DOI:
10.1039/D3QO00579H
(Research Article)
Org. Chem. Front., 2023,
10, 2752-2759
Base-catalyzed addition of silylacetylenes to ketones: a route to protected tertiary propargyl alcohols†
Received
18th April 2023
, Accepted 7th May 2023
First published on 9th May 2023
Abstract
The base-catalyzed addition of alkynylsilanes to ketone derivatives enables the formation of various silyl-protected propargylic alcohols. Commercially available and inexpensive potassium bis(trimethylsilyl)amide (KHMDS) serves as an efficient transition metal-free catalyst and permits the functionalization of a variety of derivatives, including pharmaceuticals and biorelevant compounds. Overall, the presented system complements classical routes to protected tertiary propargylic alcohols that mainly rely on stoichiometric processes or fluoride-mediated reactions.
Introduction
Propargyl alcohols are ubiquitous building blocks in chemical synthesis, being highly valued for their widespread application as valuable synthetic intermediates or medicinally relevant molecules themselves.1–15 They participate in a diverse array of transformations, owing this to their multifunctionality, and thereby being the subject of numerous studies. Protected propargyl alcohols can be accessed via well-developed stoichiometric methods, including mainly the use of a combination of propargyl alcohol, chlorosilane and organolithium reagent (Fig. 1).16 Such approaches are not without some disadvantages, including the need for an excess of both corrosive halosilane and hazardous n-butyllithium, little accessibility to commercial propargyl alcohol derivatives (compared with ubiquitous ketones), and the purification requiring high amounts of solvents (column chromatography). Moreover, the final isolating yields could be improved. For example, the reaction of 1-diphenyl-2-propyn-1-ol (Merck – 25 g per 139 €) gave the desired product in 81% yield, whereas our methodology using benzophenone as the starting reagent (Merck – 25 g per 11 €) led to identical product 3t in 97% yield. Furthermore, an analogous stoichiometric reaction utilizing ketones requires a two-step pathway (the reaction with in situ generated (trimethylsilyl)acetylide followed by the subsequent O-silylation).17 On the other hand, the concept of using silylacetylenes and ketones as the substrates has also been studied in the presence of fluorine reagents (e.g., tetrabutylammonium fluoride – TBAF,18–20 cesium fluoride – CsF,21etc.22,23). However, also these strategies have several drawbacks including lower selectivity and efficiency (formation of byproducts19,21,22 and moderate yields19,21,24,25), the need for expensive crown ethers (CsF),21 and narrow substrate scope.
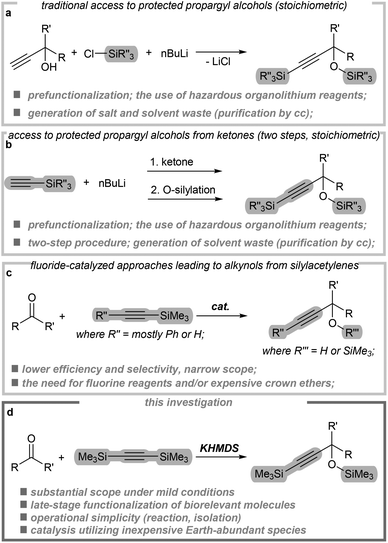 |
| Fig. 1 Context of the investigation. | |
In this context, it is also worth noting the classical alkynylation of carbonyl compounds for the generation of unprotected propargylic alcohols, originally discovered by Alexei Favorskii in the early 1900s.26 Here, besides stoichiometric approaches, several catalytic protocols were developed as well.27–37
The application of Earth-abundant species as catalysts have gained recent significant attention in organic chemistry.38–49 Considering our recent success in activating organometalloids under sustainable catalysis,50–56 we reasoned that an appropriate catalytic manifold could provide an efficient platform to generate diversified libraries of protected propargyl alcohols. To address the limitations of previously mentioned methods, herein we report the KHMDS-catalyzed addition of silylacetylenes to ketones. Examples of biorelevant molecules transformed to the corresponding protected alkynols include camphor (topical medication), (R)-(−)-carvone (food additive), nabumetone (nonsteroidal anti-inflammatory drug), etc. Thereby, the mentioned late-stage functionalization may lead to potentially biologically interesting compounds.
Results and discussion
In optimization studies, summarized in Table 1, we investigated the addition of bis(trimethylsilyl)acetylene to acetophenone.
Table 1 Optimization of addition of silylacetylene 2a to ketone 1a
a

|
Entry |
Variation from standard conditions |
Conversion of 1a a [%] |
Conversion determined via GC, with n-dodecane as internal standard. Isolated yield in parenthesis.
After 2 h.
After 24 h.
