DOI:
10.1039/D2RA06862A
(Paper)
RSC Adv., 2023,
13, 506-515
Catalyst-free synthesis of low-temperature thermally actuated shape memory polyurethanes with modified biobased plasticizers
Received
30th October 2022
, Accepted 13th December 2022
First published on 21st December 2022
Abstract
Recent years have seen research into developing specific application-based materials with particular components. Bio-based polyurethanes (PUs) with self-tightening effect through shape recovery at low temperature have been designed from sesame oil-based plasticizer (HSSO). Without using a catalyst, the produced plasticizer was used to create PU samples. In contrast, orcein-based PU has been created both with and without HSSO. The prepared samples have been analyzed through instrumental as well as chemical analyses for surface chemistry, thermal stability and morphology. The gel content and water absorption capacity of HSSO based PU samples has been observed to be 99.27% and 14.94%, respectively. Shape memory study of the PU samples revealed that HSSO-based PU showed fast shape recovery at 60 °C with shape recovery rate (Rr) and shape fixing rate (Rf) of 94.44% and 5%, respectively, in 150 seconds, whereas at 36 °C the sample showed 85% Rr in 15 minutes with 93.1196 N force and 52.78% Rr without force. Low-temperature thermal actuation and high water uptake highlight the prepared samples as suitable candidates for self-tightening structures in textile and biomedical fields.
1 Introduction
Stimulus-responsive restoration of a polymeric material to its original shape after specific deformation has opened a new dimension in material applications.1 These stimuli include, but are not limited to, changes in temperature,2 light,3 electricity,4 pH,5 and other similar variables. Biomedical devices such as cardiovascular stents,6 sutures,7 drug-eluting stents8 and clot removal devices,9 and tissue engineering,10 have made extensive use of SMPs due to their biocompatibility,11 biodegradability,11 and human body temperature shape recovery.12–14 With their tunable transition temperatures for shape recovery and high levels of biocompatibility, shape memory polyurethanes (SMPUs) have shown great promise as responsive materials for use in biomedical devices inside the human body,12–14 accompanied by coating material, packing materials and smart textile.15–17
However, environmental concerns and exhausted petroleum reserves have shifted the focus to SMPUs with biobased origin rather than petrochemical origin.18 Currently, vegetable oils like soyabean oil,19 castor oil,20 sunflower oil,21 jatropha oil22 and palm kernel oil,23 are the most common source for bio-based SMPUs (bio-SMPUs), but slow curing,24 catalytic constraint25,26 and reduced elastic strength27–29 has limited their applications in comparison to petrochemical based SMPUs. This is because the micro-phase separation of PU is negatively impacted by the lengthy dangling chains present in vegetable oil polyols (PUs). Researchers have tried to control the length of side chains either by bio-based polyester diols,24 or by rosin-based chain extender30 but found not ideal for biological applications due to their shape recovery below body temperature. PU based polyurethane SMPs had ability to recover its shape to maximum degree but different chain extenders and catalyst were applied to control their elastic properties25,31,32 as shown in Table 6. Considering these limitations, ecofriendly SMPUs, with recyclable nature but high mechanical strength, have always been in dire need.
In this research, modified sesame oil-based polyols have been utilized to formulate SMPUs, without any chain extender or catalyst, but with quick shape recovery at very low thermal actuation. Two step treatment i.e. epoxidation and hydroxylation, has been performed to obtain suitable polyol as plasticizer for shape memory property.
2 Materials and method
2.1 Materials
Sesame seed oil (SSO) extracted from sesame seed at commercial extracting unit, hydrogen peroxide (35%, Sigma-Aldrich), formic acid (DAEJUNG Chemicals & Metals), glacial acetic acid (100% pure, Merck KGaA), orcein reagent (Sigma-Aldrich), pure ethyl alcohol (Sigma-Aldrich), toluene 2,4-diisocyanate (TDI, extra pure, DAEJUNG Chemicals & Metals), benzene (Sigma-Aldrich), paraffin oil (Sigma-Aldrich) were utilized as raw materials in this practical work.
2.2 Method
2.2.1 Epoxidation of SSO (ESSO). A mixture of acetic acid (0.73 moles) and 35% hydrogen peroxide (2.35 moles) was refluxed at 40 °C for 15 minutes, SSO (0.08 moles) was added dropwise while stirring and refluxing (40 °C). After complete addition of SSO, refluxing was continued for 2.5 hours at 40 °C and 30 minutes at 130 °C. Then reaction mixture was allowed to cool at room temperature. The upper layer with bright yellow colour was separated and placed in desiccator for 6 hours. The final product (ESSO) was obtained with 90.22% yield.
2.2.2 Hydroxylation of ESSO (HSSO). A mixture of ethanol (0.804 moles), deionized water (1.66 moles) and 30 ml organic acids (acetic acid
:
formic acid by vol. 1
:
1) was heated at 65 °C with vigorous stirring (1500 rpm). ESSO (0.022 moles) was added dropwise and refluxed, while stirring, for 30 minutes at 65 °C. Then reaction mixture was allowed to cool at r.t. HSSO layer (lower layer) was separated from aqueous layer and placed in desiccator for 6 hours. 90% yield of HSSO (pale-yellow color) was obtained.
2.2.3 Preparation of HSSO-PU. HSSO (0.0074 moles) was heated at 60 °C, while stirring (650 rpm), for 5 minutes, and TDI (0.028 moles) was added dropwise while vigorously stirring at 60 °C to avoid bubbling. The mixture was heated for 10 minutes while vigorously stirring. Viscous dispersion was then shifted into mold and set to dry for 72 hours at room temperature. Very light-yellow thin layer of HSSO-based PU about 1 mm thickness was obtained. Proposed reaction scheme is presented in Scheme 1.
