DOI:
10.1039/D2QI02671F
(Research Article)
Inorg. Chem. Front., 2023,
10, 2189-2196
Pyridyl-containing graphdiyne stabilizes sub-2 nm ultrasmall copper nanoclusters for the electrochemical reduction of CO2†
Received
15th December 2022
, Accepted 4th March 2023
First published on 6th March 2023
Abstract
Developing novel carbonaceous materials with definite chemical structures is conducive to understanding structure–property relationships and expanding their applications in supported metal catalysts. Herein, a brand-new pyridine-substituted graphdiyne (Py-GDY) is synthesized through the cross-coupling of 1,3,5-triethynyl-2,4,6-tris(4-pyridyl)benzene, and further applied as a promising carrier in electrocatalysis. Thanks to the precisely introduced pyridyl groups, strong metal–support interaction between the confined Cu species and Py-GDY is desirably obtained, resulting in uniformly dispersed Cu sub-nanoclusters (<2 nm) (Py-GDY-Cu). Conversely, the Cu size increased dramatically when the pyridyl group of Py-GDY was replaced by the phenyl group (Ph-GDY-Cu). In a proof-of-concept demonstration of the electrochemical CO2 reduction reaction, Py-GDY-Cu is found to produce CH4 preferentially to Ph-GDY-Cu, owing to the favorable sub-nanocluster size. As a result, an optimum CH4 faradaic efficiency of 58% is achieved on Py-GDY-Cu, which shows a 1.6-fold enhancement compared with that of Ph-GDY-Cu. This work broadens the scope of carbonaceous materials for rational metal species immobilization toward efficient catalysis.
Introduction
Catalysis not only plays a vital role in the chemical industry but also attracts great attention in the field of fundamental research. Heterogeneous metal catalysts are widely used in diverse heterogeneous catalysis processes. Their supporting materials often determine the performance of metal catalysts by regulating their shapes, morphologies, sizes, and chemical states.1–3 Carbonaceous materials are a family of supporting materials commonly used in the energy-related research field, and tremendous effort has been devoted to expanding the library of carbonaceous materials.4,5 Heteroatom-doped carbon materials provide a representative platform for supporting metal catalysts and increasing the dispersion of metal species through strong metal–support interaction (MSI), which is expected to affect the catalytic activity.6–8 Nevertheless, the current doping strategies have long been championed by hydrothermal or high-temperature calcination treatment, in which the dopant types and the homogeneity of heteroatoms in carbon materials are difficult to control, putting obstacles in the way of understanding the structure–property relationships.9,10 Hence, developing heteroatom-doped carbon materials with well-defined chemical structures is highly desirable and remains a challenge in the controllable synthesis of carbon-supported metal catalysts.
Graphdiyne (GDY) has emerged as a new two-dimensional (2D) carbon allotrope prepared via the cross-coupling reaction of hexaethynylbenzene.11 With a conjugated structure composed of benzene rings and diacetylenic linkages (–C
C–C
C–), GDY provides defined and uniform anchoring sites for metal atoms.12–14 A series of single-atom catalysts like Fe, Ni, Cu, Mo, Pd, Pt, Ru, Rh etc. has been synthesized on GDY.12–20 MoO3 nanoparticles, CuO nanoclusters, and oxidized iridium quantum dots are also successfully supported on GDY.21–23 The metal atoms are stabilized by the interaction between the d orbitals of metal atoms and the π/π* orbitals of carbon–carbon triple bonds (–C
C–). In addition, taking advantage of the bottom-up synthetic strategy, tens of GDY analogues with desired structures have been realized by precisely introducing heteroatoms (B, N, and S) or functional groups (–F, –Cl, and –CN) into the corresponding monomers.24–29 This makes GDY analogues an ideal platform for the precise synthesis of doped carbon materials to support metal catalysts. By the rational design of GDY analogues with functional groups, the MSI could be readily manipulated, which may in turn affect the sizes, morphologies, and chemical states of the supported metal catalysts.
Pyridyl groups have been widely used as coordinating ligands in various metal complexes.30 Adopting the synthetic strategy of GDY, pyridyl groups could be uniformly incorporated into the 2D network of a GDY analogue. The pyridyl groups on the GDY analogue may play a pivotal role in the dispersion of metal atoms via strong metal–nitrogen interaction, thus facilitating the construction of nanosized metal catalysts.
Herein, a new pyridine-substituted GDY (Py-GDY; Fig. 1) is successfully synthesized via the cross-coupling reaction of 1,3,5-triethynyl-2,4,6-tris(4-pyridyl)benzene (TPyB; Fig. 1). Owing to the merits of the introduced pyridyl group, the strong interaction between the Cu species and Py-GDY leads to the formation of uniformly distributed sub-2 nm ultrasmall Cu clusters (Py-GDY-Cu; Fig. 1). As a comparison, large and uneven Cu nanoparticles were obtained on the other two GDYs, Py-Ph-GDY and Ph-GDY (see their structures in Fig. 1) with a half content of pyridyl groups and without pyridine groups, respectively. In the electrochemical CO2 reduction reaction, Py-GDY-Cu showed the highest faradaic efficiency of 58% for CH4 at −1.0 V vs. a reversible hydrogen electrode (RHE) in 1.0 M KOH, surpassing that of the uneven Cu nanoparticles on Py-Ph-GDY and Ph-GDY. The favorable selectivity for CO2-to-CH4 conversion over Py-GDY-Cu is due to the ultrasmall size of the Cu catalyst originating from the presence of coordinating pyridyl groups in the GDY material. The present work not only broadens the scope of the GDY library but also highlights a compelling support material to immobilize metal catalysts toward improved catalysis.
