DOI:
10.1039/D2RA07244K
(Paper)
RSC Adv., 2023,
13, 1580-1586
Organocatalytic chiral polymeric nanoparticles for asymmetric aldol reaction†
Received
15th November 2022
, Accepted 22nd December 2022
First published on 6th January 2023
Abstract
Chiral polymeric particles (CPPs) were studied extensively in recent years due to their importance in pharmaceutical applications. Here, nanosized CPPs were synthesized and applied as catalysts for direct asymmetric aldol reaction. The CPPs were prepared by miniemulsion or inverse miniemulsion based on various chiral amino acid derivatives and characterized by dynamic light scattering and scanning electron microscopy. The nanoparticles with spherical structure between 250 and 400 nm and high chiral surface area were used as catalysts in the aldol reaction at room temperature without additional solvent. L-tryptophan gave the highest enantiomeric excess, >86% with similar catalytic performance four times.
Introduction
Chirality is a key factor in molecular recognition, which is extremely important in many chemical, biological and medical applications.1 Chiral polymeric particles (CPPs) were used as typical functional polymeric particles in recent decades and explored as a special kind of chiral material in asymmetric catalysis, enantioselective release and crystallization, and enantioseparation.2 Efficient methods to produce and separate chiral compounds are important, in particular for enhancement of pharmaceuticals due to the different pharmacological and toxicological effects of the enantiomers.3 Previous work in our lab examined enantioselective crystallization of CPPs based on leucine, phenylalanine and alanine in their own supersaturated solution.1 Recently, a chiral membrane was formed by combining polysulphone and CPPs based on phenylalanine, and chiral recognition by the membrane was explored.4 In other works5–16 different kinds of functional CPPs were formed with narrow size distribution and controllable properties such as the surface area. Deng and coworkers managed to form hybrid organic and inorganic CPPs based on optically active helical polymers.17–26
One of the fastest growing fields in chemistry is organocatalysis which are formed by mostly natural products that does not have a metal which in substoichiometric amounts accelerates a reaction.27 Catalyzed direct asymmetric aldol reaction comprising a nonactivated ketone as a nucleophile is one of the most useful reactions for the formation of C–C chains. It is strongly preferred in the synthesis of natural products and drug molecules.28–35 The development of methods to achieve stereoselective ‘direct’ aldol reactions of unmodified ketones and/or aldehydes is an important objective in modern aldol chemistry.36 Several strategies were investigated, and methods based on enzyme-, antibody-, organometallic-, and organo-catalysis were recently reported.37,38 The first successful catalyzed asymmetric aldol reaction with more than 98% enantiomeric excess, (ee) was achieved with tin(II) complexes that were prepared in situ from the addition of tin, (II) triflate to L-proline derivatives.39 O'Reilly and coworkers created new methods to form chiral micelles and nanoparticles for applications in organocatalysis.40–43 Laura Osorio-Planes and Miquel A. Pericàs have synthesized an organocatalysis based on a copolymerization of PS and TRIP where was proven to be enantioselective in the allylation of aldehydes.44 Chetan K. Modi and his lab-workers have explored a new inexpensive strategy to synthesize L-proline derivatives onto the silica matrix without the protecting/deprotecting steps which give 99% enantioselectivity in the application of asymmetric aldol reaction.45 Another research was published where the catalyst was prepared in situ by combining the tridentate ligand derived from 1,1′-Bi-2-naphthol, (BINOL), Ti(OiPr)4 and salicylic acid in the presence of trimethylsilyl chloride and triethyl amine.46 Tang and co-workers47 synthesized a pyrrolidine derivative with a terminal hydroxyl group, which efficiently catalyses the direct aldol reactions of aromatic and aliphatic aldehydes in neat acetone with high enantioselectivity, >99% ee. There are also many reports on enantioselective aldol reactions catalysed by organocatalysts. Among the various catalysts for direct asymmetric aldol reaction, a series of organocatalysts derived from L-proline48 are of particular significance as it is a bi-functional, abundant chiral molecule, inexpensive and available in both enantiomeric forms.29 Therefore, many efforts were made to modify the structure of L-proline to improve selectivity and reusability, reduce load, and avoid the use of an organic solvent.49–54 A study by Viozquez and coworkers reveals that nearly solvent-free conditions were applied in the aldol reaction between α-keto esters and ketones by using a proline derivative as a catalyst with up to 92% ee.52 However, as the direct asymmetric aldol reaction is a powerful strategy due to the concomitant creation and functionalization of stereogenic centers, developing novel catalysts for it is in great demand.55 Biocatalysts such as aldolases56–59 or catalytic antibodies60 were used in aldol reactions. In addition to proline, alanine, valine,61 aspartate, isoleucine, alanine, tetrazole and serine as catalysts for the aldol reaction furnished the corresponding β-hydroxy ketones in high yield of >99% ee.62 Tryptophan, a cyclic and natural amino acid bearing a primary amino function, catalyzes aldol reactions between various cyclic ketones and aromatic aldehydes in water with high enantioselectivity, (up to 96% ee).63
CPPs for asymmetric catalysis are also of huge interest. Deng et al, constructed CPPs by polyacetylene with ferrocenyl amino acid derived pendant groups that enabled the particles to be used as catalysts in an asymmetric aldol reaction.2 In our group, CPPs were synthesized based on N-oleoyl-D/L-proline and used as an organocatalyst to explore the efficiency of catalysis in the asymmetric aldol reaction in an organic solvent and in water.13 There are a few additional examples of aldol reactions catalyzed by CPPs,64–66 however the efficiency and recovery of the catalyst need to be enhanced.
