DOI:
10.1039/D3QI00230F
(Research Article)
Inorg. Chem. Front., 2023,
10, 2268-2275
Structure and optical property evolution in PbM(PO4)X (M = Zn, Sn; X = halogen): SHG effect and birefringence†
Received
6th February 2023
, Accepted 7th March 2023
First published on 7th March 2023
Abstract
Four new phosphates, namely, PbZn(PO4)F (1), PbSn(PO4)Cl (2), PbSn(PO4)Br (3) and PbSn(PO4)I (4), were successfully synthesized by hydrothermal and solid-state reaction methods. PbZn(PO4)F (1) was crystallized in a polar space group Pna21 and showed a significant SHG intensity of about 1.6 × KDP. PbSn(PO4)Cl (2) and PbSn(PO4)Br (3) were isostructural compounds, and they were crystallized in a monoclinic space group P21/n while PbSn(PO4)I (4) was crystallized in a triclinic space group P
. PbZn(PO4)F (1) features a new 3D framework while compounds 2–4 show a layered structure. Interestingly, the thermal stability and band gap of PbM(PO4)X are decreased with an increase of the atomic number of the halogen atom, while the rule for birefringence is the opposite. Our work revealed the evolution effects of the halogen atoms on the structure and optical properties of PbM(PO4)X compounds.
Introduction
As a functional material, nonlinear optical (NLO) materials are important components for expanding the wavelengths of lasers and enhancing the performance of laser light sources.1–3 Over the decades, generations of researchers have built huge collections of NLO materials around their required characteristics, which include typical borates,4–6 iodates,7–9 chalcogenides10–12 and phosphates.13–16 Currently, metal phosphates are an important source for NLO material applications due to their structural advantages and good physicochemical properties, with familiar KTiOPO4 (KTP) and KH2PO4 (KDP) being reported and successfully commercialized in the 1970s. In recent years, a series of novel metal phosphates were successfully explored as promising NLO materials, such as LiPbPO4 (about 3 × KDP, 0.021@1064 nm),17 Cs2LiPO4 (1.8 × KDP),18 LiHgPO4 (11 × KDP, 0.068@1064 nm),19 KMg(H2O)PO4 (about 1.14 × KDP, 0.017@1064 nm),20 Rb3PbBi(P2O7)2 (2.5 × KDP, 0.025@1064 nm),21 Bi32Cd3P10O76 (about 4 × KDP),22 β-Cd(PO3)2 (0.25 × KDP, 0.059@1064 nm),23 Na3Sc2(PO4)2F3 (0.26 × KDP, 0.0978@546.1 nm),24 CsMgPO4·6H2O (1.36 × KDP, 0.0060@1064 nm),25 K4Mg4(P2O7)3 (1.3 × KDP, 0.0108@1064 nm),26 and K2Sb(P2O7)F (4.0 × KDP, 0.157@546 nm).27 However, the microscopic second-order multiplicity coefficients of the PO4 group, the basic structural unit of phosphates, is much smaller than that of the BO3 group, and the rigid PO4 group also exhibits weak anisotropy, which greatly hampers the availability of phosphates in the field of NLO materials. After continuous exploration, researchers have concluded that the structure–property relationship of NLO materials,28–30 that is, the optimization of the arrangement of building units or the introduction of other NLO-active building blocks into the target material, can be used to strengthen the SHG effect and birefringence of phosphates. These strategies include: (1) introducing metal ions with second-order Jahn–Teller distortion, such as KTP (about 12 × KDP, 0.090@1064 nm);31 (2) breaking the symmetry of PO4 groups, such as NaNH4PO3F·H2O (1.1 × KDP, >0.053@589.3 nm);32 and (3) introducing other anionic groups, such as Cd2(IO3)(PO4) (4 × KDP, 0.0108@1064 nm),33etc.
The Zn2+ cation, as a d10 transition metal (TM), has an 18e electron configuration with strong polarizability and significant deformability and will eliminate unwanted red shifts at the absorption edge.34 In addition, Zn2+ can also exhibit various coordination configurations, such as ZnOx (x = 4, 5, 6). Therefore, Zn2+ is proposed to be a premium NLO active building unit, which can increase the opportunities for the SHG property and birefringence magnitude if the asymmetric units are properly aligned.35,36 Recently, some excellent Zn-based phosphates have been reported, such as Rb2Zn3(P2O7)2 (1 × KDP),37 K2ZnMoP2O10 (about 6.8 × KDP, 0.0534@450.2 nm),38 and Ba3(ZnB5O10)PO4 (4 × KDP, 0.035@532 nm).39 On the other hand, introducing metal cations with stereochemically active lone pairs (SCALP) has turned out to be a valid tactic for fabricating novel NLO and birefringence crystals.40,41 In addition to the classical metal cations Pb2+ and Bi3+, Sn2+ has also attracted a lot of attention in recent years. Emerging examples include BaSn2(PO4)2 (0.07@1064 nm),42 Sn2PO4X (X = F, Cl) (0.126@546 nm, 0.181@546 nm),43 and Sn2PO4I (0.468@1064 nm),44 demonstrating its great research potential.