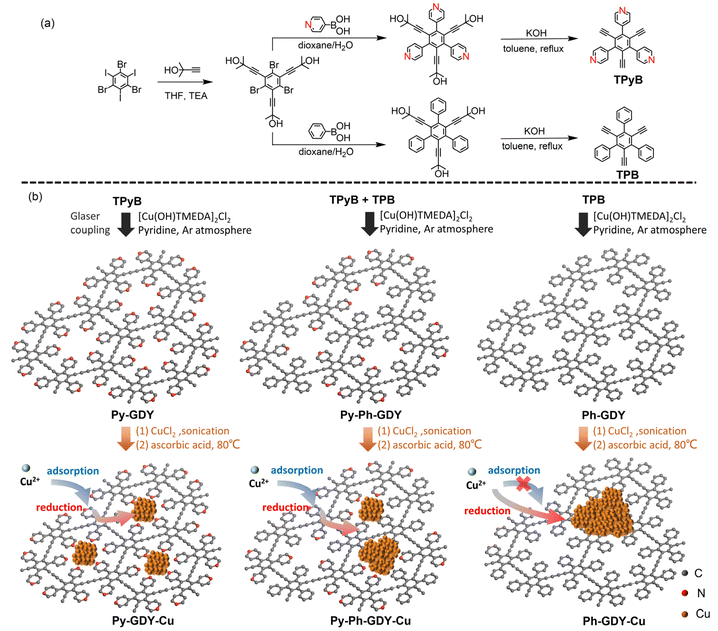 |
| Fig. 1 (a) Synthetic diagram of TPyB and TPB. (b) Schematic illustration for the preparation of Py-GDY, Py-Ph-GDY, Ph-GDY, Py-GDY-Cu, Py-Ph-GDY-Cu, Ph-GDY-Cu. | |
Results and discussion
Synthesis and characterization
TPyB was prepared from 1,3,5-tribromo-2,4,6-triiodobezene in three steps: (i) the Sonogashira reaction; (ii) the Suzuki coupling reaction; and (iii) the base-promoted deprotection reaction. The synthetic procedures are shown in Fig. 1a. All these compounds were thoroughly characterized using 1H-NMR, 13C-NMR spectroscopy, and high-resolution mass spectroscopy (Fig. S1–S10†). Using the Cu-catalyzed Glaser coupling reaction of TPyB, Py-GDY was produced with a porous framework and evenly distributed pyridyl groups, as shown in Fig. 1b. The transmission electron microscopy (TEM) image of Py-GDY (Fig. 2a) reveals a lamellar morphology of Py-GDY. Elements of C and N were uniformly distributed, as depicted by the energy dispersive spectroscopy (EDS) mapping images. The X-ray diffraction (XRD) pattern in Fig. S11† displays a broad peak at 21° assigned to the in-plane periodicity, indicating that Py-GDY is amorphous but still shows 2D ordering.31
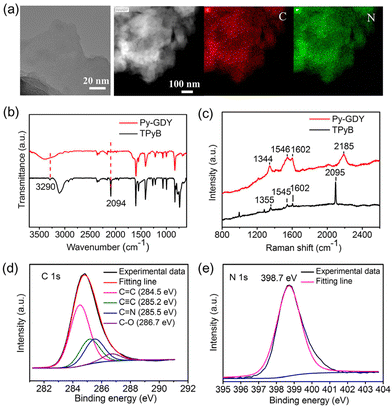 |
| Fig. 2 (a) TEM and EDS mapping images of Py-GDY. (b) FTIR spectra of Py-GDY and TPyB. (c) Raman spectra of Py-GDY and TPyB. (d) C 1s XPS spectrum, and (e) N 1s XPS spectrum of Py-GDY. | |
The structure of Py-GDY was further investigated by the Fourier transform infrared spectroscopy (FTIR), Raman spectroscopy, and X-ray photoelectron spectroscopy (XPS). By comparison of the FTIR spectra of TPyB and Py-GDY (Fig. 2b), two IR bands at 3290 and 2094 cm−1 are exclusively observed for TPyB but not for Py-GDY, and these two bands are respectively due to the C–H stretching vibration of C
C–H and the C
C stretching vibration.26 Apparently, the terminal acetylenic groups of TPyB are converted to, in principle, the diacetylenic linkages via the Glaser coupling reaction, leading to the formation of Py-GDY. The Raman spectrum (Fig. 2c) of Py-GDY displays four bands at 1344, 1546, 1602, and 2185 cm−1. The band at 2185 cm−1 is attributed to the stretching vibration of conjugated diyne links (–C
C–C
C–),11 verifying the cross-coupling of the terminal acetylenic groups. For XPS analysis, the C 1s peak of Py-GDY (Fig. 2d) is deconvoluted into four peaks of C
C at 284.5 eV, C
C at 285.2 eV, C
N at 285.5 eV, and C–O at 286.7 eV. The N 1s spectrum of Py-GDY (Fig. 2e) depicts only one peak at 398.7 eV, assigned to the N atom of pyridyl groups.9 All the results above are in good agreement with the proposed structure of Py-GDY.