Solvent-free cond.
|
1 |
No change |
99 (95) |
2 |
No catalyst |
0c |
3 |
Under solvent-free conditions |
58b |
4 |
1 mmol of 2a |
19 |
5 |
2 mmol of 2a |
90 |
6 |
Trimethylsilylacetylene instead of 2a |
80b |
7 |
1.5 mol% of KHMDS |
60b |
8 |
3 mol% of KOH at 80 °C |
0b |
9 |
3 mol% of t-BuOK at 80 °C |
20b |
10 |
3 mol% of KF at 80 °C |
0b |
11 |
3 mol% of NaHMDS at 80 °C |
0b |
12 |
In toluene |
0 |
13 |
In 1,4-dioxane |
30b |
14 |
Phenylacetylene instead of 2a |
0c,d |
After several attempts, the ideal reaction conditions were identified (Table 1, entry 1), giving product 3a in a 95% isolated yield. Control reactions revealed that the reaction does not proceed without the catalyst (entry 2). Instead, it was found that the reaction still works fine under solvent-free reaction conditions (entry 3). To ensure full conversion of 1a, it is needed to use an excess of 2a (entries 1, 5, and 6). What is more, such an excess of bis(trimethylsilyl)acetylene (BTMSA) excludes the formation of small amounts of diadduct. Please note, that 2a can be readily recovered. For details, please see Fig. 8 (part D). Next, the attempt to replace the silylating source with trimethylsilylacetylene gave an inferior result (entry 7). Further experiments showed that other main-group mediators were mostly inactive under the tested conditions (entries 9–12). Considering the Favorskii reaction, we also checked simple phenylacetylene as the reaction partner with 1a in the presence of KHMDS. However, no product was observed after 2 and 24 h (entry 14).
Next, a variety of different ketones were tested to evaluate the generality and functional-group tolerance of our protocol (Fig. 2). A wide range of 1-substituted ethanones worked efficiently under the reaction conditions (3a–3i). As an initial example 3a, ketones bearing electron-donating alkyl groups were readily converted (3b–3c), as well as phenanthrene derivative 3e (70% yield). Gratifyingly, halogenated acetophenones were readily adopted in this protocol (3g–3j, 76–79% yield), however, they required a higher amount of the catalyst (6 mol%). Moreover, other useful derivatives were also well tolerated, and led to protected alkynols 3k–3m in good to excellent yields (79–99%). Notably, 5-hexen-2-one also participated effectively in this reaction (3n, 75% yield), while preserving the ene-functionality untouched. Encouraged by these results, we then investigated the use of heterocyclic ketones. All of them afforded the expected products in good yields (3o–3p, 62–70%). Subsequently, we tested our methodology on variously substituted propyl ketones. Simple nonan-3-one, as well as propiophenone and its fluoro-substituted derivative reacted well, providing 3q, 3r, and 3s respectively in high yields (70–99%). Gratifyingly, two different benzophenones as well as cyclohexyl ketones were also readily adopted in this protocol (3t–3w, 94–99% yield). Finally, cyclohexanone was also well tolerated, and led to protected alkynol 3x in excellent yield (91%).
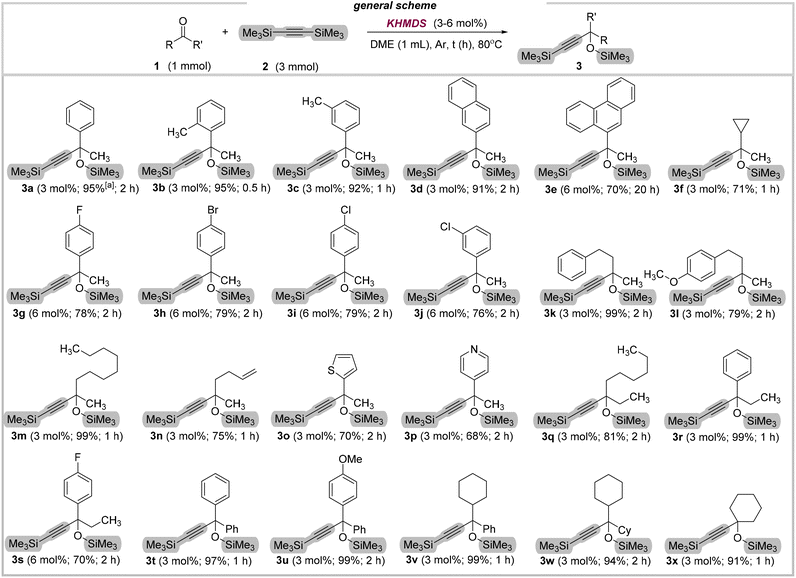 |
| Fig. 2 Substrate scope for the addition of bis(trimethylsilyl)acetylene to ketones in the presence of KHMDS. | |
Having demonstrated the unique robustness and versatility of our strategy, we sought to explore the late-stage functionalization of biorelevant compounds including drugs. Pleasingly then, camphor, (R)-(−)-carvone, and nabumetone were isolated in good to excellent yield (4a–4c; 72–96%; Fig. 3). Intrigued by the high efficiency and selectivity of the transformation, we next pursued the development of further applications of our catalytic system. Encouragingly, this strategy can also be applied to silylacetylenes other than bis(trimethylsilyl)acetylene (2a).