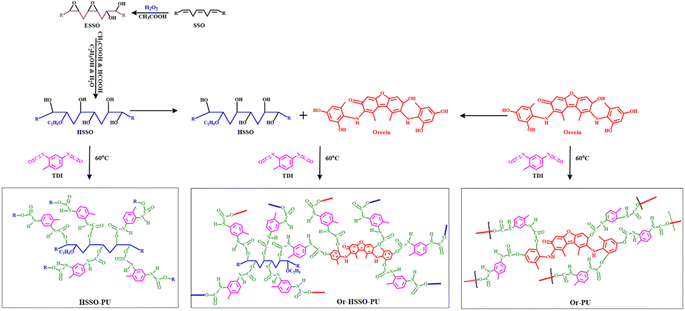 |
| Scheme 1 Synthesis of HSSO-PU, Or–HSSO-PU and Or-PU. | |
2.2.4 Preparation of Or-PU. Orcein (0.0004 moles) was dissolved with stirring in ethanol (0.338 moles) at r.t. and heated at 60 °C for 5 min. Then TDI (0.0417 moles) was added dropwise in 2 min and heated while stirring at 60 °C for 10 minutes. The viscous dark red material was shifted into plastic mold and allowed to cure for 72 hours. Or-PU with 1 mm thickness was obtained. Proposed reaction scheme is presented in Scheme 1.
2.2.5 Preparation of Or–HSSO-PU. Orcein (0.0004 moles) ethanol (0.338 moles) solution was mixed with HSSO (0.00165 moles) and stirred for 5 min. Then TDI (0.0556 moles) was added dropwise (in 3 minutes) with stirring and heating at 60 °C for 10 minutes. The dark reddish viscous liquid was shifted into mold and set to dry for 72 hours. Or–HSSO-PU with thickness of 1 mm was obtained. Proposed reaction scheme is presented in Scheme 1.
3 Characterizations
Synthesized PU samples have been analyzed through FTIR-(IRSpirit) Shimadzu with diamond ATR in range 500–4000 cm−1, TGA/DSC (STA SKZ1060A Industrial Co., Limited) from room temperature to 500 °C at ramp rate of 10 °C min−1 for 5 mg sample in Al crucible using oxidative environment and air as purge gas, light microscope (IRMECO GmbH & Co., IM-910). The morphology and surface roughness of synthesized PU composites have been studied by scanning electron microscope (SEM, ZEISS EVO, Carl Zeiss) and atomic force microscopy (AFM). Molar mass of treated SSO was determined by ebullioscopic method (eqn (1)) taking benzene as solvent.where ΔTb = elevation in boiling point of specific solvent, Kb = ebullioscopic boiling constant of specific solvent and m = molality of unknown sample. For benzene, the value of ebullioscopic boiling constant is 2.53 °C kg mol−1.33
Iodine value (IV) was determined by reported method.34 0.2 g sample with 10 ml chloroform was stirred with 30 ml of Hanus solution for 15 minutes. 10 ml of 15% KI and 100 ml DI water were added and titrated against 0.1 N Na2S2O3 till yellow colour, added 2–3 drops of starch indicator and titrated again till blue colour. Calculated IV by using eqn (2).
|
 | (2) |
where
B and
S are the volume used of Na
2S
2O
3 against blank solution and sample and
N is the normality of Na
2S
2O
3.
Epoxy value (EV) was determined by HCl–acetone titration method.35 0.25 g sample was stirred in 5 ml HCl (0.1 N) and 35 ml of acetone. 5 ml mixture, with 2–3 drops of indicator, was titrated against 0.1 N NaOH till pink color. EV value was calculated by eqn (3).
|
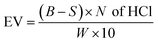 | (3) |
where
S and
B are the volume used of NaOH against sample and blank solution, and
W is the weight of sample.
Samples' density was determined by using mass/volume relationship.36 Gel contents were measured by a method reported in literature.37 Calculated amount of PU samples (HSSO-PU, Or–HSSO-PU and Or-PU) was soaked in 20 ml of dichloromethane (DCM) for 24 hours. Then samples were removed from solvent and dried at 40 °C, and weighted to calculate gel contents by using eqn (4) and (5).
|
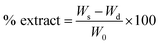 | (4) |
|
% gel content = 100 − % extract
| (5) |
where
Ws is weight of sample and
Wd is weight of dried sample.
Water absorption capacity was determined by a method reported in literature.38 Weighed PU samples were dipped in deionized water for 48 hours. At regular time intervals samples were removed form water, dried and weighed to determine water absorption by eqn (6).15
|
 | (6) |
where
Wt = weight of sample after dipping,
W0 = weight of dried sample.
Hemolytic activity, antioxidant activity and antibacterial activity of synthesized PU composites have been determined by reported methods39–41 using eqn (7) and (8), respectively.
|
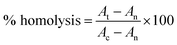 | (7) |
where
At is the absorbance of the test sample.
An is the absorbance of the control (saline control)
Ac is the absorbance of the control (Triton control).
|
 | (8) |
where;
Ablank is the absorbance of the control reaction (containing all reagents except the extract) and
Asample is the absorbance of the mixture containing the extract.
3.1 Shape memory test
Rectangular strips of PU films having dimension 3 cm × 6 mm × 3 mm (Fig. 1) were used to study shape memory behavior (Fig. 1).