In the present research, CPPs were synthesized in nanosize from several amino acids as an organocatalyst for the direct aldol reaction. The polymeric nanoparticles were formed by the miniemulsion and inverse miniemulsion methods, and their catalytic performance was tested for the asymmetric aldol reaction between aromatic aldehyde and cyclohexanone at room temperature in the absence of solvent.
Experimental
Chemicals
The following analytical grade chemicals were purchased from Aldrich and used without further purification: D/L-phe, triethylamine, acryloyl chloride, sodium hydroxide, hydrochloric acid, glutaric acid, N-hydroxysuccinimide, N,N′-dicyclohexylcarbodiimide, (DCC), 4-dimethylaminopyridine, (DMAP), azobisisobutyronitrile, (AIBN, 98%), ethanol, absolute, ethyl acetate, dichloromethane, methanol, (absolute), sodium bisulphate, (NaHSO4), sodium carbonate, (NaHCO3), sodium chloride, Tween 20, xylene and 2-propanol, Deionized water was purified by passage through an Elgastat spectrum reverse osmosis system, (Elga, High Wycombe, UK).
Synthesis of monomers
Protected amino acids. 20 mmol of L-leucine or L-phenylalanine methyl ester hydrochloride (1) were placed in a 250 mL round-bottomed flask and 90 mL of DCM were added. Triethylamine, (4.44 g, 6.1 mL, 44 mmol, 2.2 eq.) was added and the mixture was stirred, leading to full dissolution. The mixture was cooled in an ice-bath to 0–5 °C and acryloyl chloride (2, 1.8 mL, 22 mmol, 1.1 eq.) dissolved in 10 mL DCM was added dropwise for 1 h while keeping the temperature at 5–10 °C. The mixture was stirred overnight at room temperature; the clear DCM solvent was then evaporated to dryness, giving a white crude product, EtOAc, (100 mL) was added, and after shaking the solution well, the mixture was filtered by vacuum, and the solid, (HCl salts) was washed with EtOAc, (20 mL). The EtOAc phase was washed three times with acid (2 × 20 mL of 1 M NaHSO4), base 2 × (20 mL) of 5% NaHCO3, and saturated NaCl, 20 mL solution, dried with MgSO4 and filtered. The solvent was removed in vacuum by rotation evaporation to produce the monomer of protected amino acid (3), (90% yield).
Unprotected amino acids. L-Phenylalanine, (4.125 g, 25 mmol), L-tryptophan (5.106 g, 25 mmol) or L-proline (2.878 g, 25 mmol), (4) was placed in a 100 mL round-bottomed flask and 30 mL of water were added. Sodium hydroxide (2 g, 50 mmol, 2 eq.) was added (in portions) while stirring, leading to a clear solution. The mixture was cooled in an ice bath to 0–5 °C and 2 (2.0 mL, 25 mmol, 1 eq) was added dropwise for 15 min while keeping the temperature at 5–10 °C. After the addition was completed, the mixture was stirred for another 30 min at room temperature. The clear solution was acidified with 2.5 mL conc. (32%) HCl (slow addition) in which a white solid appeared, and more HCl (0.5 mL) was added dropwise up to a pH of 2. The precipitated product was filtered with suction. The flask was rinsed with cold water (20 mL) to collect the remaining solid, and the solid was washed with additional cold water (∼30 mL) and dried under vacuum to furnish the unprotected amino acid monomers (5) as a white solid in ∼50% yield.
Synthesis of the crosslinker
Glutaric acid, (6) (1.3 g, 10 mmol) and N-hydroxysuccinimide, (7) (4.6 g, 40 mmol) were placed in a 100 mL round-bottomed flask and 50 mL DCM was added. The mixture was cooled in an ice bath to 0–5 °C. DCC (4.54 g, 22 mmol) and DMAP (0.46 g, 3.7 mmol) were added slowly to the mixture and stirred for 15 minutes. The flask was taken to room temperature and left for 3 hours of further stirring until the solution became white. After the reaction was completed, the mixture was filtered by gravity. Ethyl acetate (100 mL) was added to the filtrate and washed with acid (2 × 20 mL of 1 M NaHSO4), base (2 × 20 mL of 5% NaHCO3) and saturated NaCl (20 mL). The solution was dried with MgSO4, filtered and evaporated to give the product, (8) as a white solid in 55% yield.