Based on the above ideas and considering that different NLO active building units may have synergistic effects, we endeavored to combine SCALP Pb2+, d10 TM Zn2+ or SCALP Sn2+ and halogen ions into the phosphate system to obtain new phosphate NLO materials. Four new lead halide phosphates, namely, PbZn(PO4)F (1), PbSn(PO4)Cl (2), PbSn(PO4)Br (3) and PbSn(PO4)I (4), based on the PbM(PO4)X formula, were synthesized successfully. PbZn(PO4)F (1) exhibited a significant SHG effect about 1.6 times as large as that of commercial KDP while PbSn(PO4)I (4) possessed a high birefringence intensity of 0.095 at 1064 nm. Interestingly, their structures and optical properties evolved simultaneously with the alteration of halogen elements. In this work, we present their syntheses, crystallographic structures, optical properties and related theoretical calculations.
Experimental section
Materials
All materials were of analytical purity grade, were commercially available, and used as received. Lead oxide (PbO), tin(II) oxide (SnO), ammonium dihydrogen phosphate (NH4H2PO4), tin(II) chloride (SnCl2), tin(II) bromide (SnBr2), phosphorus pentoxide (P2O5), zinc fluoride (ZnF2), hydrofluoric acid (HF, 35%), hydrochloric acid (HCl, 37%), hydroiodic acid (HI, ≥47%) and phosphoric acid (H3PO4, 85%) were obtained from Aladdin.
Synthesis
The four compounds were synthesized utilizing two different approaches. Compounds PbZn(PO4)F (1), PbSn(PO4)Cl (2) and PbSn(PO4)I (4) were synthesized by a mild hydrothermal method. The starting reagents are PbO (1 mmol), ZnF2 (1.1 mmol), NH4H2PO4 (1 mmol), HF (0.1 mL), H3PO4 (0.8 mL) and H2O (3 mL) for 1; PbO (1 mmol), SnCl2 (1 mmol), NH4H2PO4 (1 mmol), (0.1 mL), H3PO4 (0.8 mL) and H2O (3 mL) for 2; and PbO (1 mmol), SnO (1 mmol), HI (0.15 mL), H3PO4 (0.6 mL) and H2O (3.0 mL) for 4. The above mixtures were transferred to an oven and heated at 230 °C, 190 °C and 210 °C for 72 h, respectively. Colourless rod-like crystals of PbZn(PO4)F (1) were obtained in a yield of 63% (based on Pb, the same as below). Tiny sheet-like crystals of PbSn(PO4)Cl (2) were mixed with some unknown impurities, which were difficult to separate from the product. We tried to obtain its pure phase by changing the experimental conditions, but failed. Yellow sheet-like crystals of PbSn(PO4)I (4) were obtained in a yield of 45%. Compound PbSn(PO4)Br (3) was synthesized by a high-temperature solid-state reaction. The raw materials of PbO, SnBr2, and P2O5 were well ground in a molar ratio of 1
:
1
:
1 in a glovebox and placed in a quartz tube, which was sealed at 10−3 Pa. The sample was heated at 650 °C for 48 h, and cooled to 480 °C at 2 °C h−1 before the muffle furnace was turned off. Impurities were washed away with distilled water. Clean crystals of 3 were obtained in a yield of 40%.
Single-crystal structure determination
Appropriately sized crystals of the four compounds were selected for structure determination. Crystal data were collected by operating a SuperNona CCD diffractometer with graphite monochromatic Mo Kα radiation (λ = 0.71073 Å). The collected data were subjected to cell refinement and data reduction using CrysAlisPro software, and the absorption correction processing was performed by the multi-scan method. Direct analysis of the structures was conducted using the SHELXTL-97 program with a full matrix least-squares fitting on F2.45 The structures were further checked using PLATON and no higher symmetries were observed.46 Relevant crystallographic data are outlined in Table S1.† The selected bond lengths are outlined in Table S2.†
Powder X-ray diffraction
Powder X-ray diffraction (PXRD) data were recorded on a Mini-flex 600 powder X-ray diffractometer at room temperature (RT) with Cu Kα radiation (λ = 1.540598 Å, 2θ = 10°–70°, increment = 0.02°). The PXRD plots of the compounds are presented in Fig. S1.†
UV-vis-NIR diffuse reflectance and infrared spectra
The UV-vis-NIR diffuse reflectance spectra (200–2000 nm) were recorded on a PerkinElmer Lamda-950 spectrophotometer; a powder BaSO4 plate served as the 100% reflectivity reference. The experimental band gaps were made available by the calculation of the absorption value from the reflection spectra following the Kubelka–Munk function.47 The infrared spectra (IR, 4000–400 cm−1) were recorded on a Magna 750 FT-IR spectrometer, with air serving as the background, to verify the P–O bonds in these compounds.
Thermal analysis
Thermal stability measurements were carried out under a constant N2 flow on a NETZSCH STA 449C simultaneous analyzer (heating rate: 15 °C min−1; temperature range: 20–1000 °C).
