDI-HNSO3H (1) |
EtOH/reflux |
10 |
15 |
80 |
12 |
CS-TDI-Me-TDI-HNSO3H (1) |
EtOH/reflux |
20 |
45 |
83 |
After that, in order to extend the catalytic application of CS-TDI-Me-TDI-HNSO3H (1), three-component condensation of aldehyde derivatives (2a–m, 0.5 mmol), isatoic anhydride (3, 0.5 mmol), and ammonium acetate (4, 1.5 mmol) was performed under optimal conditions for the synthesis of 2,3-dihydroquinazoline derivatives (5a–m). The results are summarized in Table 2.
Table 2 Synthesis of 2,3-dihydroquinazoline derivatives (5a–m) through the three-component condensation of aldehyde derivatives (2a–m), isatoic anhydride (3), and ammonium acetate (4) in the presence of CS-TDI-Me-TDI-HNSO3H organocatalyst (1)a
The possible mechanism for the synthesis of 2,3-dihydroquinazoline derivatives in the presence of CS-TDI-Me-TDI-HNSO3H (1)
Scheme 2 shows the proposed mechanism for the synthesis of 2,3-dihydroquinazoline derivatives. CS-TDI-Me-TDI-HNSO3H organocatalyst (1) has Brønsted acidic centers. Hence, it activates carbonyl groups in isotonic anhydride by forming a hydrogen bond to facilitate the nucleophilic addition of ammonium acetate (4) and forming the intermediate I. Next, this intermediate reacts with aldehyde derivatives and forms intermediate II. Finally, by removing H2O from intermediate II, 2,3-dihydroquinazoline derivatives 5 are synthesized as the desired product.
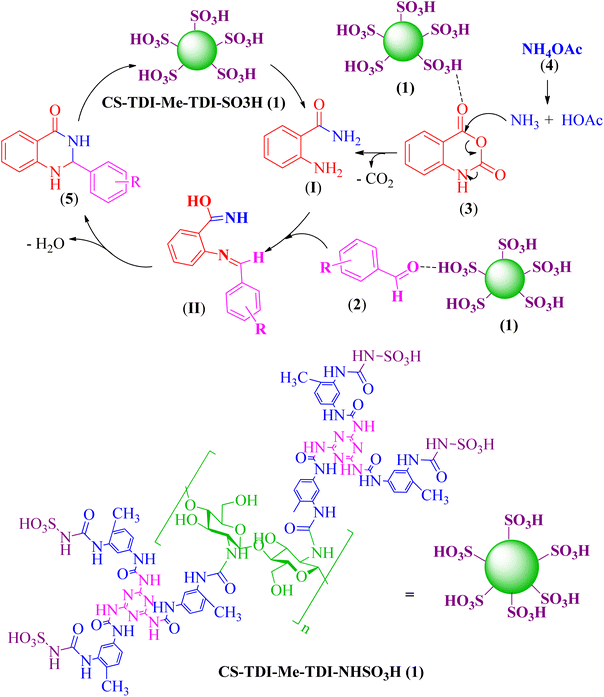 |
| Scheme 2 The proposed mechanism for the synthesis of 2,3-dihydroquinazoline derivatives 5 catalyzed by the biobased multifunctional CS-TDI-Me-TDI-HNSO3H solid acid (1). | |
Also, the reusability of CS-TDI-Me-TDI-HNSO3H solid acid catalyst (1) was studied in the synthesis of 2,3-dihydroquinazoline derivative 5a. For this purpose, the CS-TDI-Me-TDI-HNSO3H (1) was separated by filtration, washed with water and acetone, and then dried at 70 °C for 24 h. The recycled organocatalyst in each run was used after activation for the preparation of 2,3-dihydroquinazoline derivative 5a in the next run. This reaction was repeated up to five times and no significant reduction was observed in the CS-TDI-Me-TDI-HNSO3H organocatalyst (1) efficiency (Fig. 6).