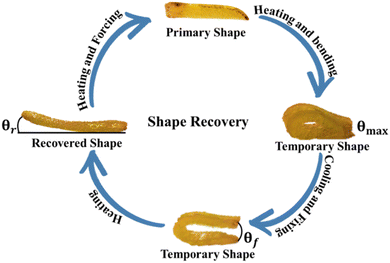 |
| Fig. 1 Shape memory bending test. | |
For shape memory study, films were heated at 54 °C, bent and cooled to maintain temporary shape. Thermal stimulus (50–60 °C) was provided to films for restoration of original shape. The displacement of shape from θmax while cooling and fixing is known as angle of fixity (θf). Attained position by bent film while displacing towards original shape after heating is known as recovered shape and the difference of angle from starting position is known as angle of recovery (θr). Cycles of conversion original → temporary → original shape were repeated five times. Ability of PU films to gain temporary shape and restoration to original shape under the influence of temperature is the shape recovery rate (Rr) and shape fixity rate (Rf), calculated with eqn (9) and (10).15
|
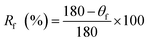 | (9) |
|
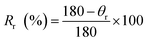 | (10) |
4 Results and discussions
4.1 Physico-chemical analyses
Molecular mass (MM), iodine value (IV), epoxy value (EV) and density of unmodified and modified SSO were calculated to confirm the effectiveness of modification protocol. Upsurge in MM and density of modified samples in comparison to unmodified sample, confirmed the change in molecular assembly. IV difference signposted the effective consumption of unsaturated contents during two step modification. EV counter-confirmed the effectiveness of two-step modification protocol (i.e. epoxidation and hydroxylation) (Table 1).
Table 1 Characteristic data of samples
Sample ID |
MM (g mol−1) |
IV |
EV |
Density (g cm−3) |
SSO |
1103.8 |
129.19 |
104.76 |
0.9130 |
ESSO |
1290.3 |
114.20 |
131.10 |
0.9523 |
HSSO |
1322.6 |
100.66 |
104.90 |
1.0889 |
4.2 FTIR analyses
SSO to ESSO modification was studied by FTIR data (Fig. 2). SSO spectra showed peaks of different functional groups (e.g. C
C, C–O–C and
C–H) at 723 cm−1, 1242 cm−1 and 3008 cm−1 respectively.42,43 After treatment, ESSO spectra showed a reduction in C
C peak with peak area condensed thrice, whereas, absorbance peak of C–O–C became sharp and wide with peak area almost doubled. At the same time, epoxy peak with peak area 0.62 was observed at 826 cm−1 in ESSO spectral line.42 Disappearance of
C–H peak (3008 cm−1)43 in ESSO, may be attributed to conversion of alkene into epoxy, confirmed by the appearance of epoxy peak in ESSO. The peaks present at 2347 cm−1 and 1738 cm−1 represent carbonyl functional group.43 FTIR spectra of HSSO (Fig. 2) obtained after treating ESSO with organic acids (acetic acid and formic acid) in ethanol and water, showed a reduction in C–O–C peak at 1242 cm−1 and also in epoxy peak at 826 cm−1. Moreover, –OH peak appeared at 3502 cm−1.43 The conformation of OH induction may be done with C–O peak formation at 1037 cm−1.43
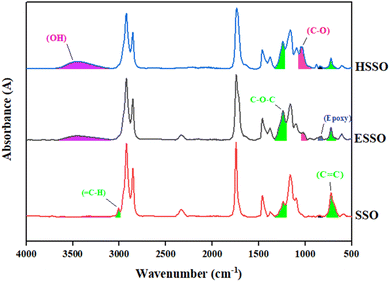 |
| Fig. 2 FTIR of SSO, ESSO and HSSO. | |
HSSO, as biobased diol, upon reaction with TDI resulted in HSSO-PU (Fig. 3). Peaks at 3502 cm−1 and 2245 cm−1 in HSSO FTIR spectra, represent OH in HSSO and NCO in TDI, respectively.33 Upon reaction of HSSO with TDI, disappearance of both OH and NCO peaks in product (Fig. 3) confirmed the consumption of these functional groups along with the formation of a new functional group C–O at 1054 cm−1.43 Breakdown of C
N bond in TDI, resulted in new bonds, i.e. C–N with peak at 1219 cm−1,33 N–H with peak at 3333 cm−1,33 and carbonyl with peak at 1738 cm−1.43 Peak at 1533 cm−1 indicated the presence of cyclic alkenes.33
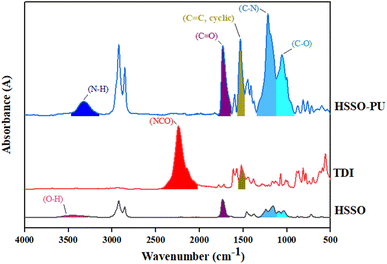 |
| Fig. 3 FTIR of HSSO-PU and its reactants. | |
Or–HSSO-PU sample along with ingredients was analyzed through FTIR (Fig. 4). Peaks of NCO and OH were found at 2245 cm−1 and 1328 cm−1, respectively,15,33 while HSSO hydroxyl group was observed at 3502 cm−1.33 After reaction of orcein and HSSO with TDI, both NCO and OH peaks disappeared rising new peaks in product. It is assumed that polyols OH reacted with C
N in TDI, resulting in CO bond between TDI and polyol with peak at 1054 cm−1.43 Similarly, N linked with H and peak appeared at 3299 cm−1.15 Likewise, breakup of double bond between C and N resulted in single bond left with peak at 1219 cm−1.15,33,43 Carbonyl peak at 1703 cm−1, also increased in size. The peak of cyclic C
C appeared at 1533 cm−1.