Second-harmonic generation
Powder second-harmonic generation (SHG) measurements were performed at RT using a Q-switched Nd:YAG laser at a wavelength of 1064 nm, following the Kurtz–Perry method. The output light intensity emitted from the samples was measured using a photomultiplier tube, and the same size of crystalline KDP (70–100 mesh) was used as the reference.48
Results and discussion
Structure of PbZn(PO4)F
PbM(PO4)X features three different structures and we obtained the pure phases of compounds 1, 3 and 4 successfully (Fig. S1†). PbZn(PO4)F is crystallized in the polar space group Pna21 (No. 33) and exhibited a 3D framework that was constructed from PO4 groups, ZnO4F triangular bipyramids and PbO2F2 units. Its asymmetric unit contained one Pb, one Zn, one P, one halogen and four O atoms. The P(1) atom coordinated with four O atoms to shape the tetrahedral configuration and the P–O bond lengths ranged from 1.515(11) to 1.551(8) Å. The Zn(1) atom was coordinated with one F and four O atoms, giving rise to a highly distorted triangular bipyramid, in which the bond angle of F(1)–Zn(1)–O(1) is equal to 161.3(3)° (Fig. 1a). The Pb(1) atom was coupled to two O atoms and two F atoms with Pb–O/F bond lengths in the range of 2.359(7)–2.514(10) Å. Due to the repulsion of the lone-pair electrons on Pb, the O and F atoms were gathered on one side of the Pb atom, resulting in a “seesaw” model of the PbO2F2 unit. The bond value calculations showed that the bond valences of Pb(1), Zn(1), P(1) and F(1) were 1.84, 2.07, 4.85 and 0.96, respectively, suggesting their oxidation states of +2, +2, +5 and −1, respectively.49,50
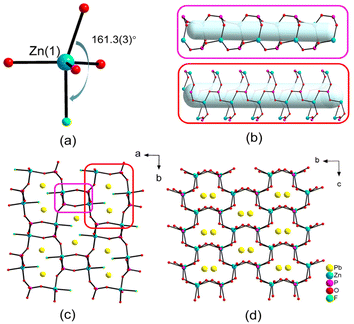 |
| Fig. 1 The ZnO4F triangular bipyramid (a), the 1D four-MPR channel (purple rectangle) and the eight-MPR channel (red rectangle) (b), the 3D structure of PbZn(PO4)F along the c-axis (c) and the a-axis (d) (the Pb–O and Pb–F bonds have been neglected for clarity). | |
The distorted ZnO4F triangular bipyramids and the PO4 tetrahedra were interconnected alternately into a 3D [Zn(PO4)F]2− anionic framework with four- and eight-member polyhedral ring (MPR) tunnels along the c-axis (Fig. 1b and c). The PbO2F2 units form a 1D [PbO2F2]4− chain along the c-axis (Fig. S2†), located at large eight-MPR tunnels. Viewed from the a-axis, there were six-member polyhedral ring tunnels, containing the Pb atoms, in the [Zn(PO4)F]2− anionic framework (Fig. 1d).
Structures of PbSn(PO4)Br and PbSn(PO4)I
PbSn(PO4)Cl (2) and PbSn(PO4)Br (3) were isostructural and crystallized in the monoclinic space group P21/n (No. 14). However, PbSn(PO4)I (4) crystallized in the triclinic space group P
(No. 2). The asymmetric units of compounds 2–4 were similar to PbZn(PO4)F (1), including one Pb, one Sn, one P, one halogen and four O atoms. All of the P(1) atoms coupled with four O atoms to shape a pyramidal configuration with the P–O bond distances ranging from 1.525(10) to 1.56(2) Å. The Sn(1) atom was coordinated with three O atoms to form a SnO3 triangular pyramid, in which the range of Sn–O bond distances was 2.104(19)–2.232(9) Å. The Pb atom was connected to four O atoms and one halogen atom in a PbO4X polyhedron. The Pb–O bond distances ranged from 2.426(9) to 2.74(2) Å, and the corresponding Pb–X bond distance was 2.830(4), 2.9770(15) and 3.135(2) Å for the Cl, Br and I compounds, respectively. The bond value calculation results showed that the bond valences of Pb, Sn, P and Cl/Br/I were 2.02/2.08/1.98, 1.90/1.81/1.90, 4.81/4.82/4.73, and 0.75/0.82/0.78, respectively, which suggests that the valences of Pb, Sn, P and halogen elements in the structures of 2–4 were +2, +2, +5 and −1, respectively.
Although the space groups of compounds 3 and 4 were different, their structures were very similar. In their structures, the PO4 tetrahedra and the SnO3 triangular pyramids were interconnected alternately into a [Sn(PO4)2]4− chain with 1D four-MPR tunnels (Fig. 2a). Two PbO4X polyhedra were edge-shared into a Pb2O6X2 dimer by oxygen atoms (Fig. 2b). The [Sn(PO4)2]4− chains were bridged by the Pb2O6X2 dimers into a 2D layered structure (Fig. 2c and d). In the structure of PbSn(PO4)I (4), the neighboring layers were identical while there was a two-fold axis between the neighboring layers of PbSn(PO4)Br (3). That is, the difference between compounds 3 and 4 is caused by different arrangements of the PbSn(PO4)X layers.
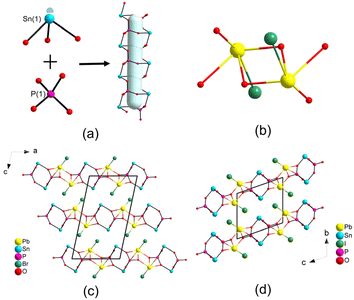 |
| Fig. 2 The SnO3 group, PO4 group and [Sn(PO4)2]4− chain (a), Pb2O6X2 dimer (b), the framework of PbSn(PO4)Br (c) and PbSn(PO4)I (d). | |
Based on the above analysis, we can find that the structural symmetry of PbM(PO4)X decreased from an orthogonal, monoclinic, to a triclinic system when the halogen atom was changed from fluorine to iodine. The F atoms in PbZn(PO4)F coordinated with Pb and Zn simultaneously while the halogen in PbSn(PO4)X can only link with Pb atoms. Due to the high coordination number of Zn, compared with that of Sn, a 3D open framework was formed by the zinc and phosphate polyhedra in PbZn(PO4)F while the SnO3 and PO4 groups could only form 1D [Sn(PO4)2]4− chains in the structure of PbSn(PO4)X. The lead oxyhalide groups in PbZn(PO4)F haven't participated in the expansion of the structural dimension while the PbO4X polyhedra in PbSn(PO4)X bridged the [Sn(PO4)2]4− chains into a 2D layered structure. The interlayer distances are increased from 7.3 and 7.5 to 8.7 Å when the halogen changed from Cl and Br to I. The sharp increase of the interlayer distance of PbSn(PO4)I corresponds to the obvious enhancement of the atomic radius of I from Br.