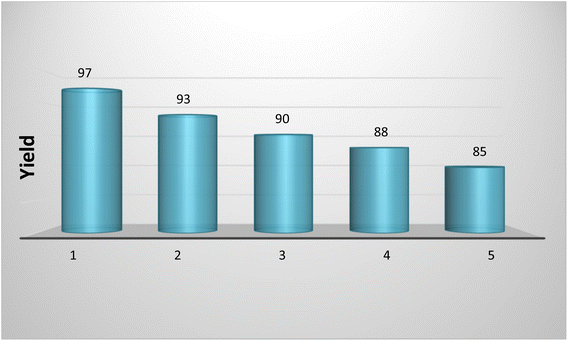 |
| Fig. 6 Reusability of the CS-TDI-Me-TDI-HNSO3H organocatalyst (1) in the model reaction to afford 5a. | |
Table 3 compares the efficiency of dendritic CS-TDI-Me-TDI-HNSO3H organocatalyst (1) with other catalysts for the synthesis of desired 2,3-dihydroquinazoline derivative 5a. For this comparison, several parameters, e.g., the reaction time, temperature, and the reaction yield, were taken into consideration. It can be seen that CS-TDI-Me-TDI-HNSO3H heterogeneous catalyst (1) showed higher efficiency than previously reported catalysts for the synthesis of 2,3-dihydroquinazoline derivatives.
Table 3 Comparison of the catalytic efficiency of CS-TDI-Me-TDI-HNSO3H (1) with other heterogeneous catalysts for 4-cholorobenzaldhyde
Entry |
Catalyst |
Time (min) |
Solvent/temperature conditions |
Yield (%) |
Reference |
1 |
CS-TDI-Me-TDI-HNSO3H (1) |
15 |
EtOH/reflux |
97 |
This work |
2 |
Wang-OSO3H |
40 |
H2O/100 °C |
84 |
108 |
3 |
Titanium silicon oxide nanopowder |
120 |
H2O/100 °C |
94 |
112 |
4 |
Montmorillonite-KSF |
150 |
Solvent-free/100 °C |
93 |
113 |
5 |
Al(H2PO4)3 |
540 |
Solvent-free/100 °C |
70 |
114 |
6 |
Co-aminobenzamid@Al-SBA-15 |
24 |
EtOH/reflux |
96 |
115 |
Experimental
Materials and methods
The CS-TDI-Me-TDI-HNSO3H nanomaterial (1) was purely prepared by modifing of known methods for similar materials having the same functional groups. Chitosan (CS, MW = 100
000–300
000 Da) was obtained from Acros Organics. Melamine (Me) and triethylamine (TEA) were provided by Sigma-Aldrich. Tetrahydrofuran (THF), sulfamic acid (H2NSO3H), and toluene-2,4-diisocyanate (TDI) were purchased from Merck-Millipore. Other chemical compounds were supplied by Merck and Aldrich Chemical Co. Characterization of the heterogeneous CS-TDI-Me-TDI-H2NSO3H (1) organocatalyst was performed using FESEM (TESCAN-MIRA III), EDS (TESCAN-MIRA II), TGA (STA 504, Bahr Co.), and XRD (Bourevestnik DRON-8) analyses. 1H NMR (500 MHz) spectra recorded on a Bruker DRX-500 Avance spectrometers in DMSO, as the solvent, at ambient temperature. FT-IR spectra were recorded as KBr pellets on a Shimadzu FT-IR-8400S spectrometer. Analytical thin-layer chromatography (TLC) was performed using Merck 0.2 mm silica gel 60F-254 Al-plates for reaction monitoring. Melting points were determined using an Electrothermal 9100 apparatus.
General method for preparation of CS-TDI-Me-TDI-HNSO3H organocatalyst (1). To a round button flask, a mixture of melamine (4 mmol, 0.5 g), TDI (12 mmol, 2 mL) and THF (15 mL) was added and stirred at room temperature under nitrogen atmosphere for 24 h. The obtained white solid (I) was filtered off and washed with THF, then dried in a vacuum oven at 60 °C for 12 h (Scheme 3). Also, H3N+SO3− (8 mmol, 0.776 g) and TEA (10 mmol, 1.4 mL) were added to THF (5 mL) and stirred for 2 h. Subsequently, the white solid (I) was added to the mixture and stirred for another 24 h at room temperature. Then, CS (0.5 g) was added and refluxed for 24 h under nitrogen atmosphere. Finally, the obtained white powder was filtered and washed with THF and EtOH and dried at 60 °C for 24 h (Scheme 3).