Pyridyl groups are widely used in coordination chemistry for their high tendency to form metal complexes.30 With its porous structure and abundant pyridyl groups, Py-GDY can be a proper support for metal catalysts. To systematically examine the effects of pyridyl groups on stabilizing metal catalysts, we also synthesized another two GDY analogues, Py-Ph-GDY and Ph-GDY, as shown in Fig. 1b. Ph-GDY was prepared by the coupling of 1,3,5-triethynyl-2,4,6-triphenyl-benzene (TPB) according to the literature method.32 Compared with Py-GDY, all the pyridyl groups were replaced by phenyl groups in Ph-GDY. To further tune the content of pyridyl groups, a mixture of TPyB and TPB with a molar ratio of 1
:
1 was used to produce Py-Ph-GDY. Ph-GDY and Py-Ph-GDY were also characterized by FTIR, Raman, and XPS (Fig. S12–S15†). The elemental analysis results show the C/N atomic ratios are 7.7 and 16.6 for Py-GDY and Py-Ph-GDY, which are close to the values of 9 and 19 calculated from their ideal chemical structures.
After preparing these three GDY analogues, the supported Cu nanocatalysts were further synthesized to investigate the effect of the pyridyl group on the size distribution of the Cu catalysts. In general, Py-GDY, Py-Ph-GDY, and Ph-GDY were respectively dispersed in a CuCl2 solution by sonication, and then ascorbic acid was added to reduce Cu2+ into Cu nanoclusters/nanoparticles.33 The resulting products are accordingly named Py-GDY-Cu, Py-Ph-GDY-Cu, and Ph-GDY-Cu. According to the EDS spectra (Fig. S16†), Cu is successfully incorporated into Py-GDY-Cu, Py-Ph-GDY-Cu, and Ph-GDY-Cu. But the morphologies of Cu change over the three substrates due to their different MSI, as shown schematically in Fig. 3a. No Cu particles are observed in the TEM image of Py-GDY-Cu (Fig. 3b). The aberration-corrected high-angle annular dark-field scanning transmission electron microscopy (HAADF-STEM) image (Fig. 3c) verifies that Cu nanoclusters with sizes smaller than 2 nm are well distributed throughout the Py-GDY support, in line with the EDS spectrum and maps of C, N, and Cu shown in Fig. S16† and Fig. 3d. For Py-Ph-GDY-Cu, Cu nanoparticles with sizes of about 10 nm (circled in red in Fig. 3e) are found; besides, Cu nanoclusters with sizes around 1–2 nm are also present on the surface of Py-Ph-GDY-Cu as revealed by the aberration-corrected HAADF-STEM image (Fig. 3f). In contrast, the TEM image (Fig. 3h) and the HAADF-STEM image (Fig. 3i) of Ph-GDY-Cu disclose the presence of Cu nanoparticles around 100 nm. The distribution of the Cu element is not even as shown in the EDS image of Cu (Fig. 3j). According to the inductively coupled plasma atomic emission spectrometry measurements, the weight percent of Cu was 4.3 wt%, 2.7 wt%, and 2.0 wt% for Py-GDY-Cu, Py-Ph-GDY-Cu, and Ph-GDY-Cu respectively. Both high loading and uniform distribution of Cu nanoclusters are achieved using Py-GDY as the support. These phenomena arise from the fact that pyridyl groups on Py-GDY-Cu can adsorb Cu2+ and provide abundant anchoring sites for the nucleation and growth of Cu nanoclusters. Since phenyl groups are not able to coordinate with Cu atoms, the regular structure composed of both pyridyl and phenyl groups shows a decreased ability to control the size of Cu on the Py-Ph-GDY-Cu. Due to the poor interaction between Ph-GDY and Cu, Cu easily aggregates into large nanoparticles. The XRD measurement was used to determine the structures of these three samples. In Fig. S17,† no peak of Cu is detected in the XRD patterns of Py-GDY-Cu and Py-Ph-GDY-Cu, mainly due to the rather small sizes of amorphous Cu nanoclusters.13,19Ph-GDY-Cu shows three diffraction peaks at 36.49°, 43.25° and 50.37°, due to the (111) crystal plane of Cu2O and the (111), (200) crystal planes of metallic Cu. The existence of Cu2O is due to the oxidation of Cu atoms under an ambient atmosphere.33
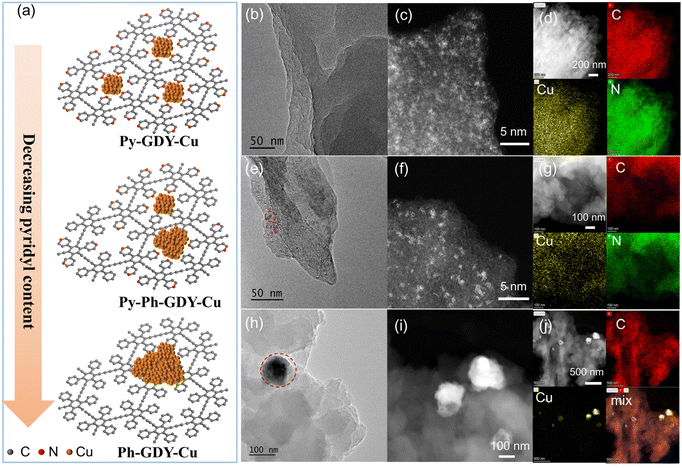 |
| Fig. 3 (a) Schematic illustration of Py-GDY-Cu, Py-Ph-GDY-Cu, and Ph-GDY-Cu. (b) TEM image, (c) aberration-corrected HAADF-STEM image, and (d) EDS mapping images of Py-GDY-Cu. (e) TEM image, (f) aberration-corrected HAADF-STEM image, and (g) EDS mapping images of Py-Ph-GDY-Cu. (h) TEM image, (i) HAADF-STEM image, and (j) EDS mapping images of Ph-GDY-Cu. | |
Further insights into the structural and electronic information on the three samples were obtained from the XPS measurements. In Fig. 4a, the characteristic peaks of C 1s and Cu 2p can be found in all three samples, and only Py-GDY-Cu and Py-Ph-GDY-Cu possess the signal of N 1s. As shown in Fig. 4b, the Cu 2p3/2 peaks around 932.9 eV and Cu 2p1/2 peaks around 952.7 eV in the Cu 2p XPS spectra are associated with Cu0 or Cu+.34 No satellite peaks of Cu2+ are observed. The Cu LMM Auger spectra of these samples are shown in Fig. 4c. Py-GDY-Cu displays the most dominant Cu+ peak, while Ph-GDY-Cu has the largest fraction of Cu0.34 This may result from the fact that small Cu nanoclusters are more easily oxidized or that Cu atoms coordinated with pyridyl groups are prone to oxidation. The N 1s spectra in Fig. 4d demonstrate the interaction between the pyridyl groups and Cu atoms. The peak at 398.7 eV is attributed to the N 1s peak of free pyridyl groups of Py-GDY. After decorating Cu nanoclusters or nanoparticles on Py-GDY and Py-Ph-GDY, a new peak at 399.9 eV appears, indicating that a part of the pyridyl groups coordinates with Cu atoms, leading to a shift in the N 1s peak in the higher energy direction.