IR and UV-vis-NIR diffuse reflectance spectra
The IR spectra of compounds 1, 3 and 4 are represented in Fig. S3.† The P–O stretching vibrations fall in the regions of 1086–921, 1074–937 and 1080–964 cm−1, while the bending vibrations fall essentially in the region of 600–400 cm−1.51,52 The UV-vis-NIR diffuse reflectance spectra of compounds 1, 3 and 4 revealed that they exhibited wide transparency ranges from 500 to 2000 nm (Fig. S4†). The cut-off edges of compounds 1, 3 and 4 were 229, 310 and 319 nm, and the corresponding optical band gaps were 4.38, 3.30, and 2.60 eV, respectively. The band gap of PbM(PO4)X decreased with the increase of the atomic number of the halogen element, while the absorption edge of compound 1 was significantly blue shifted due to the incorporation of the d10 TM and the fluorine element.
Thermal stability tests
Thermal stability tests for these three compounds were conducted in the temperatures range of 30–1000 °C. As shown in Fig. S5,† the three compounds started to break down at 660 °C, 565 °C and 554 °C respectively, and their thermal stability decreased with the increase of the atomic weight of halogen atoms. The compounds showed one step of weight loss. The total weight loss of PbZn(PO4)F (1) was 3.8%, corresponding to the partial release of the fluorine element. The total weight losses of compound 3 and 4 were 21.0% and 35.2%, respectively, which can be ascribed to the decomposition of the stannous halides.
SHG characterization
PbZn(PO4)F (1) crystallized in the non-centrosymmetric space group. The sieved (70–100 mesh) PbZn(PO4)F (1) crystal samples were tested under the illumination of a Q-switched Nd:YAG 1064 nm laser to investigate its SHG behaviour. Likewise, the same size of KDP crystal served as a reference. The results revealed that PbZn(PO4)F (1) exhibited a strong frequency doubling intensity, approximately 1.6 times that of the commercial KDP (Fig. 3), exceeding the SHG intensity of many other phosphates, uch as KMg(H2O)PO4 (about 1.14 × KDP),20 Ba3[Al(PO4)3] (about 0.5 × KDP),14 Rb2Zn3(P2O7)2 (1 × KDP),37 K2SrP4O12(0.5 × KDP),15 CsMgPO4·6H2O (1.36 × KDP),25etc. To further reveal the relationship between the SHG effect and the functional units, it is necessary to illustrate the SHG density map of the maximum tensors d33 in the valence band (VB) and the conduction band (CB) of PbZn(PO4)F (Fig. S6†). The SHG effect contributions to the VB were largely accounted for by the Zn-3d, O-2p and F-2p non-bonded electronic states; the SHG contribution in the CB was largely accounted for by the Pb-6p non-occupied orbitals. From the overall SHG density, the SHG contributions of each unit (PO4, PbO2F2 and ZnO4F groups) in PbZn(PO4)F (1) were estimated to be 40.21%, 37.66% and 22.13%, respectively, indicating the synergistic effect of the anionic groups.
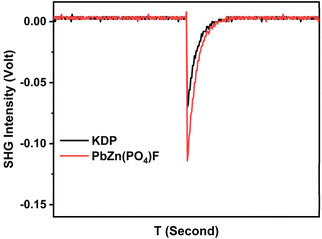 |
| Fig. 3 SHG intensity of sieved PbZn(PO4)F (70–100 mesh) with KDP as the background. | |
Optical property calculation
The optical properties of these compounds, involving SHG and birefringence, were simulated with the help of the DFT method. The simulated band gap structures revealed that compounds 1–3 showed different K points in the lowest CB and highest VB in the first Brillouin zone, indicating that they were all indirect band gap compounds with band gaps of 4.44, 2.57 and 2.78 eV, respectively. The lowest VB and the highest CB of compound 4 both fell at the Z point, that is, a direct band gap compound with a band gap of 2.58 eV (Fig. S7†). Details of the state energies are categorized in Table S3.† Due to the natural limitation of the GGA-PBE functional in the DFT method, the experimental band gap was often a bit higher than the calculated one. Therefore, the scissors of 0, 0.54 and 0.02 eV were adopted for compounds 1, 3 and 4, respectively, in this work.
The partial density of states (PDOS) for PbZn(PO4)F (1) is displayed in Fig. 4a. The low-energy interval −22 to −9 eV in the VB was largely controlled by the Pb-5d, O-2s and F-2s states. The energy interval of −9 to 0 eV was mostly filled by Zn-3d, O-2p and F-2p states. The O-2p nonbonding orbitals governed the top of the VB. The bottom of the CB originated from Pb-6p states. Thus, the band gap of PbZn(PO4)F (1) is controlled by O and Pb atoms. The PDOS plots for compounds 2–4 were comparable (Fig. 4b–d). In the case of PbSn(PO4)Br (3), for example, the lower energy interval of −22 to −10 eV in the VB was strongly influenced by the Pb-5d, O-2s and Br-4s states. The energy level interval of −9 to 0 eV was mostly filled by O-2p, Br-4p and Sn-5s states. The bottom of the CB was mainly formed by Pb-6p and Sn-5p states. The O-2p and Br-4p nonbonding orbitals governed the top of the VB. Thus, the band gap of PbSn(PO4)X is determined by X, O and Pb atoms.
