DOI:
10.1039/D3QM00574G
(Chemistry Frontiers)
Mater. Chem. Front., 2023,
7, 3797-3802
Lessons learned: how to report XPS data incorrectly about lead-halide perovskites
Received
17th May 2023
, Accepted 8th July 2023
First published on 11th July 2023
Abstract
X-Ray photoelectron spectroscopy is a powerful tool for identifying the interactions of additives or surface treatments with components in lead halide perovskites. However, with the increasing number of studies using XPS, inaccurate or faulty data analysis has been encountered during a literature survey. Herein, we describe the fundamental principle of chemical shifts of Pb atoms in XPS and critically review the commonly seen mistakes in the literature: (i) misinterpretation of the XPS mechanism; (ii) misinterpretations due to disturbed chemical environments; (iii) lack of awareness of the properties of the passivator; iv. misquoted references. We hope that this perspective can help the community avoid the pitfalls in applying the XPS technique and in explaining their experimental results.
Perovskite solar cells (PSCs)1–12 have become a game changer in the field of solar cells, and specifically an up-and-coming candidate in replacing the otherwise predominant silicon solar cells due to their relatively low preparation costs and more straightforward fabrication process.13,14 PSCs, however, do show poor long-term stability—and to circumvent this issue, additives or surface treatments (namely passivation) that target the origins of various defects are commonly adopted.7,15–18 In this context, the interactions between these various additives and the components in the perovskites could readily be unveiled qualitatively and quantitatively using X-ray photoelectron spectroscopy (XPS). By analyzing the XPS data of a target material, it is possible to determine its elemental composition and empirical formula as well as the electronic and chemical states of its elements. Therefore, XPS, which involves recording the kinetic energy of electrons that are emitted from the top 1–10 nm of the material being irradiated with a beam of X-rays, is gaining popularity in characterizing the role of these passivation strategies19 (Fig. 1). The basis of a correct XPS data analysis is the accurate calibration of the binding energy (EB),20 including calibration of the energy scale of the spectrometer and the deviation caused by the charging effect (usually with C 1s data of adventitious carbon21 or decorated noble metal22).
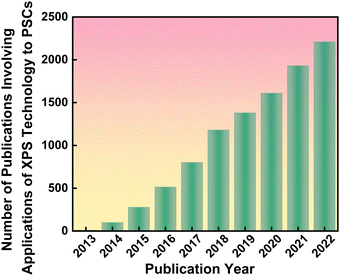 |
| Fig. 1 Plot of the number of publications per year involving perovskite solar cells and XPS according to a Google Scholar search performed in January 2023 for the term “perovskite solar cell AND (XPS OR X-ray photoelectron spectroscopy)”. | |
The electron binding energy (EB) could be calculated using the equation EB = hν − EK − WF,23 in which hν is the photon energy, EK is the measured kinetic energy, and WF is the spectrometer work function. EB is characteristically distinct for atoms with different electronic shells, and it is also, to some extent, dependent on the chemical environment of the atom. Namely, a change in the chemical state (chemical environment) could be reflected in shifted energies of expelled photoelectrons.24 The higher the electron density around an atom, the higher the EK of photoelectrons originating from its core levels, and hence the lower the chemical shift of the corresponding EB peaks in the spectrum (Fig. 2).25 In studies of PSCs, the chemical shift of the Pb 4f signal is the most commonly chosen one to verify the interaction of perovskite with functional groups of additives. Theoretically, depending on the nature (electron-accepting or electron-donating) of the functional group, a shift of the Pb 4f signal toward a higher EB is due to a decrease in the electron density on the Pb atom. In fact, the standard conduction of XPS on perovskites has already been introduced by Schulz et al., including the normative measurement conditions and correct analysis of binding energy spectra.26,27 However, as said by Aristotle, “one may go wrong in many different ways, but right only in one, which is why it is easy to fail and difficult to succeed”, and so it is necessary to point out directly the mistakes made subconsciously in XPS measurements.