Miniemulsion polymerization of chiral polymeric nanoparticles
The continuous phase was prepared by dissolving SDS (12 mg) in water (4 mL). For the disperse phase, the monomer (500 mg), AIBN (10 mg) and hexadecane (10 mg) were dissolved in toluene (1 g). For the preparation of cross-linked nanoparticles, divinylbenzene (5 mol% concerning the monomer) was added to the dispersed phase. Both phases were mixed and stirred for 30 min and emulsified by ultrasonication (1/4′′ tip; 5 min, 10 s pulse and 1 s pause, 40% amplitude) while cooling in an ice-water bath. The resulting miniemulsions were stirred at 74 °C for 16 h, cooled to room temperature and filtered.
The continuous phase was prepared by dissolving Tween 20 (150 mg) in Xylene (5.15 mL). For the disperse phase, the monomer (200 mg) and AIBN (10 mg) were dissolved in water (1.15 mL). For the preparation of cross-linked nanoparticles, glutaric acid bis, N-hydroxysuccinimide ester (5 mol% concerning the monomer) was added to the dispersed phase. Both phases were mixed and stirred for 30 min and then emulsified by ultrasonication (1/4′′ tip; 5 min, 37% amplitude) while cooling in an ice-water bath. The resulting miniemulsions were stirred at 74 °C for 16 h, cooled to room temperature and centrifuged 3 times with water and ethanol.
Characterization techniques
NMR spectra were recorded by a Bruker DZH 400/54400 MHz spectrometer. FTIR spectroscopy was carried out using a Thermo Scientific Nicolet iS10 FTIR spectrometer equipped with a Smart iTR attenuated total reflectance sampler containing a single bounce diamond crystal. Mass spectroscopy was carried out using an Agilent UHPLC 1290 MSD 6545. DLS measurements were performed with a Zetasizer 3000 HSA (Malvern Instruments Ltd., UK) operating with a 4 mW HeNe laser (632, 8 nm), a detector positioned at a scattering angle of 173°, and a temperature-controlled jacket for the cuvette. SEM was performed with an LEO Gemini 1530 Zeiss microscope operated at 0.6 kV. Particle sizes were obtained from ≈0.1 wt% diluted dispersions on a Nicomp 380 submicron particle sizer. DSC measurements were performed with a Mettler Toledo model DSC 822e equipped with a liquid-nitrogen cooling accessory and calibrated with indium; samples were heated from 25 to 350 °C at 5 °C min−1 under N2 flow.
Results and discussion
Protected and unprotected chiral amino acid monomers (Fig. 1 and 2) were synthesized in 70% and 60% yield respectively. The yield difference between the two types of chiral monomers is due to their different reactivity. Side products obtained in the reaction with unprotected monomers led to lower yield. All monomers were characterized by 1H and 13C nuclear magnetic resonance (NMR), mass spectrometry (MS) and Fourier transform infrared (FTIR) spectroscopy (see Table S1 in the ESI.†)
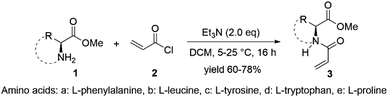 |
| Fig. 1 Synthesis of protected chiral amino acid monomers. | |
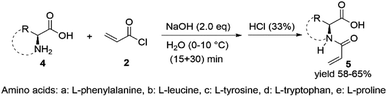 |
| Fig. 2 Synthesis of unprotected chiral amino acid monomers. | |
The formation of the monomer was probed with NMR by observing the transformation of the primary amine to the secondary amide structure. The specific amide peak appears around δ of 8.40 ppm, and no amine peak was detected. In addition to the amide peak, terminal ethylene peaks are seen at δ around 5.74, 6.10 and 6.30 ppm.
In FTIR spectra, specific absorption peaks were gathered at 1650 and between 3100 and 3300 cm−1 corresponding to the amide C
O and broad amide N–H stretching bands and at 1750 and 1250 cm−1 corresponding to ester C
O and stretching bands. Terminal alkene
C–H bond stretching peaks were seen around 3000 cm−1. For aromatic compounds additional C–H stretching bands around 3300 and 2000 cm−1 and overtones were seen. MS showed base m/z values equivalent to the mass of each monomer.
The cross-linker was synthesized by the Steglich esterification reaction where N-hydroxysuccimide (6) was used as alcohol and glutaric acid (7) was used as a carboxylic acid in the presence of DCM as a solvent. DCC as a coupling agent and DMAP as a catalyst(Fig. 3). Excess amounts of alcohol were used to consume carboxylic acid completely. The reaction was analyzed with NMR and FTIR (see Table S2,†) The specific ester absorption peaks were gathered at 1750 cm−1, and no peaks from alcohol were detected. The formed cross-linker was added to polymerization of the protected and unprotected monomers when a cross-linked polymeric amino acid catalyst was needed.