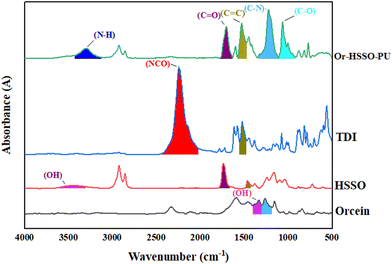 |
| Fig. 4 FTIR of Or–HSSO-PU and its reactants. | |
Similarly, FTIR analysis was applied on Or-PU and its reactants to observe different functional group formed (Fig. 5). Phenolic OH group was observed at 1328 cm−1. After reaction between polyol and isocyanate, NCO and OH functionalities disappeared with new group formation in product, i.e. N–C, C–O and N–H. Peaks of NCO and OH were at 2254 cm−1 and 1328 cm−1 respectively.15,33,43 Appearance of these new peaks in Or-PU confirmed the synthesis of product as shown in Fig. 5.
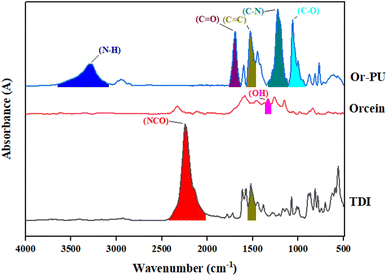 |
| Fig. 5 FTIR analysis of Or-PU and its reactants. | |
4.3 TGA
Thermal stability of prepared samples has been studied with TGA (Fig. 6). Data revealed that incorporation of orcein imparts thermal instability into samples, which might be attributed to higher oxidizable contents on its surface. Thermal degradation of sample prepared from biobased material was observed least in first two segments, which might be attributed to least oxidizable contents on its surface. In third segment (above 400 °C) thermal stability pattern reversed i.e. biobased polyol containing PU degraded quickly in comparison to samples having orcein as polyol. This reversal in degradation pattern may be attributed to fact that orcein based PU samples lost most of oxidizable components in first two segments and became thermally stable whereas biobased polyol containing PU, at 450 °C (Fig. 6), decomposed carbon chains into smaller fragments, prone to thermal degradation.44
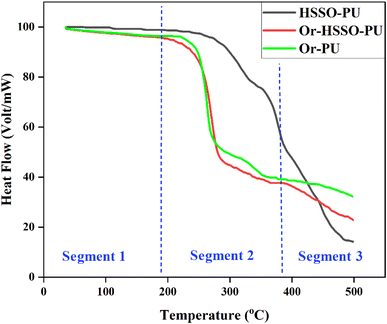 |
| Fig. 6 TGA of PU composites. | |
4.4 DSC
Prepared PU samples were analyzed through DSC (Fig. 7, Table 2). Comparative analysis, on the basis of increasing biobased polyol component, showed an augmentation trend in Tg of hard segment (TDI), signposting micro-phasic separation of hard and soft segments of PU samples by adding HSSO.45 About 100 °C increase in thermal melt of HSSO-PU than Or-PU is due to its well-defined microcrystalline structure which signpost increased cross linking density of rubbery soft segments (polyols), moreover 10 cal. low ΔHm of HSSO-PU than Or-PU represents less thermal dissipation of amorphous HSSO.46 This data evidenced a vivid micro-phasic level separation of hard and soft segments in HSSO-PU.
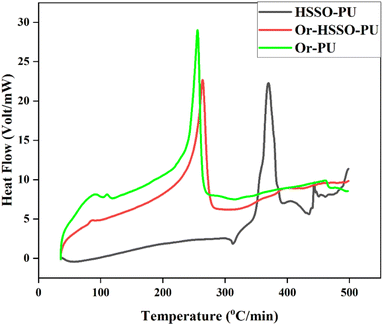 |
| Fig. 7 DSC of PU composites. | |
Table 2 DSC data of prepared PU samples
Sample ID |
Tg,HS (°C) |
Thermal melt |
Temperature (°C) |
ΔHm (cal.) |
HSSO-PU |
322.8 |
369.6 |
132.5 |
Or–HSSO-PU |
88.6 |
264.4 |
136.4 |
Or-PU |
90.4 |
255.6 |
141.9 |
Thermal melt enthalpy increases with increasing polyol content in PU structure.45 Or-PU showed highest ΔHm of all samples indicating highest alcoholic content involvement in polymerization, which might be attributed to condensed structure of orcein, but high thermal dissipation capacity of orcein decreased thermal melt temperature of Or-PU than HSSO-PU.
4.5 Gel content & water absorption capacity
Gel content analysis was applied on prepared PU samples (Table 3). HSSO-PU was found to have the highest gel contents (99.27%) in comparison to Or-PU (98.32%) and Or–HSSO-PU (97.66%) verifying huge quantity of constituents to be crosslinked during synthesis of PU samples.
Table 3 Gel content (%) and water absorption capacity (%) of PU samples
Samples |
Gel content (%) |
Water absorption capacity (%) |
0 h |
1 h |
2 h |
3 h |
4 h |
24 h |
48 h |
Dissolution was observed for a small portion of sample. |
HSSO-PU |
99.27 |
0 |
3.9 |
4.09 |
4.09 |
9.42 |
9.71 |
14.94 |
Or–HSSO-PU |
97.66 |
0 |
0.47 |
1.42 |
4.42 |
3.63a |
7.4a |
5.53a |
Or-PU |
98.32 |
0 |
3.14 |
5.45 |
6.7 |
7.54 |
10.06 |
15.01 |
Water absorption capacity of prepared PU samples was also studied to confirm their hydrophilic nature and biodegradation behavior. Water absorption results (Table 3) indicated an upsurge with temperature rise, which links soft segment's interaction with water. Swelling of material relates inversely with crosslinking density of the constituents, i.e. Or-PU composite had high swelling proportion as compared to HSSO-PU composite due to low crosslinking density.