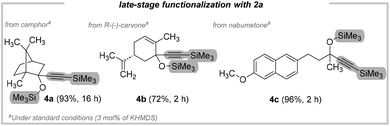 |
| Fig. 3 Late-stage functionalization of biorelevant ketones. | |
As shown in Fig. 4, the addition of 1-phenyl-2-trimethylsilylacetylene (2b), ((2-fluorophenyl)ethynyl)-trimethylsilane (2c), 2-((trimethylsilyl)ethynyl)pyridine (2d), and trimethyl(thiophen-3-ylethynyl)silane (2e) was performed, successfully providing protected alkynols 5a–5j, in very good yields (up to 99%).
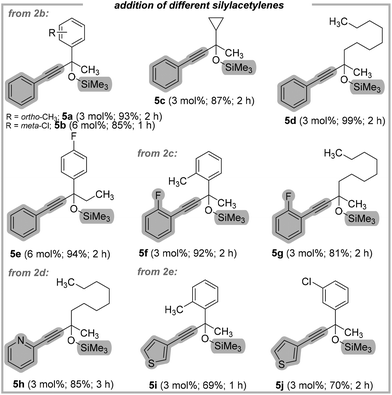 |
| Fig. 4 Substrate scope for the addition of different silylacetylenes to ketones in the presence of KHMDS. | |
With these outcomes in mind, and given the similarity of aldehydes, we hypothesized that the presented addition reaction might allow convenient access to various protected secondary alkynols. In contrast to ketones, the reactions with the corresponding aldehydes were less selective and efficient, and somehow, this is in accordance with TBAF-mediated protocol (Fig. 5).19
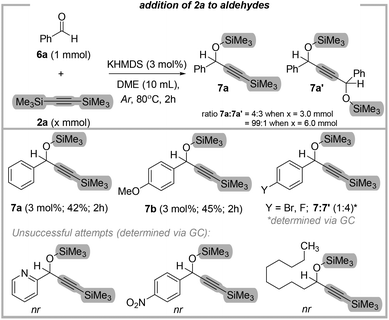 |
| Fig. 5 Initial reaction between aldehydes and BTMSA under standard conditions. | |
For instance, the reaction of 3.0 eq. of 2a with 1.0 eq. of benzaldehyde led to the mixture of mono- and diadduct (4
:
3). Notably, in the case of more sterically hindered ketones, we observed only traces of the above-mentioned diadducts (<1%). Their formation suggests that protected secondary alkynols can serve as competitive substrates toward bis(trimethylsilyl)-acetylene. The same result was obtained for the reaction of 3.0 eq. of 2a with 1.0 eq. of 4-bromobenzaldehyde. Specifically, a mixture of mono- and diadduct was also observed (1
:
4). Considering this, we decided to check the influence of higher amounts of BTMSA.
Indeed, when 6.0 eq. of 2a was used, we observed the corresponding products 7a and 7b exclusively. Unfortunately, the aldehydes with the electron-withdrawing group still gave the mixture of mono- and diadduct. What is more, picolinaldehyde, 4-nitrobenzaldehyde, and decanal were non-reactive. Next, we were wondering that reaction of benzaldehyde with 1-phenyl-2-trimethylsilylacetylene (2b) should resolve the issue of selectivity. Surprisingly, we did not observe any conversion of benzaldehyde. The reason for the observed difference is not apparent at the moment. However, further investigations involving aldehydes and silylacetylenes are ongoing in our laboratory.
The key goals of this study were to simplify the synthesis and isolation of the protected tertiary alkynols, as well as show the scalability of the proposed methodology. Therefore, our protocol was successfully scaled up to a 10 mmol scale yielding 98% (2.84 g) of product 3a (Fig. 6).
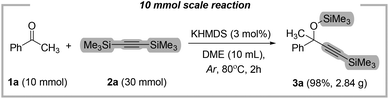 |
| Fig. 6 Scaled up synthesis of 3a. | |
Once the scope of carbonyls was identified, our KHMDS-promoted addition was compared with other established methods leading to protected/unprotected tertiary alkynols (Fig. 7).