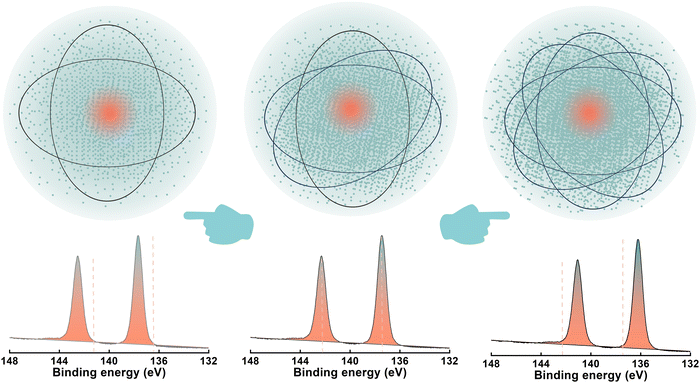 |
| Fig. 2 Schematic relationship between the electron density on the Pb atom and the chemical shift. | |
Unfortunately, some reports have provided inaccurate or inappropriate analysis of the XPS data (e.g., Pb 4f spectra) due to (i) misinterpretation of the XPS mechanism, (ii) misinterpretation due to a disturbed chemical environment, (iii) lack of awareness about the properties of the passivator, and (iv) misquoted references. Herein, a perspective on the correct understanding of XPS data is given based on examples containing erroneous/inadequate analysis of the chemical shifts in XPS. We hope that this critical perspective, which complements the work done by Schulz et al. on properly analyzing XPS data,27 will help the community avoid the pitfalls of applying the XPS technique when explaining their experimental results.
Misinterpretation of the XPS mechanism
Misinterpretations of the chemical shifts in XPS spectra are easily found in the literature. For instance, Cheng et al. concluded that the electron density around the Pb atom increased based on an actually higher-energy-shifted EB.28 In another case, Zheng et al. claimed that the higher EB at the Pb 4f level resulted from additional negative charges surrounding Pb.29 These studies conceptually misinterpreted the rule of chemical shifts in XPS, since there is no direct effect on the binding energy of core-level electrons for the different valence-charge densities because electrons do not have different energies but simultaneously share the total energy of the whole system.19,30 A photoelectron from Pb in a Pb–Cl unit would reach the detector with a lower kinetic energy than would that from a Pb–I unit due to the higher electronegativity of Cl and poor screening of the core holes.30
Misinterpretation due to disturbed chemical environments
In fact, the evolution of the chemical environment of the observed atomic species can be determined by tracking chemical shifts in the photoelectron binding energy (EB) for the multicomponent halide perovskite.27 But the operation of the XPS equipment and collection of data may not be normative. This issue is reflected in two works on the same compound, with opposite reported effects on the chemical shift of a Pb 4f signal.31–36 For example, the Pb 4f EB was reported by Wang et al. to shift to lower energy in perovskite films with a PEG/PVA overlayer31 (Fig. 3a), but the same polymers were reported to show the opposite shifts by Chen et al.32 (Fig. 3b). The former attributed the down-shifting of Pb 4f binding energy to increased electron cloud density of the Pb atom due to a strong interaction with PVA/PEG,31 while the latter believed that the observed up-shifting of the Pb core level was due to decreased electron density by the same polymer via a weaker coordination between the functional groups with Pb than with I−.32 Likewise, contradictory conclusions have been reported in works about ammonium-salt-modified perovskites: Kang et al. reported down-shifting Pb 4f and I 3d EB values in the presence of 2D TBA2PbI4 in an FAPbI3 film and speculated that the TBA cation reduced the oxidation state of Pb and I or weakened the Pb–I bond (Fig. 3c);33 in contrast, Liu et al. reported no change in the oxidation state of Pb and I in CsPbI3 after TBAI treatment and formation of 1D TBAPbI3 (Fig. 3d).34 Similarly, the Pb 4f EB was reported to shift differently in two separate works using PbS as the surface sulfidation treatment (SST) material for perovskites: to higher energy in one report, attributed to the strong bonding between S and Pb (Fig. 3e);35 but with a reversed chemical shift on the same core level after the same treatment according to the other work (Fig. 3f).36 We believe that these contradictory experimental data could be due to unregulated operation by inexperienced researchers, which may result in artifacts in the measured chemical shifts under the same chemical environment.