4.6 Morphology
Microscopic images of HSSO-PU, Or–HSSO-PU and Or-PU (Fig. 8) presented segmental separation of soft and hard components in HSSO-PU, whereas Or-PU showed a homogeneous dispersion of orcein throughout PU sample. Segmental separation type configuration in HSSO-PU has been supported by DSC thermal melt data, which specified well-defined microcrystalline hard segments.
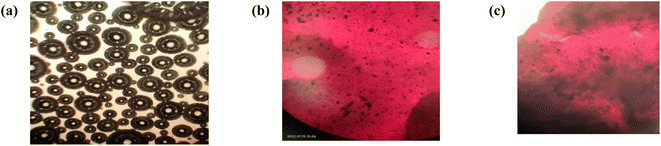 |
| Fig. 8 Microscopic images of (a) HSSO-PU (b) Or–HSSO-PU (c) Or-PU. | |
4.7 SEM and AFM
SEM and AFM analyses of prepared samples (Fig. 9) at μ level revealed homogeneity and smoothness in sample with biobased plasticizer (HSSO). On the contrary, orcein amalgamation imparted non-homogeneity and surface roughness (almost 40 folds to biobased plasticizer), which might be considered for the structural damage during shape memory studies.
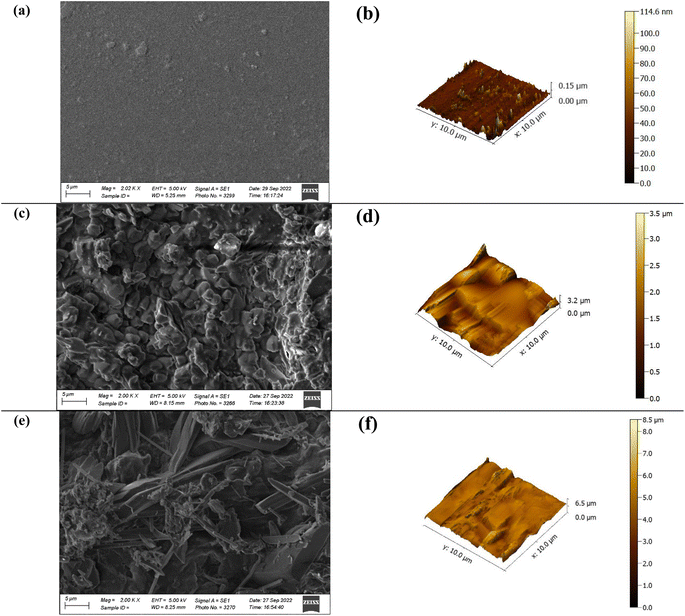 |
| Fig. 9 SEM and AFM diagrams of (a and b) HSSO-PU, (c and d) Or–HSSO-PU, (e and f) Or-PU. | |
4.8 Bioactivities
Hemolytic activity showed mild toxicity at 12 mg ml−1 concentration, which shrank with concentration drop (Table 4). The highest was shown by Or-PU at 14.2 ± 0.14 while the lowest was by HSSO-PU at 12.3 ± 0.17 at 12 mg ml−1 concentration. Likewise, antioxidant potential of HSSO-PU has been observed at a high antioxidant value of 75.34 ± 0.79 followed by Or–HSSO-PU and Or-PU that is 74.5 ± 0.77 and 69.22 ± 0.69, respectively.
Table 4 Toxicity of PU composites at different concentrations
Concentration (mg ml−1) |
Toxicity |
Antioxidant activity |
HSSO-PU |
Or–HSSO-PU |
Or-PU |
Triton X-100 |
HSSO-PU |
Or–HSSO-PU |
Or-PU |
Ascorbic acid |
12 |
12.3 ± 0.17 |
13.2 ± 0.11 |
14.2 ± 0.14 |
97.5 ± 1.8 |
75.34 ± 0.79 |
74.5 ± 0.77 |
69.22 ± 0.69 |
95.93 ± 1.5 |
6 |
10 ± 0.1 |
10.8 ± 0.09 |
11.4 ± 0.09 |
|
71.72 ± 0.7 |
70.1 ± 0.71 |
66.43 ± 0.58 |
90.62 ± 1.1 |
3 |
7.9 ± 0.08 |
8.7 ± 0.06 |
9.1 ± 0.07 |
|
63.09 ± 0.61 |
68.4 ± 0.66 |
55.57 ± 0.51 |
88.71 ± 0.99 |
1.5 |
3.8 ± 0.05 |
4.6 ± 0.06 |
7.8 ± 0.04 |
|
55.84 ± 0.48 |
65.6 ± 0.55 |
54.03 ± 0.43 |
85.22 ± 0.83 |
0.75 |
1.8 ± 0.03 |
2.8 ± 0.02 |
3.7 ± 0.03 |
|
53.06 ± 0.4 |
63.3 ± 0.51 |
46.23 ± 0.33 |
80.29 ± 0.78 |
4.9 Antibacterial activity
Antibacterial activity of samples showed that E. coli is sensitive bacteria against samples that show maximum zone of inhibition in HSSO-PU and Or-PU, while B. subtilis and S. aureus also show a zone of inhibition against HSSO-PU and Or–HSSO-PU. The antibacterial activity of HSSO-PU, Or–HSSO-PU, and Or-PU at sample concentration of 12 mg ml−1 was observed to be 1.8, 1.1, 1.1 for E. coli, 2.8, 1.7 and 1.7 for B. subtilis and 1.9, 2.4 and 1 for S. aureus, respectively. For 6 mg ml−1 sample concentration, the antibacterial activities of HSSO-PU, Or–HSSO-PU, and Or-PU were 1.1, 0 and 0.5 for E. coli, 2, 0 and 1.2 for B. subtilis, and 1.2, 0 and 0 for S. aureus, respectively. While HSSO-PU exhibits bacterial resistance behaviour with 0.7, 0.5, and 0.3 antibacterial activity for E. coli for lower sample concentrations (3, 2.5, and 0.75 mg ml−1). However, other samples with lower sample concentrations failed to exhibit antibacterial efficacy against referred bacterial species.