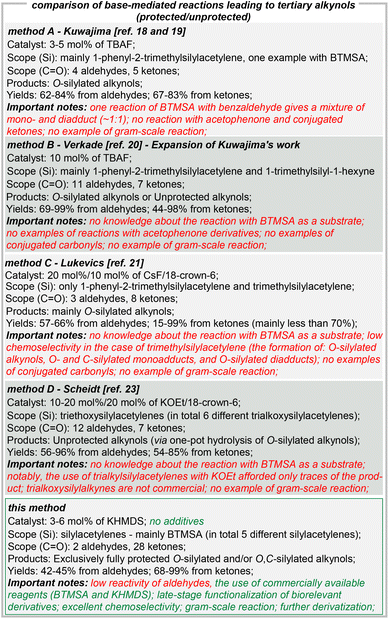 |
| Fig. 7 Applications of varied addition methods leading to protected or unprotected alkynols. | |
Admittedly, all mentioned strategies are not without their own disadvantages. In the case of this protocol, the main drawback is the low reactivity of aldehydes, unlike ketones. But apart from that, several salient benefits of our strategy should be outlined. First of all, this is the first chemoselective method utilizing BTMSA as the substrate – leading exclusively to monoadduct products. There are two important facets. Firstly, bis(trimethylsilyl)acetylene is commercially available and inexpensive. Secondly, its use leads to O- and C-protected alkynols in a one-step procedure. Furthermore, the addition of BTMSA into carbonyls followed by selective deprotection can lead to terminal alkynols. Thus, being an indirect addition of acetylene. The other noteworthy feature of our strategy is the remarkable reactivity of ketones. It enabled the synthesis of a variety of protected tertiary alkynols including biorelevant compounds such as pharmaceuticals. Moreover, this protocol was scaled up to a 10 mmol scale. This once again makes it clear that the proposed methodology has significant application potential. Lastly, considering its operational simplicity, this reaction system provides a sustainable alternative to existing synthetic solutions. Notably, the exclusion of column chromatography leads to savings in many areas (e.g., solvents, silica, time consumption, etc.).
In the end, we turned our attention to further applications of our methodology, as well as its sustainability and mechanistic insights (Fig. 8–10).
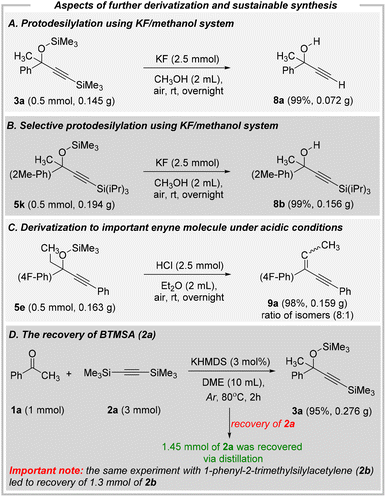 |
| Fig. 8 Potential pathways for derivatizations and rationale for the excess amount of silylacetylene. | |
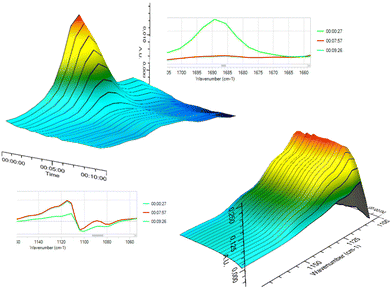 |
| Fig. 9 Illustrations of carbonyl band disappearance (top) recorded using realtime FT-IR spectroscopy during the addition of 2a to 1b at 80 °C. | |
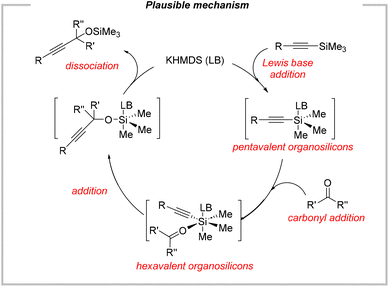 |
| Fig. 10 Plausible mechanism for KHMDS-catalyzed addition of silylacetylenes to carbonyls. | |
The KF/methanol system usually results in the deprotection of trimethylsilyl moiety, while the bulky triisopropylsilyl group remains untouched. By subjecting 2.5 mmol of potassium fluoride to the solution of 3a (Fig. 8A) or 5k (Fig. 8B) in methanol, the quantitative amounts of unprotected alkynol 8a and C-protected alkynol 8b were isolated. We could then successfully transform the silyl-protected alkynols into different products, thus showing the possibility to synthesize more complex products with challenging substitution patterns. Furthermore, the broader utility of our strategy is highlighted by the facile conversion of previously obtained O-silylated alkynol 5e to a highly important enyne derivative (Fig. 8C). To the best of our knowledge, this is the first example of direct elimination of –OTMS group from alkynols leading to enynes (please note that recently, Vasilyev reported on similar acid-mediated elimination of TMS ethers of CF3-benzyl alcohols).57 To date, unprotected alkynols were generally converted to enynes by using methanesulfonyl chloride/triethylamine system. Lastly, our initial idea was to deliver a sustainable synthetic protocol. Thus, an excess of silylacetylene (mainly 2a) could be regarded as a significant drawback of our methodology. Fortunately, this issue should not be considered problematic because bis(trimethylsilyl)acetylene (as well as 1-phenyl-2-trimethylsilylacetylene) can be readily recovered at the isolation stage (Fig. 8D).