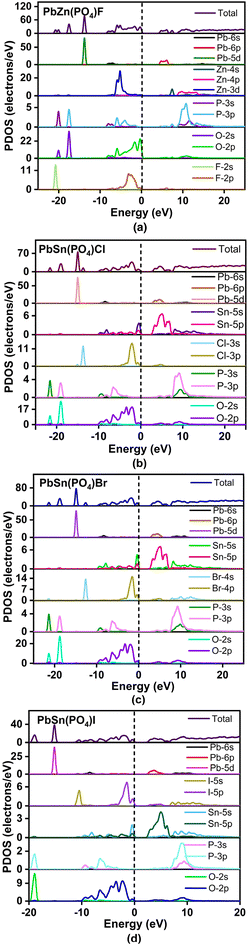 |
| Fig. 4 The PDOS for PbZn(PO4)F (a), PbSn(PO4)Cl (b), PbSn(PO4)Br (c) and PbSn(PO4)I (d). | |
As mentioned previously, compounds 1, 3 and 4 are crystallized in the orthorhombic, monoclinic and triclinic crystal systems, respectively, and they belong to biaxial crystals, that is, the three major permittivity coefficients of the three compounds were not equal, ε1 ≠ ε2 ≠ ε3, and thus the refractive indices were also not equal, n1 ≠ n2 ≠ n3. Here, the birefringence can be obtained by the equation Δn = nmax − nmin (Fig. 5). The anisotropy of PbZn(PO4)F (1) was very weak, with a birefringence of 0.004 at 1064 nm, making it difficult to satisfy the general angular phase matching conditions. However, the small birefringence (less than 0.01) makes PbZn(PO4)F (1) a potential candidate for zero-order waveplates.53 For compounds 3 and 4, the nx and nz curves follow the same direction and are even partially overlapped, but they are far from the ny curves. The birefringence of compound 3 was calculated to be 0.025 at 1064 nm and that of compound 4 was 0.095 at 1064 nm. Thus, the birefringence of PbM(PO4)X increased with increasing atomic number of the halogen element. The birefringence values of some phosphates are presented in Table S4.† Simple phosphates usually have small birefringence, and the introduction of functional units may solve this problem.
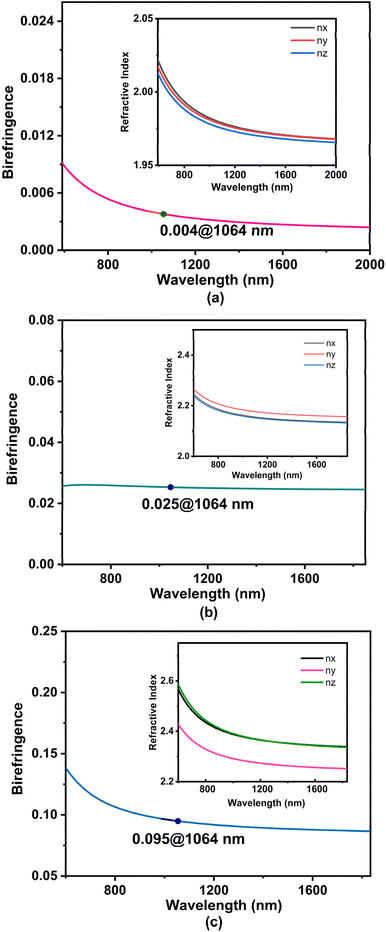 |
| Fig. 5 The birefringence of PbZn(PO4)F (a), PbSn(PO4)Br (b) and PbSn(PO4)I (c). | |
Conclusions
In summary, four new phosphates with a PbM(PO4)X formula, namely, PbZn(PO4)F (1), PbSn(PO4)Cl (2), PbSn(PO4)Br (3) and PbSn(PO4)I (4), were synthesized and characterized successfully. PbZn(PO4)F (1) was crystallized in a polar space group Pna21 and it showed a moderate SHG intensity of about 1.6 × KDP. The 3D structure consisted of a 3D [Zn(PO4)F]2− anionic framework with lead atoms located in the eight-MPR tunnels. Compounds 2–4 crystallized in the CS space group and their layered structures were similar, composed of [Sn(PO4)2]4− chains bridged by Pb2O6X2 dimers, although the crystal symmetry of PbSn(PO4)I (4) was lower than those of the isostructural compounds 2 and 3. In addition to the crystal symmetry, the thermal stability and the band gap of the compounds also decreased with an increase of the atomic number of the halogen. The NCS PbZn(PO4)F (1) was the most stable of these four compounds (660 °C) and featured the largest band gap (4.38 eV). However, the birefringence is increased with an increase of the atomic weight of halogen. Of the title compounds, the highest birefringence of 0.095 at 1064 nm was observed for PbSn(PO4)I (4). This work proved that the halogen elements can effectively regulate the structure and optical properties of oxyhalide compounds.
Conflicts of interest
There are no conflicts to declare.
Acknowledgements
This work was supported by the National Natural Science Foundation of China (Grants No.: 91963105, 22031009 and 21921001).
References
- P. F. Bordui and M. Fejer, Inorganic Crystals for Nonlinear Optical Frequency Conversion, Annu. Rev. Mater. Sci., 1993, 23, 321–379 CrossRef CAS.