 |
| Fig. 3 Synthesis of the cross-linker. | |
Protected monomers were subjected to miniemulsion polymerization, and unprotected monomers were subjected to inverse miniemulsion,5,13,14,67,68 a well-known method and very versatile technique for the formation of a broad range of polymers and structured materials in confined geometries consisting of small, stable and narrowly distributed droplets in a continuous phase.69
In Fig. 4, scanning electron microscope (SEM) images of the polymeric particles show a spherical structure with a typical nanometric size. Poly-L-Leu-OMe (A) and poly-L-Phe-OMe (B) show an average particle size of 250 nm, however poly-L-proline (C) shows an average particle size of 400 nm due to the different synthetic methodology. In addition to SEM results, dynamic light scattering (DLS) shows (Fig. S6†) that the size distribution of the nanoparticles is narrow and monomodal with ca., 85% intensity and well protected uniformity.
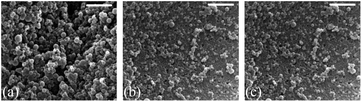 |
| Fig. 4 SEM images of polymeric (A) L-Leu-OMe (B) L-Phe-OMe and (C) L-Pro nanoparticles, scale bars = 1 μm). | |
The next step in our research focused on using the polymeric nanoparticles in asymmetric aldol reaction and exploring their catalytic activity. As a model aldol reaction we chose p-nitrobenzaldehyde (9) (0.66 mmol) and cyclohexanone (10) 6.6 mmol at room temperature for 48 hours without any external solvent and with polymeric amino acid nanoparticles as catalyst see (Fig. 5). We started examining the catalytic behavior of the nanoparticles of protected cross-linked polymers of L-phenylalanine (3a). However, unfortunately, although the yield of the reaction was good (64%), the catalyst could not impart enantioselectivity in the aldol reaction, (Table 1, entry 1). We hypothesized that the synthesis of racemic aldol product in the presence of (3a) could be due to two reasons. First – methyl ester protection of the phenylalanine prevents any hydrogen-bonding with the carbonyl group of cyclohexanone and second – the highly rigid framework of cross-linked polymer (3a) prevents it from folding and creating a shell-like cover from one side of the carbonyl group of cyclohexanone. The combined effect makes the carbonyl group of cyclohexanone prone to attack from both sides of the pi-bond, thereby producing a racemic aldol product.
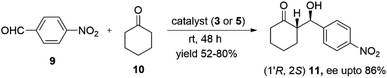 |
| Fig. 5 Enantioselective aldol reaction. | |
Table 1 Optimization table for the synthesis of 11 a
Entry |
Catalyst (3/5) |
Time (h) |
11 b (%) |
eec (%) |
Unless otherwise noted, 0.66 mmol of 9 and 6.6 mmol of 10 were mixed together. Yield of the isolated product. ee was determined by chiral HPLC analysis. Non-crosslinked and unprotected amino acid polymer. 0.1 mL of pyrrolidine was added. 0.04 mL of pyrrolidine was added. |
1 |
Crosslinked protected (L)-phenylalanine (3a) |
48 |
64 |
0 |
2 |
Non-crosslinked protected (L)-phenylalanine (5a) |
48 |
70 |
39 |
3d |
(L)-Phenylalanine (5a) |
48 |
68 |
61 |
4d |
(L)-Leucine (5b) |
48 |
52 |
0 |
5d |
(L)-Tyrosine (5c) |
48 |
59 |
57 |
6d |
(L)-Tryptophan (5d) |
48 |
72 |
86 |
7d |
(L)-Proline (5e) |
110 |
70 |
0 |
8d,e |
(L)-Proline (5e) |
5 |
80 |
0 |
9d,f |
(L)-Proline (5e) |
8 |
75 |
0 |
Next, we used a protected L-phenylalanine polymer without any cross-linker as a catalyst and performed aldol reaction. Interestingly, the non-cross-linked polymer successfully induced chirality in the asymmetric aldol reaction, and the product was obtained with moderate enantioselectivity (39%, entry 2). Inspired by this result, we synthesized non-cross-linked unprotected polymeric nanoparticles of L-phenylalanine, (5a) and used them as a catalyst in the asymmetric aldol reaction between cyclohexanone and p-nitrobenzaldehyde, resulting in the formation of (1′R,2S)-2-(hydroxy(4-nitrophenyl)methyl)-cyclohexan-1-one (9) with 61% ee and 68% yield (entry 3). Next, we examined the catalytic properties of unprotected non-cross-linked polymeric nanoparticles of the alkyl amino acid L-leucine (5b). However, it resulted no enantioselectivity with 52% yield (entry 4). We assumed that the alkyl group in the amino acid is not sufficiently bulky to provide sufficient capping from one side of the iminium ion; therefore, it furnished the racemic aldol product.
