DOI:
10.1039/D2QI02027K
(Research Article)
Inorg. Chem. Front., 2023,
10, 127-136
Pushing capacities and energy densities beyond theoretical limits of lithium primary batteries using active CFx nanocapsules with x > 1†
Received
20th September 2022
, Accepted 8th November 2022
First published on 10th November 2022
Abstract
The high theoretical capacity and long shelf-life of a Li–CFx system make it most promising for portable electronics. However, the inactivation ideology of –CF3 groups in CFx hinders the development of the Li–CFx system to realize ultra-high energy density. Here, we developed a unique carbon fluoride nanocapsule (CFNC) with x > 1 using a simple thermal-assisted chemical reaction in a controlled environment. The high curvature 3D hollow structure and rich interfaces generated by the engineered wall structure of the CFNC enable activation of –CF and –CF2 groups. The resulting structure bears high mass and rich charge transfer channels which deliver a cathode capacity of 1056 mA h g−1 and energy density up to 2487 W h kg−1 beyond the theoretical limits of the Li–CFx system without compromising the cell voltage. To understand the role of structural engineering, density functional theory (DFT) calculations were carried out to confirm active CF2 components and the effects of various fragment sizes on the voltage plateau, where a higher discharge plateau voltage is obtained with the larger fragment. The materials design presented in this study is an effective and alternative approach to realizing primary batteries with ultra-high energy densities.
Introduction
A lithium primary battery is an indispensable energy storage device and is widely used in a diverse range of portable electronic tools from civil to space applications.1–4 For sustainable advancement of these technologies, power sources, with high energy densities, safety and prolonged activity are needed.5–8 Among different systems, batteries using carbon fluoride (CFx) based cathode materials delivered high energy densities due to the high theoretical capacity of 865 mA h g−1 (when x = 1) of these materials compared to Li/SOCl2 and Li/MnO2 batteries.9–11 However, the complex chemistry and inactivation of CFx in certain molecular forms limit its full exploration; therefore, an in-depth understanding of the CFx mechanism of action and corresponding chemical modifications is required to make CFx the only choice for lithium primary batteries.12–15 In this aspect, a series of fluorinated carbonaceous materials are being investigated in different geometries, such as fluorinated nanotubes,16,17 fluorinated carbon nano-discs (CND),18 fluorographene,19,20 and fluorinated graphite.21,22 Among these, the fluorographene and fluorinated CND displayed excellent performance by delivering a capacity of 1180 mA h g−1 and an energy density of 2338 W h kg−1 at 10 mA g−1, because the reinforcement effect of the central discs and the presence of the LiF shell allowed the diffusion of lithium ions through the sheet edges or from the surface cracks.18 The fluorinated carbon capsule was decorated with conductive carbon layers by employing a combined ALD-CCVD technique to improve the kinetics of electrode reaction and decrease polarization, which is beneficial to upgrade the energy density and rate capacity.23 Recent discoveries also found that introducing a high concentration of semi-ionic bonds improves the energy density of CFx batteries.24 However, the working conversion reaction of CFx as well as the generation and chemical stability of semi-ionic bonds are not well-understood to harvest the full potential of these materials in realizing sustainable energy densities for longer operations.25,26 To achieve high-performance CFx materials certain materials characteristics need to be tuned in such a way that the resulting materials exhibit higher conductivity, larger semi-ionic bonds, and easy diffusion of lithium ions.27,28 It has been observed that semi-ionic bonds play a crucial role in pushing the performance by providing better conductivity, discharge voltage and rate capability,7,19,22,29 while the covalent bonds are chemically more stable with higher bond energies which reduced the performance.30 Therefore, it is highly important that the CFx materials should contain more semi-ionic bonds than covalent ones to ensure high performance. In this regard, Zhang et al. found an interesting phenomenon that curved structures help in achieving a higher ratio of semi-ionic bonds compared to flatter ones through preparing fullerenes C60 with single, double and multi-walled carbon nanotubes.31 Hence, realizing curved surfaces within a carbon framework will help in achieving a great shift from covalent to semi-ionic bonds in CFx. However, conventionally, carbon frameworks have large flexibility; therefore, bringing saturated curved surfaces is very challenging.32 Here, we have developed a unique 3D carbon fluoride nano-capsule (CFNC), which possesses a hollow structure with a large cavity having one end open like a capsule. More interestingly, the curved walls are further tuned by creating additional voids and flexibility which positively contribute to realizing a large number of ionic and semi-ionic bonds. Furthermore, high inter-layer space allows faster mass transport and ionic diffusion and a large cavity acts as a reservoir for the byproduct (LiF) and hence does not interfere with the ongoing discharge process. Consequently, semi-ionic and ionic bond rich CFNCs displayed an exceptionally high discharge capacity of 1056 mA h g−1 and an energy density of 2487 W h kg−1, superior to those of the previously reported fluorinated carbon materials and beyond theoretical limits. To understand the role of the structure in delivering such high performance, DFT calculations were also conducted which explain the reaction path and kinetic process among Li+ and C–F bonds. As this method can produce hectograms of CFNCs with exceptional performance it makes it suitable for commercial-scale applications.
Experimental
Sample preparation
Synthesis of carbon nanocapsules (CNCs).