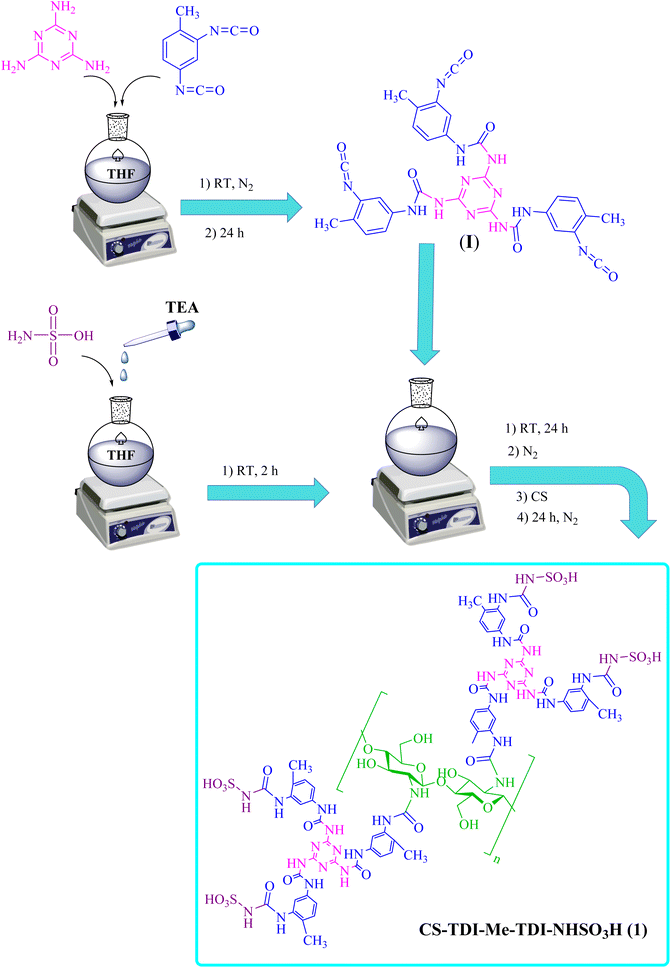 |
| Scheme 3 Schematic representation of CS-TDI-Me-TDI-HNSO3H (1) preparation steps. | |
General procedure for the synthesis of 2,3-dihydroquinazoline derivatives. A mixture of aldehyde derivatives (2, 0.5 mmol), isatoic anhydride (3, 0.5 mmol), ammonium acetate (4, 1.5 mmol), CS-TDI-Me-TDI- HNSO3H (1, 15 mg), and EtOH (5 mL) was stirred at 80 °C. After completion of the reaction, the organocatalyst was filtered and washed with acetone. The products were purified by recrystallization from EtOH. The products were identified by melting point measurement, FT-IR and 1HNMR spectroscopy.
Selected spectral data.
2-(4-Chlorophenyl)-2,3-dihydroquinazolin-4(1H)-one (2a). Melting point: 212–214 °C; FTIR (KBr, cm−1): 3305, 3184, 3062, 1654, 1606, 1431, 1090, 749 cm−1. 1H NMR (500 MHz, DMSO-d6): δH (ppm) = 5.77 (s, 1H, CH), 6.68 (t, 1H, Ar–H), 6.74 (d, 1H, Ar–H), 7.10 (s, 1H, NH), 7.24 (t, 1H, Ar–H), 7.45 (d, 1H, Ar–H), 7.50 (d, 1H, Ar–H), 7.61 (d, 1H, Ar–H), 8.27 (s, 1H, CONH).
2-(2-Chlorophenyl)-2,3-dihydroquinazolin-4(1H)-one (5b). Melting point: 207–209 °C; FTIR (cm−1): 3310, 3207, 3050, 1660, 1492, 1479; 1H NMR (500 MHz, DMSO-d6): δH (ppm) 6.14 (s, 1H), 6.72 (t, 1H, J = 7.5 Hz), 6.77 (d, 1H, J = 8.1 Hz), 7.01 (s, 1H), 7.26 (t, 1H, J = 7.7 Hz), 7.40 (d, 2H, J = 4.1 Hz), 7.49 (d, 1H, J = 4.1 Hz), 7.66 (d, 2H, J = 6.7, Hz), 8.21 (s, 1H).
2-(4-Methylphenyl)-2,3-dihydroquinazolin-4(1H)-one (5i). Melting point: 199–202 °C; FTIR (cm−1): 3300, 3170, 3032, 2931, 2827, 1510, 1249; 1H NMR (500 MHz, DMSO-d6): δH (ppm) 2.29 (s, 3H), 5.70 (s, 1H), 6.66 (t, 1H, J = 7.4 Hz), 6.73 (d, 1H, J = 8.1 Hz), 7.04 (s, 1H), 7.19 (d, J = 7.6 Hz, 1H), 7.23 (t, J = 8.1 Hz, 1H), 7.37 (d, J = 7.8 Hz, 2H), 7.61 (d, J = 7.6 Hz, 1H), 8.22 (s, 1H); δC (ppm) 13C NMR (125 MHz, DMSO-d6): 162.70, 152.90, 141.98, 134.99, 130.28, 129.65, 128.21, 127.64, 127.58, 126.88, 126.30, 121.23, 21.23.