- A. R. S. Suresh, D. Jayaraman and a. P. Mani, Review on theoretical aspect of nonlinear optics, Rev. Adv. Mater. Sci., 2012, 30, 175–183 Search PubMed.
- S.-P. Guo, Y. Chi and G.-C. Guo, Recent achievements on middle and far-infrared second-order nonlinear optical materials, Coord. Chem. Rev., 2017, 335, 44–57 CrossRef CAS.
- Y.-N. Zhang, Q.-F. Li, B.-B. Chen, Y.-Z. Lan, J.-W. Cheng and G.-Y. Yang, Na3B6O10(HCOO): an ultraviolet nonlinear optical sodium borate-formate, Inorg. Chem. Front., 2022, 9, 5032–5038 RSC.
- Y. Li, X. Chen and K. M. Ok, KF·B(OH)3: a KBBF-type material with large birefringence and remarkable deep-ultraviolet transparency, Chem. Commun., 2022, 58, 8770–8773 RSC.
- J. Jiao, M. Zhang and S. Pan, Aluminoborates as Nonlinear Optical Materials, Angew. Chem., Int. Ed., 2022, e202217037 Search PubMed.
- C. Wu, L. Lin, X. Jiang, Z. Lin, Z. Huang, M. G. Humphrey, P. S. Halasyamani and C. Zhang, K5(W3O9F4)(IO3): An Efficient Mid-Infrared Nonlinear Optical Compound with High Laser Damage Threshold, Chem. Mater., 2019, 31, 10100–10108 CrossRef CAS.
- J. Chen, C. L. Hu, F. F. Mao, B. P. Yang, X. H. Zhang and J. G. Mao, REI5O14 (RE = Y and Gd): Promising SHG Materials Featuring the Semicircle-Shaped I5O143− Polyiodate Anion, Angew. Chem., Int. Ed., 2019, 58, 11666–11669 CrossRef CAS PubMed.
- C. Wu, G. Wei, X. Jiang, Q. Xu, Z. Lin, Z. Huang, M. G. Humphrey and C. Zhang, Additive-Triggered Polar Polymorph Formation: beta-Sc(IO3)3, a Promising Next-Generation Mid-Infrared Nonlinear Optical Material, Angew. Chem., Int. Ed., 2022, 61, e202208514 CAS.
- J. Chen, Y. Zhang, H. Wu, Z. Hu, J. Wang, Y. Wu and H. Yu, AeMg6Ga6S16 (Ae = Ca, Sr, Ba): The First Double Alkaline-Earth Metal Chalcogenides with Excellent Performances, Adv. Opt. Mater., 2022, 11, 2202147–2202155 CrossRef.
- S.-F. Li, X.-M. Jiang, B.-W. Liu, D. Yan, C.-S. Lin, H.-Y. Zeng and G.-C. Guo, Superpolyhedron-Built Second Harmonic Generation Materials Exhibit Large Mid-Infrared Conversion Efficiencies and High Laser-Induced Damage Thresholds, Chem. Mater., 2017, 29, 1796–1804 CrossRef CAS.
- X. Luo, Z. Li, F. Liang, Y. Guo, Y. Wu, Z. Lin and J. Yao, Synthesis, Structure, and Characterization of Two Mixed-Cation Quaternary Chalcogenides K2BaSnQ4 (Q = S, Se), Inorg. Chem., 2019, 58, 7118–7125 CrossRef CAS PubMed.
- W. Dong, Y. Sun, Q. Yao, Q. Wang, H. Wen, J. Li, X. Xu and J. Wang, Te3O3(PO4)2: a phosphate crystal with large birefringence activated by the highly distorted [TeO5] group and antiparallel [PO4] pseudo-layer, J. Mater. Chem. C, 2020, 8, 9585–9592 RSC.
- V. V. Atuchin, V. G. Kesler, G. Meng and Z. S. Lin, The electronic structure of RbTiOPO4 and the effects of the A-site cation substitution in KTiOPO4-family crystals, J. Phys.: Condens. Matter, 2012, 24, 405503 CrossRef CAS PubMed.
- Z. Bai, L. Liu, L. Zhang, Y. Huang, F. Yuan and Z. Lin, K2SrP4O12: a deep-UV transparent cyclophosphate as a nonlinear optical crystal, Chem. Commun., 2019, 55, 8454–8457 RSC.
- J. L. Xie, Y. H. Zhou, L. H. Li, J. H. Zhang and J. L. Song, A new method for the preparation of a [Sn2(H2PO2)3]Br SHG-active polar crystal via surfactant-induced strategy, Dalton Trans., 2017, 46, 9339–9343 RSC.
- G. Han, Q. Liu, Y. Wang, X. Su, Z. Yang and S. Pan, Experimental and theoretical studies on the linear and nonlinear optical properties of lead phosphate crystals LiPbPO4, Phys. Chem. Chem. Phys., 2016, 18, 19123–19129 RSC.
- Y. Shen, Y. Yang, S. Zhao, B. Zhao, Z. Lin, C. Ji, L. Li, P. Fu, M. Hong and J. Luo, Deep-Ultraviolet Transparent Cs2LiPO4 Exhibits an Unprecedented Second Harmonic Generation, Chem. Mater., 2016, 28, 7110–7116 CrossRef CAS.
- B. L. Wu, C. L. Hu, F. F. Mao, R. L. Tang and J. G. Mao, Highly Polarizable Hg2+ Induced a Strong Second Harmonic Generation Signal and Large Birefringence in LiHgPO4, J. Am. Chem. Soc., 2019, 141, 10188–10192 CrossRef CAS PubMed.