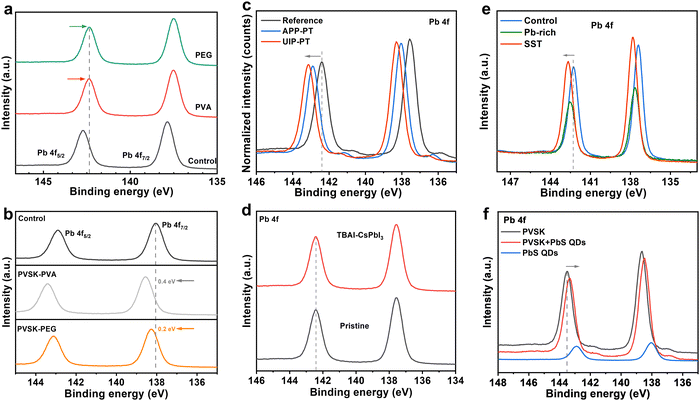 |
| Fig. 3 XPS spectra of Pb 4f peaks in perovskite films (a) without and (b) with PEG/PVA; (c) before (reference) and after post-treatment by UIPPT or APP-PT; (d) CsPbI3 films before and after TBAI treatment; (e) perovskite films (control), Pb-rich perovskite films (Pb-rich) before and after surface sulfidation treatment (SST-based); (f) pristine PbS QDs, perovskite films without (PVSK) and with PbS QDs (PVSK + PbS QDs). | |
Lack of awareness of the properties of the passivator
As described by Schulz et al., the case of Pb is interesting because the corresponding EB is influenced by the halide to which it is bound.27 It is generally believed that the electron-donating nature of a Lewis base can lead to a downward shift in the EB of the Pb 4f peak.14,31,37–42 Assuming that the experimental conditions in the same publication are consistent, the changes in Pb binding energy should be attributed to the added passivator. Unfortunately, the logical relationship between the XPS chemical shift and the role of additives in some reports is not self-consistent. For instance, the dipolar PCBB-3N-3I was deposited, in a work published in 2019, to passivate under-coordinated Pb2+via the negatively charged terminal groups.43 However, this work reported an upward-shifted Pb 4f core level energy, indicating decreased electron density. So how could a more electron-poor under-coordinated Pb2+ be called passivated? Similarly, Liu et al. reported the use of (5-mercapto-1,3,4-thiadiazol-2-ylthio) acetic acid (MTDAA) as a multi-active-site Lewis-base that shifted the Pb 4f EB towards higher energy.44 The contradictory conclusions suggest that the authors did not establish a clear connection between the concept of increased electron density in a passivated under-coordinated Pb2+ and the specific passivator they employed.45–49
Misquoted references
Sometimes, a report may blindly cite the conclusion from another published report with different experimental results, leading to a biased interpretation. By illustration, Hu et al., when using XPS to prove the incorporation of Ln3+ into CsPbBr3, first claimed the increased electron density around the Pb atom (down-shifting Pb 4f EB) to be due to a stronger interaction between Ln3+ and Br−.50 Later, another work by Duan et al. misquoted the conclusion from ref. 45 to explain the observed up-shifting of Pb and Br core levels after Ln3+ doping.51 Also, pyridine is commonly regarded as a Lewis base to bind with a Lewis acid and, therefore, as a passivator for perovskite.52 However, such a conclusion has been arbitrarily used, including even when the nitrogen of the pyridine was quaternized, in which the positive nitrogen cation cannot function as an electron donor and, therefore, cannot be indicated to be responsible for the upward shifting Pb 4f core level.53 To avoid these situations, it is essential to “seek truth from facts” and fully understand the previous studies before using them to support the new data.
In brief, to use XPS as a powerful tool for understanding the material science of perovskites, more comprehensive knowledge combining the fundamental mechanism of XPS and the electronic structure of the target compounds is needed. Furthermore, a consistent logical data analysis should be used for drawing conclusions based on XPS and chemical theories. Last but not least, rigorous handling, correct calibration, and careful referencing are also crucial for reporting XPS data.
Data availability statement
The data that support the findings of this study are available from the corresponding author on request and will be supplied on a reasonable time period.
Author contributions
C. L. and N. Z. performed the leading research, prepared the manuscript, and contributed equally to this work. P. G. provided financial support and guided the project.
Conflicts of interest
The authors declare no conflict of interest.