2-(4-Methoxylphenyl)-2,3-dihydroquinazolin-4(1H)-one (5j). Melting point: 179–181 °C; FTIR (cm−1): 3301, 3170, 3029, 2930, 2825, 1511, 1248; 1H NMR (500 MHz, DMSO-d6): δH (ppm) 3.74 (s, 3H), 5.70 (s, 1H), 6.67 (t, 1H, J = 7.6 Hz), 6.74 (d, 1H, J = 8.1 Hz), 6.95 (d, 2H, J = 8.4), 7.00 (s, 1H), 7.23 (t, J = 8.0 Hz, 1H), 7.42 (d, J = 7.6 Hz, 2H), 7.61 (d, J = 7.6 Hz, 1H), 8.17 (s, 1H); 13C NMR (125 MHz, DMSO-d6): δC (ppm) 162.62, 152.84, 135.49, 135.14, 130.07, 126.94, 126.79, 126.37, 124.56, 120.92, 118.30, 114.36, 55.74.
Conclusions
In this paper, a new heterogeneous acidic catalyst based on the renewable and biodegradable chitosan polymer, i.e., CS-TDI-Me-TDI-HNSO3H, was prepared and characterized using various spectral and analytical techniques. Subsequently, CS-TDI-Me-TDI-HNSO3H was used, as a solid acid, for the synthesis of 2,3-dihydroquinazoline derivatives under green conditions. The desired 2,3-dihydroquinazoline derivatives were prepared in high to excellent yields under optimal conditions. Low catalyst loading, mild reaction conditions and very short reaction times as well as reusability of the catalyst for at least four consecutive catalytic cycles without significant loss of its activity are the advantages of this new protocol.
Conflicts of interest
There are no conflicts to declare.
Acknowledgements
We are grateful for the financial support from The Research Council of Iran University of Science and Technology (IUST), Tehran, Iran (Grant No. 160/20969). We would also like to acknowledge the support of the Iran Nanotechnology Initiative Council (INIC).
References
- M. N. R. Kumar, React. Funct. Polym., 2000, 46, 1–27 CrossRef CAS.
- J. Dinoro, M. Maher, S. Talebian, M. Jafarkhani, M. Mehrali, G. Orive, J. Foroughi, M. S. Lord and A. Dolatshahi-Pirouz, Biomaterials, 2019, 214, 119214 CrossRef CAS PubMed.
- R. F. B. de Souza, F. C. B. de Souza, C. Rodrigues, B. Drouin, K. C. Popat, D. Mantovani and Â. M. Moraes, Mater. Sci. Eng., C, 2019, 94, 364–375 CrossRef PubMed.
- A. Barbetta, A. Carrino, M. Costantini and M. Dentini, Soft Matter, 2010, 6, 5213–5224 RSC.
- Y. Cohen, R. Rutenberg, G. Cohen, B. Veltman, R. Gvirtz, E. Fallik, D. Danino, E. Eltzov and E. Poverenov, ACS Appl. Bio Mater., 2020, 3, 2209–2217 CrossRef CAS PubMed.
- C. Cui, Q. Fu, L. Meng, S. Hao, R. Dai and J. Yang, ACS Appl. Bio Mater., 2021, 4, 85–121 CrossRef CAS PubMed.
- L. Xu, Z. Chu, H. Wang, L. Cai, Z. Tu, H. Liu, C. Zhu, H. Shi, D. Pan, J. Pan and X. Fei, ACS Appl. Bio Mater., 2019, 2, 3429–3438 CrossRef CAS PubMed.
- B. Zhao, C. Lou, Q. Zhou, Y. Zhu, W. Li and M. Jingshan, RSC Adv., 2022, 12, 8256–8262 RSC.
- R. A. Muzzarelli, J. Boudrant, D. Meyer, N. Manno, M. DeMarchis and M. G. Paoletti, Carbohydr. Polym., 2012, 87, 995–1012 CrossRef CAS.
- M. G. Dekamin, E. Kazemi, Z. Karimi, M. Mohammadalipoor and M. R. Naimi-Jamal, Int. J. Biol. Macromol., 2016, 93, 767–774 CrossRef CAS PubMed.
- H. Hamedi, S. Moradi, S. M. Hudson, A. E. Tonelli and M. W. King, Carbohydr. Polym., 2022, 119100 CrossRef CAS PubMed.