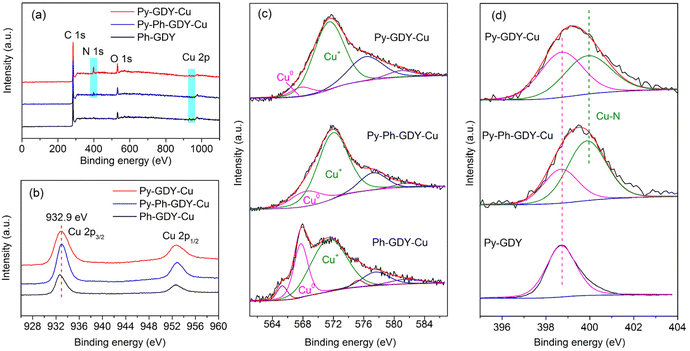 |
| Fig. 4 (a) XPS spectra, (b) Cu 2p spectra, (c) Cu LMM Auger spectra of Py-GDY-Cu, Py-Ph-GDY-Cu, and Ph-GDY-Cu. (d) N 1s spectra of Py-GDY-Cu, Py-Ph-GDY-Cu and Py-GDY. | |
Electrochemical reduction of CO2
The electrochemical reduction of CO2 has emerged as a promising method for CO2 conversion. Cu materials show a unique capability of catalyzing the electrochemical conversion of CO2 to various hydrocarbons and alcohols.35 Improved activity and selectivity of Cu catalysts toward specific products is in high demand. Previous studies show that the “catalytic particle size effect” significantly influences the activity and selectivity of Cu catalysts.13,36,37 In this work, the Cu nanoparticles ranged from sub-2 to 100 nm by tuning the pyridyl content in the supporting GDY materials. In particular, Py-GDY-Cu has pushed the Cu particle size to a sub-2 nm level, where the quantum effect, also known as the “catalytic finite-size effect”, becomes noticeable.38 With the three supported Cu catalysts in hand, we surveyed their performance in the electrochemical CO2 reduction reaction. The experiments were performed using a flow cell reactor, where a CO2 stream and the commonly used 1.0 M KOH aqueous electrolyte were fed separately into the electrochemical cell through the two sides of the gas-diffusing electrode. Before the electrolysis experiments, electrochemical conditioning was applied for all three catalysts by cyclic voltammetry scanning from 0.5 V to −0.5 V vs. RHE for 60 cycles. After that, steady-state chronoamperometry measurements were conducted to evaluate the products of CO2 reduction.
As shown in Fig. 5a–c, the electrolysis products consist of CO, CH4, HCOOH, C2H4, CH3CH2OH, CH3COOH, and H2. The faradaic efficiency (FE) of CO decreased with applying more negative potentials due to the conversion of CO into other hydrocarbons.39Ph-GDY-Cu showed a higher FE of C2 products (C2H4, CH3CH2OH, CH3COOH) than Py-Ph-GDY-Cu and Py-GDY-Cu at all the applied potentials from −0.8 to −1.2 V vs. RHE, as shown in Fig. S18.† The highest FE of 31% for C2 products was achieved by Ph-GDY-Cu at −1.0 V vs. RHE. The highest FEs of C2 products for Py-Ph-GDY-Cu and Py-GDY-Cu were 23% and 21% at −0.8 V vs. RHE. As expected, the selectivity of C2 products increases as the size of the Cu particles increases because the large Cu particles favor the dimerization of *CO.13 CH4 dominates the products under most of the tested potentials. As shown in Fig. 5a, Py-GDY-Cu leads to a higher selectivity of CH4 than Py-Ph-GDY-Cu and Ph-GDY-Cu from −0.8 V to −1.1 V vs. RHE. The highest FE of CH4 reaches 58% for Py-GDY-Cu at −1.0 V vs. RHE, while Py-Ph-GDY-Cu and Ph-GDY-Cu show CH4 FEs of 42% and 37% at the same potential. On the other hand, Py-Ph-GDY-Cu and Ph-GDY-Cu show increased selectivity toward CH4 with applying more negative potentials; this is due to the increased *H coverage and decreased *CO coverage at more cathodic potentials, which suppresses the generation of C2 products and facilitates the production of CH4.40,41 The CH4 selectivity of Py-Ph-GDY-Cu and Ph-GDY-Cu at −1.2 V vs. RHE is still slightly lower than that of Py-GDY-Cu at −1.0 V vs. RHE. In contrast, Py-GDY-Cu displays decreased FE of CH4 and increased FE of H2 at −1.1 V and −1.2 V vs. RHE, which is very likely caused by the fact that Py-GDY-Cu is more hydrophilic than the other two catalysts, as indicated by their water contact angles in Fig. S19.