We proceeded to examine the catalytic activities of other nano-polymeric catalysts based on aromatic amino acids. Non-while the directed aldol product resulted in 57% ee (entry 5) when 5c was used as catalyst, while catalyst 5d provided the enantioenriched aldol product (11) under optimized conditions in 86% ee with 72% yield (entry 6). Efforts were then invested in synthesizing non-cross-linked unprotected polymeric nanoparticles of L-proline (5e). As L-proline-based organocatalyst was widely used to induce enantioselectivity in aldol-type reactions, we aimed to examine its catalytic activity as polymeric nanoparticles. Unlike previous amino acid catalysts (5e) was sparingly soluble in cyclohexanone, and the directed asymmetric aldol reaction took nearly 5 days without any external additives to render the racemic aldol product in 70% yield (entry 7).
To improve the solubility of 5e in the reaction mixture, 100 and 40 μL (one drop) of pyrrolidine were added in two separate test tubes to understand the catalytic behavior of (5e). In both cases, the solubility improved dramatically, resulting in a transparent reaction mixture, however unfortunately racemic aldol products were obtained with good yields (entries 8 and 9). The results are summarized in Table 1, and chromatograms are shown in Fig. S7–S10.†
We also realized the recyclability of the nano-polymeric unprotected amino acid catalyst 5d. Due to the high molecular weight of the catalyst it was easy to separate it from the solvents, reactants and products, which are of much lower molecular weight. In the presence of a non-polar solvent such as n-hexane, upon centrifugation the catalyst separates from the system. The recovered catalyst showed excellent catalytic activity in the next aldol reaction. We examined the catalyst for four cycles in which the catalytic activity of the polymeric catalyst was quite similar. However, from the 4th cycle the yield started to drop with erosion of enantioselectivity. The results are summarized in Table 2.
Table 2 Recycling of the amino acid catalyst (5d)
cycle |
Yielda (%) |
eeb (%) |
Yield of the isolated product 11. ee was determined by chiral HPLC analysis. |
1 |
72 |
86 |
2 |
70 |
86 |
3 |
70 |
85 |
4 |
68 |
83 |
Conclusions
In summary, we have designed new catalysts for the asymmetric aldol reaction, which are based on chiral amino acid derivatives. To form polymeric nanoparticles we have used, miniemulsion polymerization for protected amino acids monomer and inverse miniemulsion polymerization for unprotected amino acids monomer. The all polymeric nanoparticles were characterized by SEM and DLS and tested as organocatalysts for direct asymmetric aldol reaction between p-nitrobenzaldehyde and cyclohexanone at room temperature in the absence of any solvent. The findings gathered from HPLC instrument show that the synthesized polymeric nanoparticle can catalyse the aldol reaction giving enantioselectivity up to 86% ee of the relevant aldol adducts which is the highest ee results in the literature for this polymerization method. In addition, the synthesized polymeric organocatalysts can be recovered and reused for at least four cycles while the activity remains almost unchanged, showing promising potential as organocatalysts for the aldol reactions. Further studies on size, structure, and functionality of the CPPs can enhance the performance of possible new chiral organocatalysts in the future.
Conflicts of interest
There are no conflicts to declare.
Acknowledgements
M. Abuaf thanks the Ministry of Aliyah and integration and the Bar-Ilan President scholarship program for a PhD, scholarship.
References
- M. Abuaf and Y. Mastai, Synthesis of Multi Amino Acid Chiral Polymeric Microparticles for Enantioselective Chemistry, Macromol. Chem. Phys., 2020, 221(24), 2000328 CrossRef CAS.
- Y. Zhang, H. Huang, B. Zhao and J. Deng, Preparation and Applications of Chiral Polymeric Particles, Isr. J. Chem., 2018, 58(12), 1286–1298 CrossRef CAS.
- J. E. Rekoske, Chiral separations, AIChE J., 2001, 47(1), 2 CrossRef CAS.
- M. Abuaf and Y. Mastai, Electrospinning of polymer nanofibers based on chiral polymeric nanoparticles, Polym. Adv. Technol., 2022, 3878–3886 CrossRef CAS.
- L. C. Preiss, L. Werber, V. Fischer, S. Hanif, K. Landfester, Y. Mastai and R. Muñoz-Espí, Amino-acid-based chiral nanoparticles for enantioselective crystallization, Adv. Mater., 2015, 27(17), 2728–2732 CrossRef CAS PubMed.
- D. D. Medina, J. Goldshtein, S. Margel and Y. Mastai, Enantioselective crystallization on chiral polymeric microspheres, Adv. Funct. Mater., 2007, 17(6), 944–950 CrossRef CAS.
- A. Gabashvili, D. D. Medina, A. Gedanken and Y. Mastai, Templating mesoporous silica with chiral block copolymers and its application for enantioselective separation, J. Phys. Chem. B, 2007, 111(38), 11105–11110 CrossRef CAS PubMed.
- P. Paik, A. Gedanken and Y. Mastai, Enantioselective separation using chiral mesoporous spherical silica prepared by templating of chiral block copolymers, ACS Appl. Mater. Interfaces, 2009, 1(8), 1834–1842 CrossRef CAS PubMed.