- Z. Bai, C.-L. Hu, L. Liu, L. Zhang, Y. Huang, F. Yuan and Z. Lin, KMg(H2O)PO4: A Deep-Ultraviolet Transparent Nonlinear Optical Material Derived from KTiOPO4, Chem. Mater., 2019, 31, 9540–9545 CrossRef CAS.
- L. Qi, Z. Chen, X. Shi, X. Zhang, Q. Jing, N. Li, Z. Jiang, B. Zhang and M.-H. Lee, A3BBi(P2O7)2 (A = Rb, Cs; B = Pb, Ba): Isovalent Cation Substitution to Sustain Large Second-Harmonic Generation Responses, Chem. Mater., 2020, 32, 8713–8723 CrossRef CAS.
- J. Wang, B. Xiong, H. Wu, H. Yu, Z. Hu, J. Wang and Y. Wu, Bi32Cd3P10O76: a new congruently melting nonlinear optical crystal with a large SHG response and a wide transparent region, Inorg. Chem. Front., 2021, 8, 344–351 RSC.
- J. Lv, Y. Qian, Q. Jing, X. Wang, M.-H. Lee and Z. Chen, Two Metal Phosphate Nonlinear Optical Materials Simultaneously Exhibiting Ultraviolet Transparence and a Large Birefringence, Chem. Mater., 2022, 34, 5919–5927 CrossRef CAS.
- F. Xu, G. Peng, C. Lin, D. Zhao, B. Li, G. Zhang, S. Yang and N. Ye, Na3Sc2(PO4)2F3: rational design and synthesis of an alkali rare-earth phosphate fluoride as an ultraviolet nonlinear optical crystal with an enlarged birefringence, J. Mater. Chem. C, 2020, 8, 4965–4972 RSC.
- Y. Zhou, L. Cao, C. Lin, M. Luo, T. Yan, N. Ye and W. Cheng, AMgPO4·6H2O (A = Rb, Cs): strong SHG responses originated from orderly PO4 groups, J. Mater. Chem. C, 2016, 4, 9219–9226 RSC.
- H. Yu, J. Young, H. Wu, W. Zhang, J. M. Rondinelli and P. S. Halasyamani, M4Mg4(P2O7)3 (M = K, Rb): Structural Engineering of Pyrophosphates for Nonlinear Optical Applications, Chem. Mater., 2017, 29, 1845–1855 CrossRef CAS.
- Y. Deng, L. Huang, X. Dong, L. Wang, K. M. Ok, H. Zeng, Z. Lin and G. Zou, K2Sb(P2O7)F: Cairo Pentagonal Layer with Bifunctional Genes Reveal Optical Performance, Angew. Chem., Int. Ed., 2020, 59, 21151–21156 CrossRef CAS PubMed.
- Y. Li, J. Luo and S. Zhao, Local Polarity-Induced Assembly of Second-Order Nonlinear Optical Materials, Acc. Chem. Res., 2022, 55, 3460–3469 CrossRef CAS PubMed.
- C. Wu, G. Yang, M. G. Humphrey and C. Zhang, Recent advances in ultraviolet and deep-ultraviolet second-order nonlinear optical crystals, Coord. Chem. Rev., 2018, 375, 459–488 CrossRef CAS.
- H. Yu, W. Zhang, J. Young, J. M. Rondinelli and P. S. Halasyamani, Bidenticity-Enhanced Second Harmonic Generation from Pb Chelation in Pb3Mg3TeP2O14, J. Am. Chem. Soc., 2016, 138, 88–91 CrossRef CAS PubMed.
- F. Yang, L. Wang, L. Huang and G. Zou, The study of structure evolvement of KTiOPO4 family and their nonlinear optical properties, Coord. Chem. Rev., 2020, 423, 213491 CrossRef CAS.
- J. Lu, J. N. Yue, L. Xiong, W. K. Zhang, L. Chen and L. M. Wu, Uniform Alignment of Non-π-Conjugated Species Enhances Deep Ultraviolet Optical Nonlinearity, J. Am. Chem. Soc., 2019, 141, 8093–8097 CrossRef CAS PubMed.
- Q. Q. Chen, C. L. Hu, L. J. Yao, J. Chen, M. Y. Cao, B. X. Li and J. G. Mao, Cd2(IO3)(PO4) and Cd1.62Mg0.38(IO3)(PO4): metal iodate-phosphates with large SHG responses and wide band gaps, Chem. Commun., 2022, 58, 7694–7697 RSC.
- T. Yu, L. Xiong, X. Liu, Y.-C. Yang, Z. Lin, L.-M. Wu and L. Chen, AZn(PO3)3 (A = K, Rb): Deep-Ultraviolet Nonlinear Optical Phosphates Derived from Synergy of a Unique [ZnO6] Octahedron and a [PO3]∞ Chain, Cryst. Growth Des., 2021, 21, 2445–2452 CrossRef CAS.
- L. Zhou, S. Pan, X. Dong, H. Yu, H. Wu, F. Zhang and Z. Zhou, Na2Cd7B8O20: a new noncentrosymmetric compound with special [B3O7] units, CrystEngComm, 2013, 15, 3412–3416 RSC.
- X. Pan, M. Wen, G. He, H. Li and D. Jia, Syntheses, structures and properties of metal phosphates Pb2Mg(PO4)2, Pb4Zn8(PO4)8 and alpha-BaZn2(PO4)2, Dalton Trans., 2017, 46, 16034–16040 RSC.