4.10 Shape memory
Shape memory of PU samples (HSSO-PU, Or–HSSO-PU and Or-PU) was studied under different temperatures (Fig. 10). Or–HSSO-PU and Or-PU showed rigidity till 40 °C and broke but above 40 °C started melting and did not show any shape recovery behavior. This may be attributed to strong thermal dissipation behavior of orcein, which caused melting of sample at a specific temperature through structural damage. On the other side, HSSO-PU started showing flexibility at 50 °C. Shape memory and shape fixing behaviors of HSSO-PU films were studied in temperature range of 50–60 °C (Table 5). Fast shape recovery was found with temperature rise but with more shape fixing, which may be attributed to structural damages of HSSO-PU at 60 °C (Fig. 11).
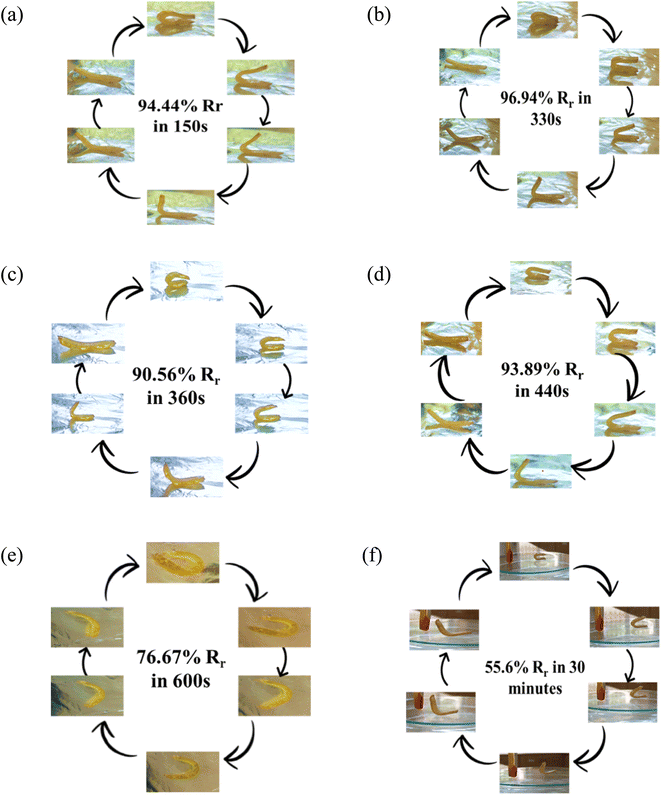 |
| Fig. 10 Shape memory study of HSSO-PU at (a) 60 °C, (b) 55 °C, (c) 54 °C, (d) 52 °C, (e) 51 °C, (f) 40 °C. | |
Table 5 Shape recovery behavior of HSSO-PU at different temperatures
Sample ID |
Temperature (°C) |
Recovery time (s) |
Shape recovery rate% (Rr) |
Ref. |
HSSO-PU |
60 |
150 |
94.44 |
This work |
55 |
330 |
96.94 |
54 |
360 |
90.56 |
52 |
440 |
93.89 |
51 |
600 |
76.67 |
40 |
30 min |
55.6 |
SMPUs |
35–45 |
NR |
98 |
47 |
PU/PEEAMA |
60 |
5 |
96 |
48 |
Table 6 Composition and Rr time comparison of body temperature sensitive PU
Sr. no. |
Polyol |
Isocyanate |
Catalyst |
Chain extender |
Rr time (seconds) |
Ref. |
1 |
Co-PLAols |
IPDI |
SnOct2 |
BDO, HDO, EG |
60 |
25 |
2 |
PCL diol |
MDI-50 |
SnOct2 |
N/R |
15 |
31 |
3 |
Co-PLA tetraol |
HDI |
SnOct2 |
PETP |
101 |
32 |
4 |
HSSO |
TDI |
N/A |
N/A |
30 min |
This work |
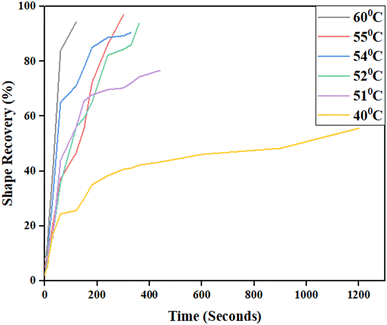 |
| Fig. 11 Shape recovery graph of HSSO-PU composite at different temperatures. | |
Biodegradability and water uptake response of PU samples containing HSSO (Table 3) has widened the application scope of these materials in textile as well as biomedical not only as drug carrier but also as shape recovery materials. To recognize shape memory response of HSSO-PU at human body temperature, shape recovery at 36 °C was studied (Fig. 12). 52.78% shape recovery was found without any force whereas 85% shape recovery was observed at 93.1196 N force.
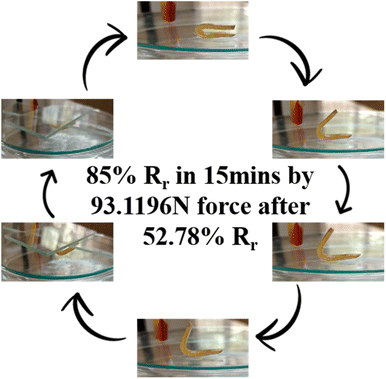 |
| Fig. 12 Shape memory study of HSSO-PU at 36 °C. | |
Upon comparison of shape recovery data from already published works, it is being claimed that current research has neither used any types of chain extenders nor catalyst to support shape recovery rate at body temperature, furthermore, not any specially designed commercial product to impart flexibility (MDI-50) has been incorporated in molecular design. This outcome i.e. synthesis without special chemical, will support HSSO-PU's expanded biomedical application domain.