Next, to get some mechanistic insights into this base catalysis, we conducted preliminary experiments. For instance, the addition was performed in the presence of a typical radical scavenger such as TEMPO (100 mol%), giving the desired product (with almost the same efficiency), thereby implying that radical pathways were likely not operative. Moreover, we monitored the reaction system using real-time in situ FT-IR spectroscopy. The kinetic plots obtained for the addition of BTMSA (2a) to 2-methylacetophenone (1b) confirmed a rapid disappearance of the distinguishing band at 1689 cm−1 of the carbonyl moiety (Fig. 9, top) with the simultaneously increased intensity of the Si–O band at 1115 cm−1 (Fig. 9, bottom).
Previously, Scheidt presented very convincing evidence for hypervalent silicon intermediates resulting from the reversible addition of potassium ethoxide. Considering this, we performed 29Si NMR analysis of the mixture containing stoichiometric amounts of BTMSA and KHMDS (ratio 1
:
1) in acetonitrile-d3. In this particular experiment, we still observe the signal corresponding to BTMSA (−19.2 ppm, lit. val. = −19.2 ppm in CDCl3). Moreover, two additional signals appeared at −17.3 ppm and 7.5 ppm. The former could be assigned to trimethylsilylacetylene. However, 1H NMR analysis excluded such a situation (no signal for the acetylenic proton, lit. val. = 2.3 ppm (ref. 58)), suggesting more likely the presence of different, hypervalent silylacetylene species. However, in the case of pentacoordinated silicon species it is not so clear. Especially, there is little knowledge concerning pentavalent trialkylsilyl species resulting from the reversible addition of disilazide anion. In general, all the previous protocols for the base-catalyzed addition of silylacetylenes to carbonyls were assuming the intermediacy of hypervalent silicon species. In our specific case, it is also the most probable pathway. Thus, a plausible catalytic cycle is presented in Fig. 10.
Conclusions
In conclusion, the base-catalyzed addition of silylacetylenes to ketones has been enabled using commercially available reagents. The employment of inexpensive potassium bis(trimethylsilyl)amide as the catalyst and bis(trimethylsilyl)-acetylene not only provides a mild and economical alternative to canonical catalysis by fluoride anions, but also represents a highly chemoselective approach with an exceptional scope that is currently absent in other methods. Hence, late-stage derivatization of biorelevant compounds, as well as selective protodesilylation and OTMS elimination, further demonstrated the synthetic potential of the transformation. The scalability and practicality of this reaction were also demonstrated by both gram-scale and recovery experiments. We expect that our strategy will streamline the synthesis of different organosilicons and enable the development of new synthetic pathways involving potassium bis(trimethylsilyl)amide and bis(trimethyl-silyl)acetylene as the reagents.‡
Conflicts of interest
There are no conflicts to declare.
Acknowledgements
This work was supported by a National Science Centre (Poland) Grant UMO-2021/43/D/ST4/00132 (K.K.). This work was also supported by the Adam Mickiewicz University (KK-ID-UB project no. 038/04/NŚ/0001). We owe a very special thanks to Dr Rafał Januszewski (AMU Poznań) for his invaluable assistance involving FT-IR analyses.
References
- S. Jiang, S. Du, R. Yang, F. Jin, Z.-Z. Zhou, W.-F. Tian, X.-R. Song and Q. Xiao, Brønsted Acid Promoted Sulfonylation of Propargylic Alcohols: Synthesis of Triaryl Allenyl Sulfones under Mild Conditions, Eur. J. Org. Chem., 2023, e202201377 CAS.
- A. R. Pandey, D. K. Tiwari, A. Prakhar, D. P. Mishra and S. K. Sharma, A review towards synthesis of heterocycles using propargyl alcohols and propargyl amines, Monatsh. Chem., 2022, 153, 383–407 CrossRef CAS.
- T. A. C. Goulart, A. M. S. Recchi, D. F. Back and G. Zeni, Selective 5-Exo-Dig versus 6-Endo-Dig Cyclization of Benzoimidazole Thiols with Propargyl Alcohols, Adv. Synth. Catal., 2022, 364, 1989–1997 CrossRef CAS.
- S. Mishra, S. R. Nair and B. Baire, Recent approaches for the synthesis of pyridines and (iso)quinolines using propargylic Alcohols, Org. Biomol. Chem., 2022, 20, 6037–6056 RSC.
- H. Zhu, Q. Zhou, N. Liu, J. Xing, W. Yao and X. Dou, Relay Rhodium(I)/Acid Catalysis for Rapid Access to Benzo-2H-Pyrans and Benzofurans, Adv. Synth. Catal., 2022, 364, 1162–1167 CrossRef CAS.
- S. Du, A.-X. Zhou, R. Yang, X.-R. Song and Q. Xiao, Recent advances in the direct transformation of propargylic alcohols to allenes, Org. Chem. Front., 2021, 8, 6760–6782 RSC.
- S. Liu, Y. Tanabe, S. Kuriyama, K. Sakata and Y. Nishibayashi, Ruthenium- and Copper-Catalyzed Propargylic Substitution Reactions of Propargylic Alcohol Derivatives with Hydrazones, Chem. – Eur. J., 2021, 27, 15650–15659 CrossRef CAS PubMed.