† At −1.0 V vs. RHE, the partial current densities of CH4 (Fig. 5e) were 105 mA cm−2, 75 mA cm−2, and 54 mA cm−2 for Py-GDY-Cu, Py-Ph-GDY-Cu, and Ph-GDY-Cu, respectively. Previous studies revealed that Cu+ species could promote the generation of C2 products42,43 while small-size Cu catalysts, such as sub-nanometric Cu clusters, favor the production of CH4.13,36 Which factor dictates the production selectivity of Py-GDY-Cu, Py-Ph-GDY-Cu and Ph-GDY-Cu? In our case, Py-GDY-Cu with the largest content of Cu+ among these three samples displayed the highest CH4 selectivity. Therefore, the product selectivity for our catalysts is not controlled by the Cu oxidation state. In contrast, the size effect could reasonably explain the product selectivity. Small Cu nanoclusters on Py-GDY-Cu prefer producing CH4 over larger Cu aggregates on Py-Ph-GDY-Cu and Ph-GDY-Cu, in line with the previous works.13,36
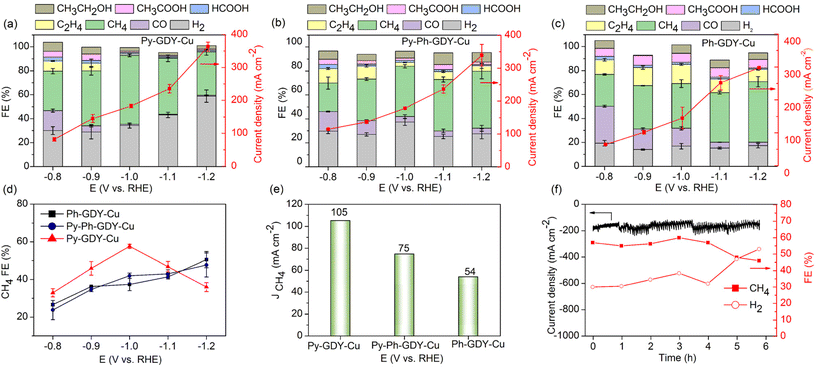 |
| Fig. 5 CO2 electroreduction performances of (a) Py-GDY-Cu, (b) Py-Ph-GDY-Cu, (c) Ph-GDY-Cu in 1.0 M KOH. (d) FE of CH4 at −0.8 V to −1.2 V vs. RHE. (e) Partial current density of CH4 at −1.0 V vs. RHE. (f) Stability test of Py-GDY-Cu and the FE of CH4, H2 measured every 1 h at −1.0 V vs. RHE. | |
The stability of Py-GDY-Cu was examined at the potential of −1.0 V vs. RHE in the 1.0 M KOH electrolyte. In Fig. 5f, no evident decay in the current density is observed after 6 h electrolysis, although some fluctuations exist during the test. The FE of CH4 is 57% at the beginning and declines to 46% within 6 h, while the FE of H2 increases from 30% to 53%, as shown in Fig. 5f. The change in FE is mainly caused by the flooding of the gas diffusing layer and the salt accumulation in the gas chamber during the electrolysis.44 The structure of Py-GDY-Cu after 6 h electrolysis was characterized by TEM and XRD. As shown in Fig. S20,† no XRD peaks of Cu or CuO are found, and only the peaks ascribed to KHCO3 appear. The aberration-corrected HAADF-STEM image (Fig. S21†) shows Cu nanoclusters retained on the surface of Py-GDY-Cu. These results indicate good stability of Cu nanoclusters on Py-GDY toward the electrochemical reduction of CO2.
Conclusions
In summary, we have prepared a pyridyl-containing graphdiyne, Py-GDY, with evenly distributed pyridyl groups and uniform pores on the conjugated networks. The abundant pyridyl groups and porous structure of Py-GDY provide coordinating sites for anchoring metal atoms, and Py-GDY-Cu with sub-2 nm ultrasmall Cu clusters is realized using this novel supporting material. Py-Ph-GDY-Cu with decreased content of pyridyl groups possesses both Cu nanoclusters and nanoparticles (about 10 nm). For Ph-GDY-Cu, larger and uneven Cu nanoparticles are present. These results indicate the importance of pyridyl groups to restrict the aggregation of Cu and to facilitate the generation of Cu nanoclusters. Regarding the electrochemical reduction of CO2, Py-GDY-Cu exhibits the highest FE of 58% for CH4 at −1.0 V vs. RHE in 1.0 M KOH, which is more significant than the highest CH4 FE obtained at −1.2 V vs. RHE by Py-Ph-GDY-Cu and Ph-GDY-Cu. The partial current densities of CH4 at −1.0 V vs. RHE were 105 mA cm−2, 75 mA cm−2, and 54 mA cm−2 for Py-GDY-Cu, Py-Ph-GDY-Cu and Ph-GDY-Cu, respectively. The enhanced selectivity of CH4 for Py-GDY-Cu is attributed to the small sizes of Cu nanoclusters stabilized by the pyridyl groups. Notably, the sub-2 nm ultrasmall Cu clusters of Py-GDY-Cu do not aggregate into large nanoparticles during CO2 reduction. Overall, the precise installation of pyridyl groups into carbon-rich materials has been successfully illustrated by taking advantage of the bottom-up wet chemistry synthesis method of graphdiynes. The strong coordination pyridyl groups play a significant role in stabilizing ultrasmall Cu nanoclusters. Our strategy paves the way for synthesizing well-defined nitrogen-containing carbon materials and lays a foundation for the synthesis of ultrasmall metal nanoclusters.