- S. Torkaman, H. Rahmani, A. Ashori and S. H. M. Najafi, Carbohydr. Polym., 2021, 258, 117675 CrossRef CAS PubMed.
- N. Rostami, M. G. Dekamin, E. Valiey and H. FaniMoghadam, RSC Adv., 2022, 12, 21742–21759 RSC.
- X. Chen, H. Yang and N. Yan, Chem.–Eur. J., 2016, 22, 13402–13421 CrossRef CAS PubMed.
- A. El Kadib, ChemSusChem, 2015, 8, 217–244 CrossRef CAS PubMed.
- B. List, R. A. Lerner and C. F. Barbas, J. Am. Chem. Soc., 2000, 122, 2395–2396 CrossRef CAS.
- D. W. C. MacMillan, Nature, 2008, 455, 304–308 CrossRef CAS PubMed.
- A. Antenucci, S. Dughera and P. Renzi, ChemSusChem, 2021, 14, 2785–2853 CrossRef CAS PubMed.
- M. Debruyne, V. Van Speybroeck, P. Van Der Voort and C. V. Stevens, Green Chem., 2021, 23, 7361–7434 RSC.
- F. Khan and S. R. Ahmad, Macromol. Biosci., 2013, 13, 395–421 CrossRef CAS PubMed.
- S. K. L. Levengood and M. Zhang, J. Mater. Chem. B, 2014, 2, 3161–3184 RSC.
- J. Wróblewska-Krepsztul, T. Rydzkowski, I. Michalska-Pożoga and V. K. Thakur, Nanomaterials, 2019, 9, 404 CrossRef PubMed.
- M. Lee, B.-Y. Chen and W. Den, Appl. Sci., 2015, 5, 1272–1283 CrossRef CAS.
- M. U. A. Khan, S. Haider, M. A. Raza, S. A. Shah, S. I. Abd Razak, M. R. A. Kadir, F. Subhan and A. Haider, Int. J. Biol. Macromol., 2021, 192, 820–831 CrossRef CAS PubMed.
- F. Li, S. Y. H. Abdalkarim, H.-Y. Yu, J. Zhu, Y. Zhou and Y. Guan, ACS Appl. Bio Mater., 2020, 3, 1944–1954 CrossRef CAS PubMed.
- S. Mukherjee, J. W. Yang, S. Hoffmann and B. List, Chem. Rev., 2007, 107, 5471–5569 CrossRef CAS PubMed.
- J. Alkabli, M. A. Rizk, R. F. Elshaarawy and W. El-Sayed, Int. J. Biol. Macromol., 2021, 184, 454–462 CrossRef CAS PubMed.
- Z. Kheilkordi, G. Mohammadi Ziarani, F. mohajer, A. Badiei and M. Sillanpää, RSC Adv., 2022, 12, 12672–12701 RSC.
- N. Rostami, M. G. Dekamin, E. Valiey and H. Fanimoghadam, Sci. Rep., 2022, 12, 8642 CrossRef CAS PubMed.
- Z. Alirezvani, M. G. Dekamin and E. Valiey, Sci. Rep., 2019, 9, 17758 CrossRef PubMed.
- E. Valiey, M. G. Dekamin and Z. Alirezvani, Int. J. Biol. Macromol., 2019, 129, 407–421 CrossRef CAS PubMed.
- Z. Alirezvani, M. G. Dekamin, F. Davoodi and E. Valiey, ChemistrySelect, 2018, 3, 10450–10463 CrossRef CAS.
- M. G. Dekamin, S. Z. Peyman, Z. Karimi, S. Javanshir, M. R. Naimi-Jamal and M. Barikani, Int. J. Biol. Macromol., 2016, 87, 172–179 CrossRef CAS PubMed.
- M. G. Dekamin, Z. Karimi, Z. Latifidoost, S. Ilkhanizadeh, H. Daemi, M. R. Naimi-Jamal and M. Barikani, Int. J. Biol. Macromol., 2018, 108, 1273–1280 CrossRef CAS PubMed.
- M. G. Dekamin, S. Ilkhanizadeh, Z. Latifidoost, H. Daemi, Z. Karimi and M. Barikani, RSC Adv., 2014, 4, 56658–56664 RSC.
- G. Rajesh Krishnan and K. Sreekumar, in New and Future Developments in Catalysis, ed. S. L. Suib, Elsevier, Amsterdam, 2013, pp. 343–364, DOI:10.1016/B978-0-444-53876-5.00016-7.
- S. Ilkhanizadeh, J. Khalafy and M. G. Dekamin, Int. J. Biol. Macromol., 2019, 140, 605–613 CrossRef CAS PubMed.