- X.-R. Song, R. Yang and Q. Xiao, Recent Advances in the Synthesis of Heterocyclics via Cascade Cyclization of Propargylic Alcohols, Adv. Synth. Catal., 2021, 363, 852–876 CrossRef CAS.
- G. R. Kumar, M. Rajesh, S. Lin and S. Liu, Propargylic Alcohols as Coupling Partners in Transition-Metal-Catalyzed Arene C–H Activation, Adv. Synth. Catal., 2020, 362, 5238–5256 CrossRef CAS.
- U. M. Gonela and J. S. Yadav, Synthesis of chiral propargyl alcohols following the base-induced elimination protocol: application in the total synthesis of natural products, New J. Chem., 2020, 44, 4972–4986 RSC.
- H. Tsuji and M. Kawatsura, Transition-Metal-Catalyzed Propargylic Substitution of Propargylic Alcohol Derivatives Bearing an Internal Alkyne Group, Asian J. Org. Chem., 2020, 9, 1924–1941 CrossRef CAS.
- H. Qian, D. Huang, Y. Bi and G. Yan, 2-Propargyl Alcohols in Organic Synthesis, Adv. Synth. Catal., 2019, 361, 3240–3280 CrossRef CAS.
- R. Roy and S. Saha, Scope and advances in the catalytic propargylic substitution reaction, RSC Adv., 2018, 8, 31129–31193 RSC.
- Y. Miyake, S. Uemura and Y. Nishibayashi, Catalytic Propargylic Substitution Reactions, ChemCatChem, 2009, 1, 342–356 CrossRef CAS.
- R. J. Detz, H. Hiemstra and J. H. van Maarseveen, Catalyzed Propargylic Substitution, Eur. J. Org. Chem., 2009, 6263–6276 CrossRef CAS.
- T. Ishikawa, M. Okano, T. Aikawa and S. Saito, Novel Carbon–Carbon Bond-Forming Reactions Using Carbocations Produced from Substituted Propargyl Silyl Ethers by the Action of TMSOTf, J. Org. Chem., 2001, 66, 4635–4642 CrossRef CAS PubMed.
- T. Ishikawa, T. Aikawa, Y. Mori and S. Saito, Lewis Acid-Catalyzed Nucleophilic Substitutions of Propargylic and Allylic Silyl Ethers with Enol Silyl Ethers, Org. Lett., 2003, 5, 51–54 CrossRef CAS PubMed.
- E. Nakamura and I. Kuwajima, Fluoride Catalyzed Addition of Silylacetylenes to Carbonyl Compounds, Angew. Chem., Int. Ed. Engl., 1976, 15, 498–499 CrossRef.
- I. Kuwajima, E. Nakamura and K. Hashimoto, Fluoride catalyzed reaction of silylacetylenes with carbonyl compounds, Tetrahedron, 1983, 39, 975–982 CrossRef CAS.
- V. R. Chintareddy, K. Wadhwa and J. G. Verkade, Tetrabutylammonium Fluoride (TBAF)-Catalyzed Addition of Substituted Trialkylsilylalkynes to Aldehydes, Ketones, and Trifluoromethyl Ketones, J. Org. Chem., 2011, 76, 4482–4488 CrossRef CAS PubMed.
- E. Abele, K. Rubina, R. Abele, J. Popelis, I. Mazeika and E. Lukevics, Fluoride-ion-mediated reactions of trimethylsilylacetylene with carbonyl compounds and terminal acetylenes, J. Organomet. Chem., 1999, 586, 184–189 CrossRef CAS.
- A. B. Holmes, C. L. D. Jennings-White, A. H. Schulthess, B. Akinde and D. R. M. Walton, Selective desilylation of bis(trimethylsilyl)acetylenes, J. Chem. Soc., Chem. Commun., 1979, 840–842 RSC.
- R. B. Lettan and K. A. Scheidt, Lewis Base-Catalyzed Additions of Alkynes Using Trialkoxysilylalkynes, Org. Lett., 2005, 7, 3227–3230 CrossRef CAS PubMed.
- A. Mori, A. Fujita, K. Ikegashira, Y. Nishihara and T. Hiyama, TBAHF2 and TBAH2F3 as Activating Agents of Organosilanes, Synlett, 1997, 693–694 CrossRef CAS.
- T. Kitazawa, T. Minowa and T. Mukaiyama, Lewis Base-catalyzed Alkynylation of Carbonyl Compounds with Trimethylsilylacetylenes, Chem. Lett., 2006, 35, 1002–1003 CrossRef CAS.
- A. E. Favorskii and M. P. Skosarevskii, About the Reaction of Powdered Potassium Hydroxide on a Mixture of Phenylacetylene and Acetone, Zh. Russ. Khim. O-va., 1900, 32, 652 Search PubMed.