Author contributions
L. D. conceived the project and designed the experiments. H. D. carried out the experiments and the electrochemical measurements. H. Z., T. S., L. G., C. H., contributed to TEM characterization. S. W., H. L., and H. X. contributed to the synthesis of several compounds and the Raman characterization. H. D., H. Z., and L. D. wrote the manuscript. All authors commented on the manuscript.
Conflicts of interest
There are no conflicts to declare.
Acknowledgements
This work is supported by the National Natural Science Foundation of China (22179057 and 21790051), Stable Support Plan Program of Shenzhen Natural Science Fund (20200925152742003) and Educational Commission of Guangdong Province (2020KTSCX121).
Notes and references
- Y. Wang, H. Su, Y. He, L. Li, S. Zhu, H. Shen, P. Xie, X. Fu, G. Zhou, C. Feng, D. Zhao, F. Xiao, X. Zhu, Y. Zeng, M. Shao, S. Chen, G. Wu, J. Zeng and C. Wang, Advanced electrocatalysts with single-metal-atom active sites, Chem. Rev., 2020, 120, 12217–12314 CrossRef CAS PubMed.
- L. Liu and A. Corma, Metal catalysts for heterogeneous catalysis: from single atoms to nanoclusters and nanoparticles, Chem. Rev., 2018, 118, 4981–5079 CrossRef CAS PubMed.
- Z. Li, S. Ji, Y. Liu, X. Cao, S. Tian, Y. Chen, Z. Niu and Y. Li, Well-defined materials for heterogeneous catalysis: From nanoparticles to isolated single-atom sites, Chem. Rev., 2020, 120, 623–682 CrossRef CAS PubMed.
- A. Han, B. Wang, A. Kumar, Y. Qin, J. Jin, X. Wang, C. Yang, B. Dong, Y. Jia, J. Liu and X. Sun, Recent advances for MOF-derived carbon-supported single-atom catalysts, Small Methods, 2019, 3, 1800471 CrossRef.
- N. M. Julkapli and S. Bagheri, Graphene supported heterogeneous catalysts: An overview, Int. J. Hydrogen Energy, 2015, 40, 948–979 CrossRef CAS.
- C.-L. Yang, L.-N. Wang, P. Yin, J. Liu, M.-X. Chen, Q.-Q. Yan, Z.-S. Wang, S.-L. Xu, S.-Q. Chu, C. Cui, H. Ju, J. Zhu, Y. Lin, J. Shui and H.-W. Liang, Sulfur-anchoring synthesis of platinum intermetallic nanoparticle catalysts for fuel cells, Science, 2021, 374, 459–464 CrossRef CAS PubMed.
- L.-P. Yuan, T. Tang, J.-S. Hu and L.-J. Wan, Confinement strategies for precise synthesis of efficient electrocatalysts from the macroscopic to the atomic level, Acc. Mater. Res., 2021, 2, 907–919 CrossRef CAS.
- I. C. Gerber and P. Serp, A theory/experience description of support effects in carbon-supported catalysts, Chem. Rev., 2020, 120, 1250–1349 CrossRef CAS PubMed.
- D. Guo, R. Shibuya, C. Akiba, S. Saji, T. Kondo and J. Nakamura, Active sites of nitrogen-doped carbon materials for oxygen reduction reaction clarified using model catalysts, Science, 2016, 351, 361–365 CrossRef CAS PubMed.
- S. Chen, T. Luo, X. Li, K. Chen, J. Fu, K. Liu, C. Cai, Q. Wang, H. Li, Y. Chen, C. Ma, L. Zhu, Y.-R. Lu, T.-S. Chan, M. Zhu, E. Cortés and M. Liu, Identification of the highly active Co–N4 coordination motif for selective oxygen reduction to hydrogen peroxide, J. Am. Chem. Soc., 2022, 144, 14505–14516 CrossRef CAS PubMed.
- G. Li, Y. Li, H. Liu, Y. Guo, Y. Li and D. Zhu, Architecture of graphdiyne nanoscale films, Chem. Commun., 2010, 46, 3256–3258 RSC.
- L. Hui, Y. Xue, H. Yu, Y. Liu, Y. Fang, C. Xing, B. Huang and Y. Li, Highly efficient and selective generation of ammonia and hydrogen on a graphdiyne-based catalyst, J. Am. Chem. Soc., 2019, 141, 10677–10683 CrossRef CAS PubMed.
- W. Rong, H. Zou, W. Zang, S. Xi, S. Wei, B. Long, J. Hu, Y. Ji and L. Duan, Size-dependent activity and selectivity of atomic-level Cu nanoclusters during CO/CO2 electroreduction, Angew. Chem., Int. Ed., 2020, 60, 466–472 CrossRef PubMed.
- H. Zou, W. Rong, S. Wei, Y. Ji and L. Duan, Regulating kinetics and thermodynamics of electrochemical nitrogen reduction with metal single-atom catalysts in a pressurized electrolyser, Proc. Natl. Acad. Sci. U. S. A., 2020, 117, 29462–29468 CrossRef CAS PubMed.