- M. G. Dekamin, M. Azimoshan and L. Ramezani, Green Chem., 2013, 15, 811–820 RSC.
- X. Chen, S. Song, H. Li, G. k. Gözaydın and N. Yan, Acc. Chem. Res., 2021, 54, 1711–1722 CrossRef CAS PubMed.
- V. Mourya and N. N. Inamdar, React. Funct. Polym., 2008, 68, 1013–1051 CrossRef CAS.
- X. Fei Liu, Y. Lin Guan, D. Zhi Yang, Z. Li and K. De Yao, J. Appl. Polym. Sci., 2001, 79, 1324–1335 CrossRef.
- M. R. Kumar, R. A. Muzzarelli, C. Muzzarelli, H. Sashiwa and A. Domb, Chem. Rev., 2004, 104, 6017–6084 CrossRef PubMed.
- Q. Li, E. Dunn, E. Grandmaison and M. F. Goosen, J. Bioact. Compat. Polym., 1992, 7, 370–397 CrossRef CAS.
- T. Chandy and C.
P. Sharma, Biomater., Artif. Cells, Artif. Organs, 1990, 18, 1–24 CrossRef CAS PubMed.
- A. M. Heimbuck, T. R. Priddy-Arrington, M. L. Padgett, C. B. Llamas, H. H. Barnett, B. A. Bunnell and M. E. Caldorera-Moore, ACS Appl. Bio Mater., 2019, 2, 2879–2888 CrossRef CAS PubMed.
- J. Zhu, G. Jiang, G. Song, T. Liu, C. Cao, Y. Yang, Y. Zhang and W. Hong, ACS Appl. Bio Mater., 2019, 2, 5042–5052 CrossRef CAS PubMed.
- L.-Y. Zheng and J.-F. Zhu, Carbohydr. Polym., 2003, 54, 527–530 CrossRef CAS.
- J. Zhou, Z. Dong, H. Yang, Z. Shi, X. Zhou and R. Li, Appl. Surf. Sci., 2013, 279, 360–366 CrossRef CAS.
- P. K. Sahu, P. K. Sahu, S. K. Gupta and D. D. Agarwal, Ind. Eng. Chem. Res., 2014, 53, 2085–2091 CrossRef CAS.
- E. Guibal, Prog. Polym. Sci., 2005, 30, 71–109 CrossRef CAS.
- F. Quignard, A. Choplin and A. Domard, Langmuir, 2000, 16, 9106–9108 CrossRef CAS.
- X. Zhao, S. Li, Y. Hu, X. Zhang, L. Chen, C. Wang, L. Ma and Q. Zhang, Chem. Eng. J., 2022, 428, 131368 CrossRef CAS.
- N. Sudheesh, S. K. Sharma and R. S. Shukla, J. Mol. Catal. A: Chem., 2010, 321, 77–82 CrossRef CAS.
- M. Dohendou, K. Pakzad, Z. Nezafat, M. Nasrollahzadeh and M. G. Dekamin, Int. J. Biol. Macromol., 2021, 192, 771–819 CrossRef CAS PubMed.
- Z. Kheilkordi, G. M. Ziarani, F. Mohajer, A. Badiei and R. S. Varma, Green Chem., 2022, 24, 4304–4327 RSC.
- V. Mohanasrinivasan, M. Mishra, J. S. Paliwal, S. K. Singh, E. Selvarajan, V. Suganthi and C. Subathra Devi, 3 Biotech, 2014, 4, 167–175 CrossRef CAS PubMed.
- N. Li and R. Bai, Water Sci. Technol., 2006, 54, 103–113 CrossRef CAS PubMed.
- M. Barakat, Arabian J. Chem., 2011, 4, 361–377 CrossRef CAS.
- M. Sivakami, T. Gomathi, J. Venkatesan, H.-S. Jeong, S.-K. Kim and P. Sudha, Int. J. Biol. Macromol., 2013, 57, 204–212 CrossRef CAS PubMed.
- J. Ma and Y. Sahai, Carbohydr. Polym., 2013, 92, 955–975 CrossRef CAS PubMed.
- H. Vaghari, H. Jafarizadeh-Malmiri, A. Berenjian and N. Anarjan, Sustainable Chem. Processes, 2013, 1, 1–12 CrossRef.
- N. Yan and X. Chen, Nature, 2015, 524, 155–157 CrossRef CAS PubMed.
- D. Maiti, R. Das, T. Prabakar and S. Sen, Green Chem., 2022, 24, 3001–3008 RSC.