Acknowledgements
P. G. acknowledges the National Natural Science Foundation of China (Grant No. 22175180, 21975260).
References
- A. Kojima, K. Teshima, Y. Shirai and T. Miyasaka, Organometal halide perovskites as visible-light sensitizers for photovoltaic cells, J. Am. Chem. Soc., 2009, 131, 6050–6051 CrossRef CAS PubMed.
- H.-S. Kim, C.-R. Lee, J.-H. Im, K.-B. Lee, T. Moehl, A. Marchioro, S.-J. Moon, R. Humphry-Baker, J.-H. Yum, J. E. Moser, M. Grätzel and N.-G. Park, Lead iodide perovskite sensitized all-solid-state submicron thin film mesoscopic solar cell with efficiency exceeding 9%, Sci. Rep., 2012, 2, 591 CrossRef PubMed.
- J. Burschka, N. Pellet, S.-J. Moon, R. Humphry-Baker, P. Gao, M. K. Nazeeruddin and M. Grätzel, Sequential deposition as a route to high-performance perovskite-sensitized solar cells, Nature, 2013, 499, 316–319 CrossRef CAS PubMed.
- M. Liu, M. B. Johnston and H. J. Snaith, Efficient planar heterojunction perovskite solar cells by vapour deposition, Nature, 2013, 501, 395–398 CrossRef CAS PubMed.
- N. J. Jeon, J. H. Noh, Y. C. Kim, W. S. Yang, S. Ryu and S. I. Seok, Solvent engineering for high-performance inorganic-organic hybrid perovskite solar cells, Nat. Mater., 2014, 13, 897–903 CrossRef CAS PubMed.
- M. Saliba, T. Matsui, J.-Y. Seo, K. Domanski, J.-P. Correa-Baena, M. K. Nazeeruddin, S. M. Zakeeruddin, W. Tress, A. Abate, A. Hagfeldt and M. Grätzel, Cesium-containing triple cation perovskite solar cells: improved stability, reproducibility and high efficiency, Energy Environ. Sci., 2016, 9, 1989–1997 RSC.
- Q. Jiang, Y. Zhao, X. Zhang, X. Yang, Y. Chen, Z. Chu, Q. Ye, X. Li, Z. Yin and J. You, Surface passivation of perovskite film for efficient solar cells, Nat. Photonics, 2019, 13, 460–466 CrossRef CAS.
- G. Kim, H. Min, S. Lee Kyoung and Y. Lee, Do, M. Yoon So and I. Seok Sang, Impact of strain relaxation on performance of α-formamidinium lead iodide perovskite solar cells, Science, 2020, 370, 108–112 CrossRef CAS PubMed.
- H. Min, D. Y. Lee, J. Kim, G. Kim, K. S. Lee, J. Kim, M. J. Paik, Y. K. Kim, K. S. Kim, M. G. Kim, T. J. Shin and S. I. Seok, Perovskite solar cells with atomically coherent interlayers on SnO2 electrodes, Nature, 2021, 598, 444–450 CrossRef CAS PubMed.
- Best Research-Cell Efficiencies Chart, https://www.nrel.gov/pv/cell-efficiency.html, accessed 21 December, 2022.
- P. Gao, M. Grätzel and M. K. Nazeeruddin, Organohalide lead perovskites for photovoltaic applications, Energy Environ. Sci., 2014, 7, 2448–2463 RSC.
- Z. Zhang, L. Qiao, K. Meng, R. Long, G. Chen and P. Gao, Rationalization of passivation strategies toward high-performance perovskite solar cells, Chem. Soc. Rev., 2023, 52, 163–195 RSC.
- J. Huang, Y. Yuan, Y. Shao and Y. Yan, Understanding the physical properties of hybrid perovskites for photovoltaic applications, Nat. Rev. Mater., 2017, 2, 17042 CrossRef CAS.
- J. Y. Kim, J.-W. Lee, H. S. Jung, H. Shin and N.-G. Park, High-efficiency perovskite solar cells, Chem. Rev., 2020, 120, 7867–7918 CrossRef CAS PubMed.