- H. Miyamoto, S. Yasaka and K. Tanaka, Solvent-Free Addition of Ethynylbenzene to Ketones, Bull. Chem. Soc. Jpn., 2001, 74, 185–186 CrossRef CAS.
- P. G. Cozzi, J. Rudolph, C. Bolm, P.-O. Norrby and C. Tomasini, Me2Zn-Mediated Addition of Acetylenes to Aldehydes and Ketones, J. Org. Chem., 2005, 70, 5733–5736 CrossRef CAS PubMed.
- D. N. Tomilin, O. V. Petrova, L. N. Sobenina, A. I. Mikhaleva and B. A. Trofimov, A convenient synthesis of hetarylethynyl ketones from hetarylcarbaldehydes and acetylene, Chem. Heterocycl. Compd., 2013, 49, 341–344 CrossRef CAS.
- E. Yu. Shmidt, I. A. Bidusenko, N. I. Protsuk, A. I. Mikhaleva and B. A. Trofimov, Improved synthesis of tertiary propargyl alcohols by the Favorskii reaction of alkyl aryl (hetaryl) ketones with acetylene, Russ. J. Org. Chem., 2013, 49, 8–11 CrossRef CAS.
- Y. N. Sum, D. Yu and Y. Zhang, Synthesis of acetylenic alcohols with calcium carbide as the acetylene source, Green Chem., 2013, 15, 2718–2721 RSC.
- B. A. Trofimov and E. Y. Schmidt, Reactions of acetylenes in superbasic media. Recent advances, Russ. Chem. Rev., 2014, 83, 600 CrossRef.
- E. Yu. Schmidt, N. A. Cherimichkina, I. A. Bidusenko, N. I. Protzuk and B. A. Trofimov, Alkynylation of Aldehydes and Ketones Using the Bu4NOH/H2O/DMSO Catalytic Composition: A Wide-Scope Methodology, Eur. J. Org. Chem., 2014, 4663–4670 CrossRef CAS.
- A. Hosseini, D. Seidel, A. Miska and P. R. Schreiner, Fluoride-Assisted Activation of Calcium Carbide: A Simple Method for the Ethynylation of Aldehydes and Ketones, Org. Lett., 2015, 17, 2808–2811 CrossRef CAS PubMed.
- V. V. Voronin, M. S. Ledovskaya, A. S. Bogachenkov, K. S. Rodygin and V. P. Ananikov, Acetylene in Organic Synthesis: Recent Progress and New Uses, Molecules, 2018, 23, 2442 CrossRef PubMed.
- K. S. Rodygin, M. S. Ledovskaya, V. V. Voronin, K. A. Lotsman and V. P. Ananikov, Calcium Carbide: Versatile Synthetic Applications, Green Methodology and Sustainability, Eur. J. Org. Chem., 2021, 43–52 CrossRef CAS.
- N. A. Sitte, F. Ghiringhelli, G. A. Shevchenko, F. Rominger, A. S. K. Hashmi and T. Schaub, Copper-Catalysed Synthesis of Propargyl Alcohol and Derivatives from Acetylene and other Terminal Alkynes, Adv. Synth. Catal., 2022, 364, 2227–2234 CrossRef CAS.
- E. M. Leitao, T. Jurca and I. Manners, Catalysis in service of main
group chemistry offers a versatile approach to p-block molecules and materials, Nat. Chem., 2013, 5, 817–829 CrossRef CAS PubMed.
- R. L. Melen, Dehydrocoupling routes to element–element bonds catalysed by main group compounds, Chem. Soc. Rev., 2016, 45, 775–788 RSC.
- L. C. Wilkins and R. L. Melen, Enantioselective Main Group Catalysis: Modern Catalysts for Organic Transformations, Coord. Chem. Rev., 2016, 324, 123–139 CrossRef CAS.
- K. Kuciński and G. Hreczycho, Hydrosilylation and hydroboration in a sustainable manner: from Earth-abundant catalysts to catalyst-free solutions, Green Chem., 2020, 22, 5210–5224 RSC.
- M. M. D. Roy, A. A. Omaña, A. S. S. Wilson, M. S. Hill, S. Aldridge and E. Rivard, Molecular Main Group Metal Hydrides, Chem. Rev., 2021, 121, 12784–12965 CrossRef CAS PubMed.
- T. R. Puleo, S. J. Sujansky, S. E. Wright and J. S. Bandar, Organic superbases in recent synthetic methodology research, Chem. – Eur. J., 2021, 27, 4216–4229 CrossRef CAS PubMed.
- T. X. Gentner and R. E. Mulvey, Alkali–Metal Mediation: Diversity of Applications in Main–Group Organometallic Chemistry, Angew. Chem., Int. Ed., 2021, 60, 9247–9262 CrossRef CAS PubMed.
- H. Gao, A. Battley and E. M. Leitao, The ultimate Lewis acid catalyst: using tris (pentafluorophenyl) borane to create bespoke siloxane architectures, Chem. Commun., 2022, 58, 7451–7465 RSC.