- Y. Mastai, M. Sedlák, H. Cölfen and M. Antonietti, The separation of racemic crystals into enantiomers by chiral block copolymers, Chem.–Eur. J., 2002, 8(11), 2429–2437 CrossRef CAS PubMed.
- P. Paik, A. Gedanken and Y. Mastai, Chiral-mesoporous-polypyrrole nanoparticles: Its chiral recognition abilities and use in enantioselective separation, J. Mater. Chem., 2010, 20(20), 4085–4093 RSC.
- P. Paik, A. Gedanken and Y. Mastai, Chiral separation abilities: Aspartic acid block copolymer-imprinted mesoporous silica, Microporous Mesoporous Mater., 2010, 129(1–2), 82–89 CrossRef CAS.
- T. Menahem and Y. Mastai, Chiral soluble polymers and microspheres for enantioselective crystallization, J. Polym. Sci., Part A: Polym. Chem., 2006, 44(9), 3009–3017 CrossRef CAS.
- S. R. Adler and Y. Mastai, Chiral polymeric nanoparticles for aldol reaction, React. Funct. Polym., 2015, 96, 1–4 CrossRef CAS.
- T. Menahem and Y. Mastai, Enantioselective crystallization in miniemulsions based on chiral surfactants, New J. Chem., 2008, 32(6), 925–928 RSC.
- R. Muñoz-Espí, Y. Mastai, S. Gross and K. Landfester, Colloidal systems for crystallization processes from liquid phase, CrystEngComm, 2013, 15(12), 2175–2191 RSC.
- D. H. Dressler and Y. Mastai, Controlling polymorphism by crystallization on self-assembled multilayers, Cryst. Growth Des., 2007, 7(5), 847–850 CrossRef CAS.
- H. Zhang, B. Zhao and J. Deng, Optically Active Hybrid Materials Constructed from Helically Substituted Polyacetylenes, Chem. Rec., 2016, 16(2), 964–976 CrossRef CAS PubMed.
- B. Chen, J. Deng and W. Yang, Hollow Two-Layered Chiral Nanoparticles Consisting of Optically Active Helical Polymer/Silica: Preparation and Application for Enantioselective Crystallization, Adv. Funct. Mater., 2011, 21(12), 2345–2350 CrossRef CAS.
- X. Luo, J. Deng and W. Yang, Helix-Sense-Selective Polymerization of Achiral Substituted Acetylenes in Chiral Micelles, Angew. Chem., Int. Ed., 2011, 50(21), 4909–4912 CrossRef CAS PubMed.
- J. Deng, B. Chen, X. Luo and W. Yang, Synthesis of nano-latex particles of optically active helical substituted polyacetylenes via catalytic microemulsion polymerization in aqueous systems, Macromolecules, 2009, 42(4), 933–938 CrossRef CAS.
- J. Deng, X. Luo, W. Zhao and W. Yang, A novel type of optically active helical polymers: Synthesis and characterization of poly, N-propargylureas, J. Polym. Sci., Part A: Polym. Chem., 2008, 46(12), 4112–4121 CrossRef CAS.
- D. Zhang, C. Song, J. Deng and W. Yang, Chiral microspheres consisting purely of optically active helical substituted polyacetylene: the first preparation via precipitation polymerization and application in enantioselective crystallization, Macromolecules, 2012, 45(18), 7329–7338 CrossRef CAS.
- D. Zhang, C. Ren, W. Yang and J. Deng, Helical polymer as mimetic enzyme catalyzing asymmetric aldol reaction, Macromol. Rapid Commun., 2012, 33(8), 652–657 CrossRef CAS PubMed.
- Z. Zhang, J. Deng, W. Zhao, J. Wang and W. Yang, Synthesis of optically active poly, N-propargylsulfamides with helical conformation, J. Polym. Sci., Part A: Polym. Chem., 2007, 45(3), 500–508 CrossRef CAS.
- Y. Zhang and J. Deng, Chiral helical polymer materials derived from achiral monomers and their chiral applications, Polym. Chem., 2020, 5407–5423 RSC.
- L. Song, M. Pan, R. Zhao, J. Deng and Y. Wu, Recent advances, challenges and perspectives in enantioselective release, J. Controlled Release, 2020, 156–171 CrossRef CAS PubMed.
- P. Knochel and G. A. Molander, Comprehensive organic synthesis, Newnes, 2014 Search PubMed.
- A. G. Doyle and E. N. Jacobsen, Small-molecule H-bond donors in asymmetric catalysis, Chem, Rev., 2007, 107(12), 5713–5743 CrossRef CAS PubMed.
- H. Pellissier, Asymmetric organocatalysis, Tetrahedron, 2007, 38(63), 9267–9331 CrossRef.
- S. Mukherjee, J. W. Yang, S. Hoffmann and B. List, Asymmetric enamine catalysis, Chem, Rev., 2007, 107(12), 5471–5569 CrossRef CAS PubMed.
- J. G. Hernández and E. Juaristi, Recent efforts directed to the development of more sustainable asymmetric organocatalysis, Chem. Commun., 2012, 48(44), 5396–5409 RSC.