5 Conclusion
In this research biobased polyols have been designed through an eco-friendly and facile approach. SSO has been treated to obtain hydroxylated plasticizer (HSSO), which has induced low thermal actuation shape recovery with high thermal stability in PU samples, whereas orcein has imparted rigidity and easy thermal degradation. HSSO has framed separate and well-defined hard and soft segments in PU. Prepared samples have 100% thermal stability till 270 °C, but shape recovery response initiated at very low thermal stimulus i.e. 36 °C, which has made it a suitable candidate for self-tightening structures not only in textiles and biomedical applications like dental implants, but also in all applications that require shape recovery at very low temperature.
Conflicts of interest
There are no conflicts to declare.
References
- W. M. Huang and et al, ., Shape memory materials, Mater. Today, 2010, 13(7), 54–61 CrossRef CAS.
- C. Liu and et al, ., Magnetic mesoporous silica microspheres with thermo-sensitive polymer shell for controlled drug release, J. Mater. Chem., 2009, 19(27), 4764–4770 RSC.
- P. Pan and et al, ., Photosensitive drug delivery systems for cancer therapy: Mechanisms and applications, J. Controlled Release, 2021, 338, 446–461 CrossRef CAS PubMed.
- M. Gosecka and M. Gosecki, Chemoresponsive polymer systems for selective molecular recognition of organic molecules in biological systems, Acta Biomater., 2020, 116, 32–66 CrossRef CAS PubMed.
- F. Ofridam and et al, ., pH-sensitive polymers: Classification and some fine potential applications, Polym. Adv. Technol., 2021, 32(4), 1455–1484 CrossRef CAS.
- Y. Li and et al, ., 4D printed shape memory polymers and their structures for biomedical applications, Sci. China: Technol. Sci., 2020, 63(4), 545–560 CrossRef.
- J. Delaey, P. Dubruel and S. Van Vlierberghe, Shape-memory polymers for biomedical applications, Adv. Funct. Mater., 2020, 30(44), 1909047 CrossRef CAS.
- V. C. Sonawane and et al, ., Fabrication and characterization of shape memory polymers based bioabsorbable biomedical drug eluting stent, Artif. Cells, Nanomed., Biotechnol., 2017, 45(8), 1740–1750 CrossRef CAS PubMed.
- W. Zhao and et al, ., Shape memory polymers and their composites in biomedical applications, Mater. Sci. Eng., C, 2019, 97, 864–883 CrossRef CAS PubMed.
- M. Zare and et al, ., Highly porous 3D sponge-like shape memory polymer for tissue engineering application with remote actuation potential, Compos. Sci. Technol., 2019, 184, 107874 CrossRef CAS.
- X. Cheng and et al, ., Enhanced biocompatibility of polyurethane-type shape memory polymers modified by plasma immersion ion implantation treatment and collagen coating: an in vivo study, Mater. Sci. Eng., C, 2019, 99, 863–874 CrossRef CAS PubMed.
- D. J. Maitland and et al, ., Photothermal properties of shape memory polymer micro-actuators for treating stroke, Lasers Surg. Med., 2002, 30(1), 1–11 CrossRef PubMed.
- S. Thakur and J. Hu, Polyurethane: a shape memory polymer (SMP), Aspects of polyurethanes, 2017, pp. 53–71 Search PubMed.
- P. Ping and et al, ., Shape-memory and biocompatibility properties of segmented polyurethanes based on poly (L-lactide), Frontiers of Chemistry in China, 2007, 2(4), 331–336 CrossRef.
- S. S. Khasraghi, A. Shojaei and U. Sundararaj, Bio-based UV curable polyurethane acrylate: Morphology and shape memory behaviors, Eur. Polym. J., 2019, 118, 514–527 CrossRef CAS.
- A. Lendlein and S. Kelch, Shape-Memory Polymers, Angew. Chem., Int. Ed., 2002, 41(12), 2034–2057 CrossRef CAS.
- H. Xie, K.-K. Yang and Y.-Z. Wang, Photo-cross-linking: a powerful and versatile strategy to develop shape-memory polymers, Prog. Polym. Sci., 2019, 95, 32–64 CrossRef CAS.
- H. S. El-Sheshtawy and et al, ., Eco-friendly polyurethane acrylate (PUA)/natural filler-based composite as an antifouling product for marine coating, Appl. Microbiol. Biotechnol., 2021, 105(18), 7023–7034 CrossRef CAS PubMed.
- T. Tsujimoto, T. Takayama and H. Uyama, Biodegradable shape memory polymeric material from epoxidized soybean oil and polycaprolactone, Polymers, 2015, 7(10), 2165–2174 CrossRef CAS.
- J. Lu and et al, ., Self-healable castor oil-based waterborne polyurethane/MXene film with outstanding electromagnetic interference shielding effectiveness and excellent shape memory performance, J. Colloid Interface Sci., 2021, 588, 164–174 CrossRef CAS PubMed.
- S. Nicolas and et al, ., Shape memory epoxy vitrimers based on waste frying sunflower oil, J. Appl. Polym. Sci., 2021, 138(36), 50904 CrossRef CAS.
- L. L. Taung Mai and et al, ., Non edible oil-based epoxy resins from Jatropha oil and their shape memory behaviors, Polymers, 2021, 13(13), 2177 CrossRef CAS PubMed.