- M. Magre, M. Szewczyk and M. Rueping, s-Block metal catalysts for the hydroboration of unsaturated bonds, Chem. Rev., 2022, 122, 8261–8312 CrossRef CAS PubMed.
- K. Kuciński, H. Stachowiak-Dłużyńska and G. Hreczycho, Catalytic silylation of O–nucleophiles via Si–H or Si–C bond cleavage: A route to silyl ethers, silanols and siloxanes, Coord. Chem. Rev., 2022, 459, 214456 CrossRef.
- S. E. Wright and J. S. Bandar, A Base-Promoted Reductive Coupling Platform for the Divergent Defluorofunctionalization of Trifluoromethylarenes, J. Am. Chem. Soc., 2022, 144, 13032–13038 CrossRef CAS PubMed.
- S. P. Pajk, Z. Qi, S. J. Sujansky and J. S. Bandar, A base-catalyzed approach for the anti-Markovnikov hydration of styrene derivatives, Chem. Sci., 2022, 13, 11427–11432 RSC.
- K. Kuciński and G. Hreczycho, Lithium triethylborohydride as catalyst for solvent-free hydroboration of aldehydes and ketones, Green Chem., 2019, 21, 1912–1915 RSC.
- K. Kuciński, H. Stachowiak and G. Hreczycho, Silylation of silanols with hydrosilanes via main-group catalysis: the synthesis of unsymmetrical siloxanes and hydrosiloxanes, Inorg. Chem. Front., 2020, 7, 4190–4196 RSC.
- K. Kuciński, H. Stachowiak and G. Hreczycho, Silylation of Alcohols, Phenols, and Silanols with Alkynylsilanes – an Efficient Route to Silyl Ethers and Unsymmetrical Siloxanes, Eur. J. Org. Chem., 2020, 4042–4049 CrossRef.
- K. Kuciński and G. Hreczycho, Transition Metal-Free Catalytic C–H Silylation of Terminal Alkynes with bis(Trimethylsilyl)acetylene Initiated by KHMDS, ChemCatChem, 2022, 14, e202200794 Search PubMed.
- K. Kuciński and G. Hreczycho, Silicon–nitrogen bond formation via dealkynative coupling of amines with bis(trimethylsilyl)acetylene mediated by KHMDS, Chem. Commun., 2022, 58, 11386–11389 RSC.
- E. Szafoni, K. Kuciński and G. Hreczycho, Cobalt-catalyzed synthesis of silyl ethers via cross-dehydrogenative coupling between alcohols and hydrosilanes, Green Chem. Lett. Rev., 2022, 15, 757–764 CrossRef CAS.
- H. Stachowiak-Dłużyńska, K. Kuciński, K. Broniarz, E. Szafoni, M. Gruszczyński, D. Lewandowski, G. Consiglio and G. Hreczycho, Access to germasiloxanes and alkynylgermanes mediated by earth-abundant species, Sci. Rep., 2023, 13, 5618 CrossRef PubMed.
- O. V. Khoroshilova, I. A. Boyarskaya and A. V. Vasilyev, Synthesis of α-(Trifluoromethyl)styrenes and 1,3-Di(trifluoromethyl)indanes via Electrophilic Activation of TMS Ethers of (Trifluoromethyl)benzyl Alcohols in Brønsted Acids, J. Org. Chem., 2022, 87, 15845–15862 CrossRef CAS PubMed.
- A. J. Pearce, X. Y. See and I. A. Tonks, Oxidative nitrene transfer from azides to alkynes via Ti(II)/Ti(IV) redox catalysis: formal [2 + 2 + 1] synthesis of pyrroles, Chem. Commun., 2018, 54, 6891–6894 RSC.
- V. Maraval, C. Duhayon, Y. Coppel and R. Chauvin, The Intricate Assembling of gem-Diphenylpropargylic Units, Eur. J. Org. Chem., 2008, 5144–5156 CrossRef CAS.
-
I. Kownacki, B. Marciniec, B. Dudziec, A. Kownacka, M. Majchrzak, M. Szulc and B. Orwat, New (Triorganosilyl)Alkynes and Their Derivatives and a New Catalytic Method for Obtaining New and Conventional Substituted (Triorganosilyl)Alkynes and Their Derivatives, US Patent, US2014005427A1, 2014 Search PubMed.
- M. R. Hall, M. Korb, S. A. Moggach and P. J. Low, Further Chemistry of Ruthenium Alkenyl Acetylide Complexes: Routes to Allenylidene Complexes via a Series of Electrophilic Addition Reactions, Organometallics, 2020, 39, 2838–2853 CrossRef CAS.
Footnotes |
† Electronic supplementary information (ESI) available: Characterization data including NMR spectra. See DOI: https://doi.org/10.1039/d3qo00579h |
‡ The authors have cited additional references within the ESI.59–61 |
|
This journal is © the Partner Organisations 2023 |