- K. Gopalaiah and H. B. Kagan, Use of nonfunctionalized enamides and enecarbamates in asymmetric synthesis, Chem. Rev., 2011, 111(8), 4599–4657 CrossRef CAS PubMed.
- V. Bisai, A. Bisai and V. K. Singh, Enantioselective organocatalytic aldol reaction using small organic molecules, Tetrahedron, 2012, 24(68), 4541–4580 CrossRef.
- S. G. Zlotin, A. S. Kucherenko and I. P. Beletskaya, Organocatalysis of asymmetric aldol reaction, Catalysts and reagents, Russ. Chem. Rev., 2009, 78(8), 737 CrossRef CAS.
- M. K. Ghorai, S. Samanta and S. Das, Synthesis of 3, 5-Disubstituted Cyclohex-2-en-1-one via a Five-Step Domino Reaction Catalyzed by Secondary Amines: Formation of, E-α, β-Unsaturated Methyl Ketones, Asian J. Org. Chem., 2013, 2(12), 1026–1030 CrossRef CAS.
- B. Alcaide and P. Almendros, The direct catalytic asymmetric aldol reaction, Eur. J. Org. Chem., 2002, 2002(10), 1595–1601 CrossRef.
- R. A. Rather, M. U. Khan and Z. N. Siddiqui, Sulphated alumina tungstic acid, SATA, a highly efficient and novel heterogeneous mesostructured catalyst for the synthesis of pyrazole carbonitrile derivatives and evaluation of green metrics, RSC Adv., 2020, 10(2), 818–827 RSC.
- D. E. Ward and V. Jheengut, Proline-catalyzed asymmetric aldol reactions of tetrahydro-4H-thiopyran-4-one with aldehydes, Tetrahedron Lett., 2004, 45(45), 8347–8350 CrossRef CAS.
- I. Shiina, Asymmetric M ukaiyama Aldol Reactions Using Chiral Diamine–Coordinated Sn, II Triflate: Development and Application to Natural Product Synthesis, Chem. Rec., 2014, 14(1), 144–183 CrossRef CAS PubMed.
- J. Skey and R. K. O'reilly, Synthesis of chiral micelles and nanoparticles from amino acid based monomers using RAFT polymerization, J. Polym. Sci., Part A: Polym. Chem., 2008, 46(11), 3690–3702 CrossRef CAS.
- J. Skey, H. Willcock, M. Lammens, F. Du Prez and R. K. O'Reilly, Synthesis and self-assembly of amphiphilic chiral poly, amino acid star polymers, Macromolecules, 2010, 43(14), 5949–5955 CrossRef CAS.
- J. Skey, C. F. Hansell and R. K. O'Reilly, Stabilization of amino acid derived diblock copolymer micelles through favorable D: L side chain interactions, Macromolecules, 2010, 43(3), 1309–1318 CrossRef CAS.
- B. L. Moore and R. K. O'Reilly, Preparation of chiral amino acid materials and the study of their interactions with 1, 1-Bi-2-naphthol, J. Polym. Sci., Part A: Polym. Chem., 2012, 50(17), 3567–3574 CrossRef CAS.
- L. Clot-Almenara, C. Rodríguez-Escrich, L. Osorio-Planes and M. A. Pericàs, Polystyrene-supported TRIP: a highly recyclable catalyst for batch and flow enantioselective allylation of aldehydes, ACS Catal., 2016, 6(11), 7647–7651 CrossRef CAS.
- P. Lakhani and C. K. Modi, Spick-and-span protocol for designing of silica-supported enantioselective organocatalyst for the asymmetric aldol reaction, Mol. Catal., 2022, 525, 112359 CrossRef CAS.
- R. A. Singer and E. M. Carreira, An in situ procedure for catalytic, enantioselective acetate aldol addition, Application to the synthesis of, R, (−)-epinephrine, Tetrahedron Lett., 1997, 38(6), 927–930 CrossRef CAS.
- Z. Tang, F. Jiang, L.-T. Yu, X. Cui, L.-Z. Gong, A.-Q. Mi, Y.-Z. Jiang and Y.-D. Wu, Novel small organic molecules for a highly enantioselective direct aldol reaction, J, Am, Chem, Soc., 2003, 125(18), 5262–5263 CrossRef CAS PubMed.
- B. List, P. Pojarliev and C. Castello, Proline-catalyzed asymmetric aldol reactions between ketones and α-unsubstituted aldehydes, Org, Lett., 2001, 3(4), 573–575 CrossRef CAS PubMed.
- L. Zhang, S. Luo and J. P. Cheng, Non-covalent immobilization of asymmetric organocatalysts, Catal. Sci. Technol., 2011, 1(4), 507–516 RSC.
- Q. Zhao, Y.-h. Lam, M. Kheirabadi, C. Xu, K. Houk and C. E. Schafmeister, Hydrophobic substituent effects on proline catalysis of aldol reactions in water, J. Org. Chem., 2012, 77(10), 4784–4792 CrossRef CAS PubMed.