- Y. Xue, B. Huang, Y. Yi, Y. Guo, Z. Zuo, Y. Li, Z. Jia, H. Liu and Y. Li, Anchoring zero valence single atoms of nickel and iron on graphdiyne for hydrogen evolution, Nat. Commun., 2018, 9, 1460 CrossRef PubMed.
- G. Shi, Y. Xie, L. Du, X. Fu, X. Chen, W. Xie, T.-B. Lu, M. Yuan and M. Wang, Constructing Cu–C bonds in a graphdiyne-regulated Cu single-atom electrocatalyst for CO2 reduction to CH4, Angew. Chem., Int. Ed., 2022, 61, e202203569 CAS.
- X.-P. Yin, S.-F. Tang, C. Zhang, H.-J. Wang, R. Si, X.-L. Lu and T.-B. Lu, Graphdiyne-based Pd single-atom catalyst for semihydrogenation of alkynes to alkenes with high selectivity and conversion under mild conditions, J. Mater. Chem. A, 2020, 8, 20925–20930 RSC.
- H. Yu, Y. Xue, B. Huang, L. Hui, C. Zhang, Y. Fang, Y. Liu, Y. Zhao, Y. Li, H. Liu and Y. Li, Ultrathin nanosheet of graphdiyne-supported palladium atom catalyst for efficient hydrogen production, iScience, 2019, 11, 31–41 CrossRef CAS PubMed.
- X. P. Yin, H. J. Wang, S. F. Tang, X. L. Lu, M. Shu, R. Si and T. B. Lu, Engineering the coordination environment of single-atom platinum anchored on graphdiyne for optimizing electrocatalytic hydrogen evolution, Angew. Chem., Int. Ed., 2018, 57, 9382–9386 CrossRef CAS PubMed.
- H. Yu, L. Hui, Y. Xue, Y. Liu, Y. Fang, C. Xing, C. Zhang, D. Zhang, X. Chen, Y. Du, Z. Wang, Y. Gao, B. Huang and Y. Li, 2D graphdiyne loading ruthenium atoms for high efficiency water splitting, Nano Energy, 2020, 72, 104667 CrossRef CAS.
- Y. Yao, Y. Zhu, C. Pan, C. Wang, S. Hu, W. Xiao, X. Chi, Y. Fang, J. Yang, H. Deng, S. Xiao, J. Li, Z. Luo and Y. Guo, Interfacial sp C–O–Mo hybridization originated high-current density hydrogen evolution, J. Am. Chem. Soc., 2021, 143, 8720–8730 CrossRef CAS PubMed.
- C. Pan, C. Wang, X. Zhao, P. Xu, F. Mao, J. Yang, Y. Zhu, R. Yu, S. Xiao, Y. Fang, H. Deng, Z. Luo, J. Wu, J. Li, S. Liu, S. Xiao, L. Zhang and Y. Guo, Neighboring sp-hybridized carbon participated molecular oxygen activation on the interface of sub-nanocluster CuO/graphdiyne, J. Am. Chem. Soc., 2022, 144, 4942–4951 CrossRef CAS PubMed.
- Z. Wang, Z. Zheng, Y. Xue, F. He and Y. Li, Acidic water oxidation on quantum dots of IrOx/graphdiyne, Adv. Energy Mater., 2021, 11, 2101138 CrossRef CAS.
- N. Wang, X. Li, Z. Tu, F. Zhao, J. He, Z. Guan, C. Huang, Y. Yi and Y. Li, Synthesis and electronic structure of boron-graphdiyne with an sp-hybridized carbon skeleton and its application in sodium storage, Angew. Chem., Int. Ed., 2018, 57, 3968–3973 CrossRef CAS PubMed.
- L. Gao, X. Ge, Z. Zuo, F. Wang, X. Liu, M. Lv, S. Shi, L. Xu, T. Liu, Q. Zhou, X. Ye and S. Xiao, High quality pyrazinoquinoxaline-based graphdiyne for efficient gradient storage of lithium ions, Nano Lett., 2020, 20, 7333–7341 CrossRef CAS PubMed.
- J. He, N. Wang, Z. Yang, X. Shen, K. Wang, C. Huang, Y. Yi, Z. Tu and Y. Li, Fluoride graphdiyne as a free-standing electrode displaying ultra-stable and extraordinary high Li storage performance, Energy Environ. Sci., 2018, 11, 2893–2903 RSC.
- Q. Pan, S. Chen, C. Wu, Z. Zhang, Z. Li and Y. Zhao, Sulfur-rich graphdiyne-containing electrochemical active tetrathiafulvalene for highly efficient lithium storage application, ACS Appl. Mater. Interfaces, 2019, 11, 46070–46076 CrossRef CAS PubMed.
- N. Wang, J. He, Z. Tu, Z. Yang, F. Zhao, X. Li, C. Huang, K. Wang, T. Jiu, Y. Yi and Y. Li, Synthesis of chlorine-substituted graphdiyne and applications for lithium-ion storage, Angew. Chem., 2017, 129, 10740–10745 CrossRef PubMed.
- Z. Zhang, C. Wu, Q. Pan, F. Shao, Q. Sun, S. Chen, Z. Li and Y. Zhao, Interfacial synthesis of crystalline two-dimensional cyano-graphdiyne, Chem. Commun., 2020, 56, 3210–3213 RSC.
- N. Elgrishi, M. B. Chambers, X. Wang and M. Fontecave, Molecular polypyridine-based metal complexes as catalysts for the reduction of CO2, Chem. Soc. Rev., 2017, 46, 761–796 RSC.