- M. Akram, N. Arshad, M. K. Aktan and A. Braem, ACS Appl. Bio Mater., 2020, 3, 7052–7060 CrossRef CAS PubMed.
- E. Valiey and M. G. Dekamin, RSC Adv., 2022, 12, 437–450 RSC.
- E. Valiey and M. G. Dekamin, Nanoscale Adv., 2022, 4, 294–308 RSC.
- D. Astruc, F. Lu and J. R. Aranzaes, Angew. Chem., Int. Ed., 2005, 44, 7852–7872 CrossRef CAS PubMed.
- S. Karami, M. G. Dekamin, E. Valiey and P. Shakib, New J. Chem., 2020, 44, 13952–13961 RSC.
- T. Chutimasakul, P. Na Nakhonpanom, W. Tirdtrakool, A. Intanin, T. Bunchuay, R. Chantiwas and J. Tantirungrotechai, RSC Adv., 2020, 10, 21009–21018 RSC.
- M. Hasanpour Galehban, B. Zeynizadeh and H. Mousavi, RSC Adv., 2022, 12, 16454–16478 RSC.
- L. Zhang, C. Yu, W. Zhao, Z. Hua, H. Chen, L. Li and J. Shi, J. Non-Cryst. Solids, 2007, 353, 4055–4061 CrossRef CAS.
- K. Li, J. Wang, Y. He, G. Cui, M. A. Abdulrazaq and Y. Yan, Chem. Eng. J., 2018, 351, 258–268 CrossRef CAS.
- H. Karimipour, A. Shahbazi and V. Vatanpour, J. Environ. Chem. Eng., 2021, 9, 104849 CrossRef CAS.
- D. W. Jenkins and S. M. Hudson, Chem. Rev., 2001, 101, 3245–3274 CrossRef CAS PubMed.
- X.-N. Zhao, G.-F. Hu, M. Tang, T.-T. Shi, X.-L. Guo, T.-T. Li and Z.-H. Zhang, RSC Adv., 2014, 4, 51089–51097 RSC.
- M. Cupery, Ind. Eng. Chem., 1938, 30, 627–631 CrossRef CAS.
- S. A. El-Hakam, S. E. Samra, S. M. El-Dafrawy, A. A. Ibrahim, R. S. Salama and A. I. Ahmed, RSC Adv., 2018, 8, 20517–20533 RSC.
- E. Valiey, M. G. Dekamin and Z. Alirezvani, Sci. Rep., 2021, 11, 11199 CrossRef CAS PubMed.
- P. R. Singh, D. U. Singh and S. D. Samant, Synlett, 2004, 2004, 1909–1912 CrossRef.
- Y. T. Reddy, P. R. Reddy, M. N. Reddy, B. Rajitha and P. A. Crooks, Synth. Commun., 2008, 38, 3201–3207 CrossRef CAS.
- R. Nagarajan, C. J. Magesh and P. T. Perumal, Synthesis, 2004, 2004, 69–74 Search PubMed.
- T.-S. Jin, G. Sun, Y. W. Li and T.-S. Li, Green Chem., 2002, 4, 255–256 RSC.
- B. Wang, J. He and R. C. Sun, Chin. Chem. Lett., 2010, 21, 794–797 CrossRef CAS.
- C. Huang, Y. Fu, H. Fu, Y. Jiang and Y. Zhao, Chem. Commun., 2008, 6333–6335 RSC.
- K. Pedrood, M. Sherafati, M. Mohammadi-Khanaposhtani, M. S. Asgari, S. Hosseini, H. Rastegar, B. Larijani, M. Mahdavi, P. Taslimi and Y. Erden, Int. J. Biol. Macromol., 2021, 170, 1–12 CrossRef CAS PubMed.
- I. MaajidáTaily, Chem. Commun., 2021, 57, 631–634 RSC.
- N. Nikooei, M. G. Dekamin and E. Valiey, Res. Chem. Intermed., 2020, 46, 3891–3909 CrossRef CAS.
- M. Rahman, I. Ling, N. Abdullah, R. Hashim and A. Hajra, RSC Adv., 2015, 5, 7755–7760 RSC.
- L. Fišnerová, B. Brunová, Z. Kocfeldová, J. Tíkalová, E. Maturová and J. Grimová, Collect. Czech. Chem. Commun., 1991, 56, 2373–2381 CrossRef.
- K. B. Gudasi, S. A. Patil, M. V. Kulkarni and M. Nethaji, Transition Met. Chem., 2009, 34, 325–330 CrossRef CAS.