- Y. Q. Fu, Y. J. An, W. M. Liu, Z. C. Li, G. Zhang and J.-C. Tao, Highly diastereo-and enantioselective direct aldol reaction catalyzed by simple amphiphilic proline derivatives, Catal. Lett., 2008, 124(3), 397–404 CrossRef CAS.
- S. F. Viozquez, A. Bañón-Caballero, G. Guillena, C. Najera and E. Gómez-Bengoa, Enantioselective direct aldol reaction of α-keto esters catalyzed by, S (a)-binam-d-prolinamide under quasi solvent-free conditions, Org. Biomol. Chem., 2012, 10(20), 4029–4035 RSC.
- J.-W. Xu, X.-K. Fu, X.-Y. Hu and C.-L. Wu, Simple and facile L-prolinamides derived from achiral cycloalkylamines as organocatalysts for the highly efficient large-scale asymmetric direct aldol reactions, Catal. Lett., 2011, 141(8), 1156–1163 CrossRef CAS.
- M. Lei, S. Xia, J. Wang, Z. Ge, T. Cheng and R. Li, An efficient dipeptide-catalyzed direct asymmetric aldol reaction of equimolar reactants in solid media, Chirality, 2010, 22(6), 580–586 CAS.
- Y. Yuan, Z. Guan and Y. He, Biocatalytic direct asymmetric aldol reaction using proteinase from Aspergillus melleus, Sci. China: Chem., 2013, 56(7), 939–944 CrossRef CAS.
- O. Eyrisch and W. D. Fessner, Disaccharide mimetics by enzymatic tandem aldol additions, Angew. Chem., Int. Ed. Engl., 1995, 34(15), 1639–1641 CrossRef CAS.
- T. Kimura, V. P. Vassilev, G.-J. Shen and C.-H. Wong, Enzymatic synthesis of β-hydroxy-α-amino acids based on recombinant D-and L-threonine aldolases, J. Am. Chem. Soc., 1997, 119(49), 11734–11742 CrossRef CAS.
- P. Nemec, J. Altmann, S. Marhold, H. Burda and H. H. Oelschlager, Neuroanatomy of magnetoreception: the superior colliculus involved in magnetic orientation in a mammal, Science, 2001, 294(5541), 366–368 CrossRef CAS PubMed.
- W. A. Greenberg, A. Varvak, S. R. Hanson, K. Wong, H. Huang, P. Chen and M. J. Burk, Development of an efficient, scalable, aldolase-catalyzed process for enantioselective synthesis of statin intermediates, Proc. Natl. Acad. Sci., 2004, 101(16), 5788–5793 CrossRef CAS PubMed.
- F. Tanaka and C. F. Barbas III, Antibody-catalyzed Aldol Reactions, Mod. Aldol React., 2004, 273–310 CAS.
- M. Amedjkouh, Primary amine catalyzed direct asymmetric aldol reaction assisted by water, Tetrahedron: Asymmetry, 2005, 16(8), 1411–1414 CrossRef CAS.
- A. Córdova, W. Zou, I. Ibrahem, E. Reyes, M. Engqvist and W.-W. Liao, Acyclic amino acid-catalyzed direct asymmetric aldol reactions: alanine, the simplest stereoselective organocatalyst, Chem. Commun., 2005,(28), 3586–3588 RSC.
- Z. Jiang, Z. Liang, X. Wu and Y. Lu, Asymmetric aldol reactions catalyzed by tryptophan in water, Chem. Commun., 2006,(26), 2801–2803 RSC.
- F. Bächle and A. Pfaltz, Screening of Chiral Organocatalysts for the Aldol Reaction by Mass Spectrometric Monitoring of the Retro Reaction, Chimia, 2012, 66, 570–605 CrossRef.
- S. Itsuno, Chiral polymer synthesis by means of repeated asymmetric reaction, Prog. Polym. Sci., 2005, 30(5), 540–558 CrossRef CAS.
- S. Itsuno and K. Komura, Highly stereoselective synthesis of chiral aldol polymers using repeated asymmetric Mukaiyama aldol reaction, Tetrahedron, 2002, 58(41), 8237–8246 CrossRef CAS.
- C. D. Anderson, E. D. Sudol and M. S. El-Aasser, 50 nm polystyrene particles via miniemulsion polymerization, Macromolecules, 2002, 35(2), 574–576 CrossRef CAS.
- K. Landfester, Miniemulsions for nanoparticle synthesis, Colloid Chem., 2003, 75–123 CAS.
- W. Xu, Z. Cheng, L. Zhang, Z. Zhang, J. Zhu, N. Zhou and X. Zhu, Synthesis and properties of crosslinked chiral nanoparticles via RAFT miniemulsion polymerization, J. Polym. Sci. A Polym. Chem., 2010, 48(6), 1324–1331 CrossRef CAS.
|
This journal is © The Royal Society of Chemistry 2023 |