- W. Hui, L. Chao, H. Lu, F. Xia, Q. Wei, Z. Su, T. Niu, L. Tao, B. Du, D. Li, Y. Wang, H. Dong, S. Zuo, B. Li, W. Shi, X. Ran, P. Li, H. Zhang, Z. Wu, C. Ran, L. Song, G. Xing, X. Gao, J. Zhang, Y. Xia, Y. Chen and W. Huang, Stabilizing black-phase formamidinium perovskite formation at room temperature and high humidity, Science, 2021, 371, 1359 CrossRef CAS PubMed.
- J. Luo, J. Xia, H. Yang, L. Chen, Z. Wan, F. Han, H. A. Malik, X. Zhu and C. Jia, Toward high-efficiency, hysteresis-less, stable perovskite solar cells: unusual doping of a hole-transporting material using a fluorine-containing hydrophobic Lewis acid, Energy Environ. Sci., 2018, 11, 2035–2045 RSC.
- N. Ahn, D.-Y. Son, I.-H. Jang, S. M. Kang, M. Choi and N.-G. Park, Highly reproducible perovskite solar cells with average efficiency of 18.3% and best efficiency of 19.7% fabricated via Lewis base adduct of lead(II) iodide, J. Am. Chem. Soc., 2015, 137, 8696–8699 CrossRef CAS PubMed.
- C. Luo, G. Zheng, F. Gao, X. Wang, Y. Zhao, X. Gao and Q. Zhao, Facet orientation tailoring via 2D-seed-induced growth enables highly efficient and stable perovskite solar cells, Joule, 2022, 6, 240–257 CrossRef CAS.
- G. Greczynski and L. Hultman, A step-by-step guide to perform x-ray photoelectron spectroscopy, J. Appl. Phys., 2022, 132, 011101 CrossRef CAS.
- G. Greczynski and L. Hultman, Compromising science by ignorant instrument calibration—Need to revisit half a century of published XPS data, Angew. Chem., Int. Ed., 2020, 59, 5002–5006 CrossRef CAS PubMed.
- D. R. Baer, Summary of ISO/TC 201 standard: XVIII, ISO 19318:2004—surface chemical analysis—X-ray photoelectron spectroscopy—Reporting of methods used for charge control and charge correction, Surf. Interface Anal., 2005, 37, 524–526 CrossRef CAS.
- D. J. Hnatowich, J. Hudis, M. L. Perlman and R. C. Ragaini, Determination of charging effect in photoelectron spectroscopy of nonconducting solids, J. Appl. Phys., 1971, 42, 4883–4886 CrossRef CAS.
- A. Einstein, Über einen die Erzeugung und Verwandlung des Lichtes betreffenden heuristischen Gesichtspunkt, Ann. Phys., 1905, 322, 132–148 CrossRef.
- A. Fahlman, K. Hamrin, J. Hedman, R. Nordberg, C. Nordling and K. Siegbahn, Electron spectroscopy and chemical binding, Nature, 1966, 210, 4–8 CrossRef CAS.
-
K. Siegbahn and N. Nordling, ESCA: Atomic, molecular and solid state structure studied by means of electron spectroscopy, Upsala, 1967 Search PubMed.
- R. L. Z. Hoye, P. Schulz, L. T. Schelhas, A. M. Holder, K. H. Stone, J. D. Perkins, D. Vigil-Fowler, S. Siol, D. O. Scanlon, A. Zakutayev, A. Walsh, I. C. Smith, B. C. Melot, R. C. Kurchin, Y. Wang, J. Shi, F. C. Marques, J. J. Berry, W. Tumas, S. Lany, V. Stevanović, M. F. Toney and T. Buonassisi, Perovskite-Inspired Photovoltaic Materials: Toward Best Practices in Materials Characterization and Calculations, Chem. Mater., 2017, 29, 1964–1988 CrossRef CAS.
- S. Béchu, M. Ralaiarisoa, A. Etcheberry and P. Schulz, Photoemission spectroscopy characterization of halide perovskites, Adv. Energy Mater., 2020, 10, 1904007 CrossRef.
- W. Cheng, X. He, J.-G. Wang, W. Tian and L. Li, N-(2-aminoethyl) acetamide additive enables phase-pure and stable α-FAPbI3 for efficient self-powered photodetectors, Adv. Mater., 2022, 34, 2208325 CrossRef CAS PubMed.