- N. Trinh and et al, ., The Effect of Polyol Types and Molecular Weight on the Shape Memory Properties of Palm Kernel Oil–based Polyurethane, Mater. Today: Proc., 2019, 17, 898–904 CAS.
- L. Zhang and et al, ., Bio-based shape memory polyurethanes (Bio-SMPUs) with short side chains in the soft segment, J. Mater. Chem. A, 2014, 2(29), 11490–11498 RSC.
- L. Gu and et al, ., Bio-based polyurethanes with shape memory behavior at body temperature: Effect of different chain extenders, RSC Adv., 2016, 6(22), 17888–17895 RSC.
- L. Boton and et al, ., Synthesis and properties of quick-drying UV-curable hyperbranched waterborne polyurethane coating, Prog. Org. Coat., 2018, 125, 201–206 CrossRef CAS.
- S. Kumar and et al, ., Biodegradable hybrid nanocomposite of chitosan/gelatin and green synthesized zinc oxide nanoparticles for food packaging, Foods, 2020, 9(9), 1143 CrossRef CAS PubMed.
- S. S. Suryawanshi and et al, ., Bioconversion of sugarcane molasses into bioplastic (polyhydroxybutyrate) using Bacillus cereus 2156 under statistically optimized culture conditions, Anal. Chem. Lett., 2020, 10(1), 80–92 CrossRef CAS.
- S. Arasaretnam, Preparation of Biobased Plastic from Banana Peel and Application in Industrial Waste Water Purification, in Conference Proceedings of the 2nd Asia International Conference on Multidisciplinary, 2020 Search PubMed.
- L. Zhang and et al, ., Highly recoverable rosin-based shape memory polyurethanes, J. Mater. Chem. A, 2013, 1(10), 3263–3267 RSC.
- H.-M. Dou and et al, ., Bio-based, biodegradable and amorphous polyurethanes with shape memory behavior at body temperature, RSC Adv., 2019, 9(23), 13104–13111 RSC.
- S. Shi and et al, ., Bio-based (co) polylactide-urethane networks with shape memory behavior at body temperature, RSC Adv., 2016, 6(83), 79268–79274 RSC.
- N. T. Thanh, Study on effects of isocyanate on some properties of epoxy varnish, J. Chem., 2022, 60(1), 15–20 CAS.
- N. Peamaroon, J. Jakmunee and N. Moonrungsee, A Simple Colorimetric Procedure for the Determination of Iodine Value of Vegetable Oils Using a Smartphone Camera, J. Anal. Test., 2021, 5(4), 379–386 CrossRef.
- Z. He and et al, ., Ultrasonication-assisted rapid determination of epoxide values in polymer mixtures containing epoxy resin, Anal. Methods, 2014, 6(12), 4257–4261 RSC.
- A. K. Bryan and et al, ., Measurement of mass, density, and volume during the cell cycle of yeast, Proc. Natl. Acad. Sci. U. S. A., 2010, 107(3), 999–1004 CrossRef CAS PubMed.
- M. Atif and et al, ., Effect of novel UV-curing approach on thermo-mechanical properties of colored epoxy composites in outsized dimensions, J. Compos. Mater., 2016, 50(22), 3147–3156 CrossRef CAS.
- H. Daud and et al, ., Preparation and characterization of guar gum based biopolymeric hydrogels for controlled release of antihypertensive drug, Arabian J. Chem., 2021, 14(5), 103111 CrossRef CAS.
- G. Kumar, L. Karthik and K. V. B. Rao, Hemolytic activity of Indian medicinal plants towards human erythrocytes: an in vitro study, Elixir Applied Botany, 2011, 40(5534), e5537 Search PubMed.
- A. N.-A. Amr and et al, ., Anti-cancer and anti-oxidant activity of some Egyptian medicinal plants, J. Med. Plants Res., 2009, 3(10), 799–808 Search PubMed.
- A. Ramjan and et al, ., Evaluation of thrombolytic potential of three medicinal plants available in Bangladesh, as a potent source of thrombolytic compounds, Avicenna J. Phytomed., 2014, 4(6), 430–436 Search PubMed.
- B. Smith, The Infrared Spectra of Polymers V: Epoxies, Spectroscopy, 2022, 37(3), 17–19 Search PubMed.
- Sigma-Aldrich, IR Spectraum Table and Chart, 2022, available from, https://www.sigmaaldrich.com/PK/en/technical-documents/technical-article/analytical-chemistry/photometry-and-reflectometry/ir-spectrum-table Search PubMed.
- M. Atif and et al, ., Electrochemical Evaluation of Human Hair Derived Carbon Particles, ECS J. Solid State Sci. Technol., 2020, 9(5), 051003 CrossRef CAS.
- P. Somdee and et al, ., Thermal analysis of polyurethane elastomers matrix with different chain extender contents for thermal conductive application, J. Therm. Anal. Calorim., 2019, 138(2), 1003–1010 CrossRef CAS.
- L. M. Leung and J. T. Koberstein, DSC annealing study of microphase separation and multiple endothermic behavior in polyether-based polyurethane block copolymers, Macromolecules, 1986, 19(3), 706–713 CrossRef CAS.
- M. Ahmad and et al, ., Synthesis and characterization of polyurethane-based shape-memory polymers for tailored Tg around body temperature for medical applications, Macromol. Chem. Phys., 2011, 212(6), 592–602 CrossRef CAS.
- A. Kausar and A. Ur Rahman, Effect of graphene nanoplatelet addition on properties of thermo-responsive shape memory polyurethane-based nanocomposite, Fullerenes, Nanotubes, Carbon Nanostruct., 2016, 24(4), 235–242 CrossRef CAS.
|
This journal is © The Royal Society of Chemistry 2023 |