- A. K. Tiwari, V. K. Singh, A. Bajpai, G. Shukla, S. Singh and A. K. Mishra, Eur. J. Med. Chem., 2007, 42, 1234–1238 CrossRef CAS PubMed.
- W. Armarego, Adv. Heterocycl. Chem., 1979, 24, 1–62 CrossRef CAS.
- S. H. Kim, S. H. Kim, T. H. Kim and J. N. Kim, Tetrahedron Lett., 2010, 51, 2774–2777 CrossRef CAS.
- P. H. Tran, T.-P. T. Bui, X.-Q. B. Lam and X.-T. T. Nguyen, RSC Adv., 2018, 8, 36392–36399 RSC.
- A. Harutyunyan, Russ. J. Org. Chem., 2016, 52, 1012–1017 CrossRef CAS.
- A. Mishra and S. Batra, Synthesis, 2009, 2009, 3077–3088 CrossRef.
- N. J. Vickers, Curr. Biol., 2017, 27, R713–R715 CrossRef CAS PubMed.
- T. H. Oude Munnink, E. G. de Vries, S. R. Vedelaar, H. Timmer-Bosscha, C. P. Schroder, A. H. Brouwers and M. N. Lub-de Hooge, Mol. Pharmaceutics, 2012, 9, 2995–3002 CrossRef CAS PubMed.
- A. A. Kalinin, A. D. Voloshina, N. V. Kulik, V. V. Zobov and V. A. Mamedov, Eur. J. Med. Chem., 2013, 66, 345–354 CrossRef CAS PubMed.
- H. Ghafuri, M. Ghafori Gorab and H. Dogari, Sci. Rep., 2022, 12, 4221 CrossRef CAS PubMed.
- M. Keshavarz, M. G. Dekamin, M. Mamaghani and M. Nikpassand, Sci. Rep., 2021, 11, 14457 CrossRef CAS PubMed.
- G. Yashwantrao, V. P. Jejurkar, R. Kshatriya and S. Saha, ACS Sustainable Chem. Eng., 2019, 7, 13551–13558 CrossRef CAS.
- N. Ghorashi, Z. Shokri, R. Moradi, A. Abdelrasoul and A. Rostami, RSC Adv., 2020, 10, 14254–14261 RSC.
- M. Mohammadi and A. Ghorbani-Choghamarani, RSC Adv., 2022, 12, 2770–2787 RSC.
- M. R. Anizadeh, M. A. Zolfigol, M. Yarie, M. Torabi and S. Azizian, Res. Chem. Intermed., 2020, 46, 3945–3960 CrossRef CAS.
- M. A. Diab, A. Z. El-Sonbati, D. M. D. Bader and M. S. Zoromba, J. Polym. Environ., 2012, 20, 29–36 CrossRef CAS.
- B. B. F. Mirjalili, Z. Zaghaghi and A. Monfared, J. Chin. Chem. Soc., 2020, 67, 197–201 CrossRef CAS.
- A. D. Rao, B. Vykunteswararao, T. Bhaskarkumar, N. R. Jogdand, D. Kalita, J. K. D. Lilakar, V. Siddaiah, P. D. Sanasi and A. Raghunadh, Tetrahedron Lett., 2015, 56, 4714–4717 CrossRef.
- H. Ghafuri, N. Goodarzi, A. Rashidizadeh and M. A. Douzandegi Fard, Res. Chem. Intermed., 2019, 45, 5027–5043 CrossRef CAS.
- H. FaniMoghadam, M. G. Dekamin and N. Rostami, Res. Chem. Intermed., 2022, 48, 3061–3089 CrossRef CAS.
- H. R. Shaterian and F. Rigi, Res. Chem. Intermed., 2015, 41, 721–738 CrossRef CAS.
- R. Mekala, M. Madhubabu, G. Dhanunjaya, S. Regati, K. Chandrasekhar and J. Sarva, Synth. Commun., 2017, 47, 121–130 CrossRef CAS.
- S. U. Tekale, S. B. Munde, S. S. Kauthale and R. P. Pawar, Org. Prep. Proced. Int., 2018, 50, 314–322 CrossRef CAS.
- H. R. Shaterian, A. R. Oveisi and M. Honarmand, Synth. Commun., 2010, 40, 1231–1242 CrossRef CAS.
- J. Safaei-Ghomi, R. Teymuri and A. Bakhtiari, BMC Chem., 2019, 13, 26 CrossRef PubMed.
|
This journal is © The Royal Society of Chemistry 2023 |
Click here to see how this site uses Cookies. View our privacy policy here.