- T. Zheng, L. Fan, H. Zhou, Y. Zhao, B. Jin and R. Peng, Engineering of electron extraction and defect passivation via anion-doped conductive fullerene derivatives as interlayers for efficient invert perovskite
solar cells, ACS Appl. Mater. Interfaces, 2020, 12, 24747–24755 CrossRef CAS PubMed.
- G. Greczynski and L. Hultman, X-ray photoelectron spectroscopy: Towards reliable binding energy referencing, Prog. Mater. Sci., 2020, 107, 100591 CrossRef CAS.
- M. Wang, Y. Zhao, X. Jiang, Y. Yin, I. Yavuz, P. Zhu, A. Zhang, G. S. Han, H. S. Jung, Y. Zhou, W. Yang, J. Bian, S. Jin, J.-W. Lee and Y. Yang, Rational selection of the polymeric structure for interface engineering of perovskite solar cells, Joule, 2022, 6, 1032–1048 CrossRef CAS.
- C. Chen, X. Wang, Z. Li, X. Du, Z. Shao, X. Sun, D. Liu, C. Gao, L. Hao, Q. Zhao, B. Zhang, G. Cui and S. Pang, Polyacrylonitrile-coordinated perovskite solar cell with open-circuit voltage exceeding 1.23 V, Angew. Chem., Int. Ed., 2021, 61, e202113932 Search PubMed.
- D.-H. Kang, S.-Y. Kim, J.-W. Lee and N.-G. Park, Efficient surface passivation of perovskite films by a post-treatment method with a minimal dose, J. Mater. Chem. A, 2021, 9, 3441–3450 RSC.
- X. Liu, X. Wang, T. Zhang, Y. Miao, Z. Qin, Y. Chen and Y. Zhao, Organic tetrabutylammonium cation intercalation to heal inorganic CsPbI3 perovskite, Angew. Chem., Int. Ed., 2021, 60, 12351–12355 CrossRef CAS PubMed.
- X. Li, W. Zhang, X. Guo, C. Lu, J. Wei and J. Fang, Constructing heterojunctions by surface sulfidation for efficient inverted perovskite solar cells, Science, 2022, 375, 434–437 CrossRef CAS PubMed.
- R. Ma, Z. Ren, C. Li, Y. Wang, Z. Huang, Y. Zhao, T. Yang, Y. Liang, X. W. Sun and W. C. H. Choy, Establishing multifunctional interface layer of perovskite ligand modified lead sulfide quantum dots for improving the performance and stability of perovskite solar cells, Small, 2020, 16, 2002628 CrossRef CAS PubMed.
- J. Xia, C. Liang, S. Mei, H. Gu, B. He, Z. Zhang, T. Liu, K. Wang, S. Wang, S. Chen, Y. Cai and G. Xing, Deep surface passivation for efficient and hydrophobic perovskite solar cells, J. Mater. Chem. A, 2021, 9, 2919–2927 RSC.
- Q. Yao, Q. Xue, Z. Li, K. Zhang, T. Zhang, N. Li, S. Yang, C. J. Brabec, H.-L. Yip and Y. Cao, Graded 2D/3D perovskite heterostructure for efficient and operationally stable MA-Free perovskite solar cells, Adv. Mater., 2020, 32, 2000571 CrossRef CAS PubMed.
- Z. Wu, M. Jiang, Z. Liu, A. Jamshaid, L. K. Ono and Y. Qi, Highly efficient perovskite solar cells enabled by multiple ligand passivation, Adv. Energy Mater., 2020, 10, 1903696 CrossRef CAS.
- X. Wang, W. Sun, Y. Tu, Q. Xiong, G. Li, Z. Song, Y. Wang, Y. Du, Q. Chen, C. Deng, Z. Lan, P. Gao and J. Wu, Lansoprazole, a cure-four, enables perovskite solar cells efficiency exceeding 24%, Chem. Eng. J., 2022, 446, 137416 CrossRef CAS.
- Abd R. B. Mohd Yusoff, M. Vasilopoulou, D. G. Georgiadou, L. C. Palilis, A. Abate and M. K. Nazeeruddin, Passivation and process engineering approaches of halide perovskite films for high efficiency and stability perovskite solar cells, Energy Environ. Sci., 2021, 14, 2906–2953 RSC.