- Z. Song, H. Yu, H. Wu, Z. Hu, J. Wang and Y. Wu, Syntheses, structures and characterization of non-centrosymmetric Rb2Zn3(P2O7)2 and centrosymmetric Cs2M3(P2O7)2 (M = Zn and Mg), Inorg. Chem. Front., 2020, 7, 3482–3490 RSC.
- H. Liu, H. Wu, H. Yu, Z. Hu, J. Wang and Y. Wu, K2ZnMoP2O10: a novel nonlinear optical molybdophosphate with a strong second harmonic generation response and moderate birefringence, J. Mater. Chem. C, 2021, 9, 15321–15328 RSC.
- H. Yu, W. Zhang, J. Young, J. M. Rondinelli and P. S. Halasyamani, Design and Synthesis of the Beryllium-Free Deep-Ultraviolet Nonlinear Optical Material Ba3Zn(B5O10)PO4, Adv. Mater., 2015, 27, 7380–7385 CrossRef CAS PubMed.
- Q. Wei, C. He, K. Wang, X. F. Duan, X. T. An, J. H. Li and G. M. Wang, Sb6O7(SO4)2 : A Promising Ultraviolet Nonlinear Optical Material with an Enhanced Second-Harmonic-Generation Response Activated by SbIII Lone-Pair Stereoactivity, Chem. – Eur. J., 2021, 27, 5880–5884 CrossRef CAS PubMed.
- Y. J. Jia, X. Zhang, Y. G. Chen, X. Jiang, J. N. Song, Z. Lin and X. M. Zhang, PbBi(SeO3)2F and Pb2Bi(SeO3)2Cl3: Coexistence of Three Kinds of Stereochemically Active Lone-Pair Cations Exhibiting Excellent Nonlinear Optical Properties, Inorg. Chem., 2022, 61, 15368–15376 CrossRef CAS PubMed.
- Y. Yang, Y. Qiu, P. Gong, L. Kang, G. Song, X. Liu, J. Sun and Z. Lin, Lone-Pair Enhanced Birefringence in an Alkaline-Earth Metal Tin(II) Phosphate BaSn2(PO4)2, Chem. – Eur. J., 2019, 25, 5648–5651 CrossRef CAS PubMed.
- T. Zheng, Q. Wang, J. Ren, L. Cao, L. Huang, D. Gao, J. Bi and G. Zou, Halogen regulation triggers structural transformation from centrosymmetric to noncentrosymmetric switches in tin phosphate halides Sn2PO4X (X = F, Cl), Inorg. Chem. Front., 2022, 9, 4705–4713 RSC.
- J. Guo, A. Tudi, S. Han, Z. Yang and S. Pan, Sn2PO4I: An Excellent Birefringent Material with Giant Optical Anisotropy in Non π-Conjugated Phosphate, Angew. Chem., Int. Ed., 2021, 60, 24901–24904 CrossRef CAS PubMed.
- G. M. Sheldrick, Crystal structure refinement with SHELXL, Acta Crystallogr., Sect. C: Struct. Chem., 2015, 71, 3–8 Search PubMed.
- A. L. Spek, Single-crystal structure validation with the program PLATON, J. Appl. Crystallogr., 2003, 36, 7–13 CrossRef CAS.
- F. M. P. Kubelka, An Article on Optics of Paint Layers, Z. Tech. Phys., 1931, 12, 886–892 Search PubMed.
- S. K. Kurtz and T. T. Perry, A Powder Technique for the Evaluation of Nonlinear Optical Materials, J. Appl. Phys., 1968, 39, 3798–3813 CrossRef CAS.
- M. O. K. N. Brese, Bond-valence parameters for solids, Acta Crystallogr., Sect. B: Struct. Sci., 1991, 47, 192–197 CrossRef.
- D. A. I. Brown, Bond–valence parameters obtained from a systematic analysis of the Inorganic Crystal Structure Database, Acta Crystallogr., Sect. B: Struct. Sci., 1985, 41, 244–247 CrossRef.
- D. Balaji, T. R. Mandlimath, J. Chen, Y. Matsushita and S. P. Kumar, Langbeinite Phosphates KPbM2(PO4)3 (M = Cr, Fe): Synthesis, Structure, Thermal Expansion, and Magnetic Properties Investigation, Inorg. Chem., 2020, 59, 13245–13253 CrossRef CAS PubMed.
- M. Wen, H. Wu and X. Wu, Influence of Cation on the Anion Frameworks and Properties of Four Lead Phosphates, A2PbBi2(PO4)2(P2O7) (A = Rb, Cs) and A2PbP2O7 (A = K, Rb), Inorg. Chem., 2020, 59, 2945–2951 CrossRef CAS PubMed.
- M. Cheng, W. Jin, Z. Yang and S. Pan, Cs3B3O3F6 with a Deep-Ultraviolet Cutoff Edge and a Suitable Birefringence as the Potential Zero-Order Waveplate Material, Inorg. Chem., 2020, 59, 13014–13018 CrossRef CAS PubMed.
Footnote |
† Electronic supplementary information (ESI) available: Experimental methods, crystal data, selected bond distances, PXRD patterns, IR and UV-vis-NIR diffuse reflectance spectra, thermal stability curves, band structures and SHG density map. CCDC 2237156–2237159. For ESI and crystallographic data in CIF or other electronic format see DOI: https://doi.org/10.1039/d3qi00230f |
|
This journal is © the Partner Organisations 2023 |