- R. Matsuoka, R. Toyoda, R. Shiotsuki, N. Fukui, K. Wada, H. Maeda, R. Sakamoto, S. Sasaki, H. Masunaga, K. Nagashio and H. Nishihara, Expansion of the graphdiyne family: A triphenylene-cored analogue, ACS Appl. Mater. Interfaces, 2019, 11, 2730–2733 CrossRef CAS PubMed.
- W. Zhou, H. Shen, C. Wu, Z. Tu, F. He, Y. Gu, Y. Xue, Y. Zhao, Y. Yi, Y. Li and Y. Li, Direct synthesis of crystalline graphdiyne analogue based on supramolecular interactions, J. Am. Chem. Soc., 2019, 141, 48–52 CrossRef CAS PubMed.
- D.-L. Meng, M.-D. Zhang, D.-H. Si, M.-J. Mao, Y. Hou, Y.-B. Huang and R. Cao, Highly selective tandem electroreduction of CO2 to ethylene over atomically isolated nickel–nitrogen site/copper nanoparticle catalysts, Angew. Chem., Int. Ed., 2021, 60, 25485–25492 CrossRef CAS PubMed.
- I. Platzman, R. Brener, H. Haick and R. Tannenbaum, Oxidation of polycrystalline copper thin films at ambient conditions, J. Phys. Chem. C, 2008, 112, 1101–1108 CrossRef CAS.
- S. Nitopi, E. Bertheussen, S. B. Scott, X. Liu, A. K. Engstfeld, S. Horch, B. Seger, I. E. L. Stephens, K. Chan, C. Hahn, J. K. Norskov, T. F. Jaramillo and I. Chorkendorff, Progress and perspectives of electrochemical CO2 reduction on copper in aqueous electrolyte, Chem. Rev., 2019, 119, 7610–7672 CrossRef CAS PubMed.
- Q. Hu, Z. Han, X. Wang, G. Li, Z. Wang, X. Huang, H. Yang, X. Ren, Q. Zhang, J. Liu and C. He, Facile synthesis of sub-nanometric copper clusters by double confinement enables selective reduction of carbon dioxide to methane, Angew. Chem., Int. Ed., 2020, 59, 19054–19059 CrossRef CAS PubMed.
- R. Reske, H. Mistry, F. Behafarid, B. Roldan Cuenya and P. Strasser, Particle size effects in the catalytic electroreduction of CO2 on Cu nanoparticles, J. Am. Chem. Soc., 2014, 136, 6978–6986 CrossRef CAS PubMed.
- L. Li, A. H. Larsen, N. A. Romero, V. A. Morozov, C. Glinsvad, F. Abild-Pedersen, J. Greeley, K. W. Jacobsen and J. K. Nørskov, Investigation of catalytic finite-size-effects of platinum metal clusters, J. Phys. Chem. Lett., 2013, 4, 222–226 CrossRef CAS PubMed.
- A. Wuttig, C. Liu, Q. Peng, M. Yaguchi, C. H. Hendon, K. Motobayashi, S. Ye, M. Osawa and Y. Surendranath, Tracking a common surface-bound intermediate during CO2-to-fuels catalysis, ACS Cent. Sci., 2016, 2, 522–528 CrossRef CAS PubMed.
- Y. Huang, A. D. Handoko, P. Hirunsit and B. S. Yeo, Electrochemical reduction of CO2 using copper single-crystal surfaces: Effects of CO* coverage on the selective formation of ethylene, ACS Catal., 2017, 7, 1749–1756 CrossRef CAS.
- X. Liu, P. Schlexer, J. Xiao, Y. Ji, L. Wang, R. B. Sandberg, M. Tang, K. S. Brown, H. Peng, S. Ringe, C. Hahn, T. F. Jaramillo, J. K. Nørskov and K. Chan, PH effects on the electrochemical reduction of CO2 towards C2 products on stepped copper, Nat. Commun., 2019, 10, 32 CrossRef CAS PubMed.
- Y. Zhou, F. Che, M. Liu, C. Zou, Z. Liang, P. De Luna, H. Yuan, J. Li, Z. Wang, H. Xie, H. Li, P. Chen, E. Bladt, R. Quintero-Bermudez, T.-K. Sham, S. Bals, J. Hofkens, D. Sinton, G. Chen and E. H. Sargent, Dopant-induced electron localization drives CO2 reduction to C2 hydrocarbons, Nat. Chem., 2018, 10, 974–980 CrossRef CAS PubMed.
- P.-P. Yang, X.-L. Zhang, F.-Y. Gao, Y.-R. Zheng, Z.-Z. Niu, X. Yu, R. Liu, Z.-Z. Wu, S. Qin, L.-P. Chi, Y. Duan, T. Ma, X.-S. Zheng, J.-F. Zhu, H.-J. Wang, M.-R. Gao and S.-H. Yu, Protecting copper oxidation state via intermediate confinement for selective CO2 electroreduction to C2+ fuels, J. Am. Chem. Soc., 2020, 142, 6400–6408 CrossRef CAS PubMed.
- W. Ma, X. He, W. Wang, S. Xie, Q. Zhang and Y. Wang, Electrocatalytic reduction of CO2 and CO to multi-carbon compounds over Cu-based catalysts, Chem. Soc. Rev., 2021, 50, 12897–12914 RSC.
|
This journal is © the Partner Organisations 2023 |
Click here to see how this site uses Cookies. View our privacy policy here.