- T. Wu, Y. Wang, X. Li, Y. Wu, X. Meng, D. Cui, X. Yang and L. Han, Efficient Defect Passivation for Perovskite Solar Cells by Controlling the Electron Density Distribution of Donor-π-Acceptor Molecules, Adv. Energy Mater., 2019, 9, 1803766 CrossRef.
- M. Zhang, Q. Chen, R. Xue, Y. Zhan, C. Wang, J. Lai, J. Yang, H. Lin, J. Yao, Y. Li, L. Chen and Y. Li, Reconfiguration of interfacial energy band structure for high-performance inverted structure perovskite solar cells, Nat. Commun., 2019, 10, 4593 CrossRef PubMed.
- B. Liu, H. Bi, D. He, L. Bai, W. Wang, H. Yuan, Q. Song, P. Su, Z. Zang, T. Zhou and J. Chen, Interfacial defect passivation and stress release via multi-active-site ligand anchoring enables efficient and stable methylammonium-free perovskite solar cells, ACS Energy Lett., 2021, 6, 2526–2538 CrossRef CAS.
- X. Yang, Y. Fu, R. Su, Y. Zheng, Y. Zhang, W. Yang, M. Yu, P. Chen, Y. Wang, J. Wu, D. Luo, Y. Tu, L. Zhao, Q. Gong and R. Zhu, Superior carrier lifetimes exceeding 6 μs in polycrystalline halide perovskites, Adv. Mater., 2020, 32, 2002585 CrossRef CAS PubMed.
- B. Chen, P. N. Rudd, S. Yang, Y. Yuan and J. Huang, Imperfections and their passivation in halide perovskite solar cells, Chem. Soc. Rev., 2019, 48, 3842–3867 RSC.
- X. Yang, Y. Ni, Y. Zhang, Y. Wang, W. Yang, D. Luo, Y. Tu, Q. Gong, H. Yu and R. Zhu, Multiple-defect management for efficient perovskite photovoltaics, ACS Energy Lett., 2021, 6, 2404–2412 CrossRef CAS.
- Z. Zhang, Y. Gao, Z. Li, L. Qiao, Q. Xiong, L. Deng, Z. Zhang, R. Long, Q. Zhou, Y. Du, Z. Lan, Y. Zhao, C. Li, K. Müllen and P. Gao, Marked passivation effect of naphthalene-1,8-Dicarboximides in high-performance perovskite solar cells, Adv. Mater., 2021, 33, 2008405 CrossRef CAS PubMed.
- L. Scalon, R. Szostak, F. L. Araújo, K. F. Adriani, J. F. R. V. Silveira, W. X. C. Oliveira, J. L. F. Da Silva, C. C. Oliveira and A. F. Nogueira, Improving the stability and efficiency of perovskite solar cells by a bidentate anilinium salt, JACS Au, 2022, 2, 1306–1312 CrossRef CAS PubMed.
- Q. Hu, Z. Li, Z. Tan, H. Song, C. Ge, G. Niu, J. Han and J. Tang, Rare earth ion-doped CsPbBr3 nanocrystals, Adv. Opt. Mater., 2018, 6, 1700864 CrossRef.
- J. Duan, Y. Zhao, X. Yang, Y. Wang, B. He and Q. Tang, Lanthanide ions doped CsPbBr3 halides for HTM-Free 10.14%-efficiency inorganic perovskite solar cell with an ultrahigh open-circuit voltage of 1.594 V, Adv. Energy Mater., 2018, 8, 1802346 CrossRef.
- R. Fu, Y. Zhao, Q. Li, W. Zhou, D. Yu and Q. Zhao, Enhanced long-term stability of perovskite solar cells by 3-hydroxypyridine dipping, Chem. Commun., 2017, 53, 1829–1831 RSC.
- X. Wu, L. Zhang, Z. Xu, S. Olthof, X. Ren, Y. Liu, D. Yang, F. Gao and S. (Frank), Liu, Efficient perovskite solar cells via surface passivation by a multifunctional small organic ionic compound, J. Mater. Chem. A, 2020, 8, 8313–8322 RSC.
Footnote |
† These authors contributed equally to this work. |
|
This journal is © the Partner Organisations 2023 |