CNCs were developed on the surface of zinc oxide (ZnO) nanoparticles by the catalytic chemical vapor deposition (CCVD) technique. The typical process is based on the following steps: first, 20 g of ZnO nanoparticles (used as a template) were placed in the middle of a rotating furnace and heated up to an optimum reaction temperature of 600 °C–650 °C with a heating rate of 10 °C min−1 under N2 (a carrier gas). Subsequently, acetylene (C2H2) gas was introduced into the furnace with a flow rate of 30 mL min−1 for 30 min at optimum temperature. Later, the furnace was cooled down to room temperature to obtain ZnO@CNCs. Second, etching of the ZnO template was carried out using HNO3 solution. After that, GCs were washed with distilled water and ethanol alternately six times to remove the impurities and dried at 60 °C for 24 h. Finally, to remove some residual impurities and achieve partial graphitization of CNC walls, the final CNC product was annealed at 900 °C for 4 h.
Synthesis of carbon fluoride nano-capsules (CFNCs).
Typically, CNCs were put into a horizontal furnace and heated up to the reaction temperature of 325–380 °C with a heating rate of 10 °C min−1 under a N2 atmosphere. Then, a mixture of 85% N2 and 15% fluorine (F2) gas was introduced into the furnace with a total flow rate of 160 mL min−1 for 120 min to furnish the fluorination process. Finally, the furnace was allowed to cool down naturally to room temperature under N2 and the obtained samples were grey to white in color and labeled as CFNC-325, CFNC-340, CFNC-350, CFNC-360, CFNC-370, and CFNC-380, respectively.
Performance measurements
Electrochemical measurements were carried out with conventional 2032-coin cells. The cathode was prepared on an aluminium foil by coating with a slurry of active materials (as-obtained CFNCs), conductive agent (Ketjen black) and polymer binder (polyvinylidene fluoride, PVDF) dissolved in a solvent (N-methylpyrrolidone, NMP) with a mass ratio of 8
:
1
:
1, respectively. The coating was dried in a vacuum oven at 80 °C for 12 h. Then, the coated foil was cut into disks of 14 mm diameter to fabricate the cathode discs and pressed under 12 MPa for 30 s. Finally, the obtained electrodes were weighed and transferred into an argon-filled glovebox to assemble the coin cells. The mass loading of activating materials is about 0.4 mg cm−2 and the thickness of the electrode is about 5 μm. Typically, coin cells were assembled with lithium foil as the anode and a Celgard 2400 membrane as a separator. The coin cells were discharged on a NEWARE battery testing system at a constant current from the initial voltage to 1.5 V at a voltage change rate of 0.01C–0.1C. All the above processes were carried out at room temperature.
Characterization
The morphological and structural features of pure CNCs and CFNCs were characterized by field emission scanning electron microscopy (JSM-7600F; JEOL, Ltd, Tokyo, Japan) and transmission electron microscopy (JEM-2100F; JEOL, Ltd, Tokyo, Japan) using an operating voltage of 200 kV. The X-ray diffraction patterns were recorded in the 2θ range of 10–60° using an X-ray diffractometer (XRD-7000; Shimadzu, equipped with Cu Kα, λ = 0.154178 nm). The elemental composition of the FGCs was analyzed by X-ray photoelectron spectroscopy (XPS, Escalab 250Xi), using a Kartos Axis Ultra with monochromatized Al Kα radiation (1486.6 eV). The thermogravimetric analysis (TGA, STA8000) was performed with an STA8000 under a N2 atmosphere at a heating rate of 5 °C min−1 from 50 to 800 °C. Raman spectra were recorded using a Renishaw 1000 Raman imaging microscope system with an excitation wavelength of 785 nm.
Statistical analysis
All the calculations were performed using Amsterdam Modeling Suite (AMS) software within density functional theory (DFT), with which the Li–CFx or F–C interactions are described. The generalized gradient approximation (GGA) with the PBE/BP86/B3LYP exchange–correlation functional and TZP basis sets was used for all structural optimizations and binding energy calculations.
Results and discussion
Structure and electrochemical performance
To obtain uniformly structured CFNCs, carbon NCs (CNCs) were used as the sacrificial material, schematically shown in Fig. S1a.† A serialized device is developed to realize CNCs of hectogram-scale (Fig. S1b†). Initially, pure CNCs with uniform wall thickness were synthesized using our previous methods, and these CNCs bear a distorted wall structure due to the unique arrangement of graphitic carbon fragments.33 This unique wall structure and overall cup-like morphology make them highly suitable for fluorination reaction and modulating the C–F bond type.34 Afterwards, the CNCs were fluorinated in the presence of F2 gas at 325–380 °C to develop various compositions of CFNCs. It is hypothesized that the strain induced by the curved structure decreases the overlap between carbon and fluorine orbitals, weakening the C–F2 bonding to activate C–F2 bonds, hence contributing positively towards discharge capacity (Fig. 1a). Furthermore, specific temperature conditions were optimized to accelerate the generation of F radicals and their diffusion for efficient solid–gas reaction. The successful conversion of CNCs to CFNCs can also be observed from the obvious color change from black to white after the fluorination reaction (Fig. 1b and c). Because the CFNCs have the advantage of being fluffy, the measured tap density of CFNCs is 0.15 g cm−3. The scanning electron microscopy (SEM) images of CNCs and CFNCs exhibited uniformly distributed hollow capsules (Fig. S2†); however, widening of the opening of capsules was observed. This effect is more pronounced in the samples prepared at 360 °C (CFNC-360), where almost all the capsules have a clear opening. The widening/appearing of a clear opening might occur due to a vigorous fluorination reaction at opening edges due to the presence of dangling bonds. The magnified transmission electron microscopy (TEM) image of CNCs delineated a capsule-like shape that possesses a uniform wall thickness of 5.9 nm (Fig. S3a and b†). The microstructural analysis through HRTEM and fast Fourier transform (FFT) illustrated the partial graphitic nature of CNCs in which the walls are defect rich and consist of graphene sheets (Fig. S3b†). Regarding CFNC-360, it retained the original open-ended capsule-like shape with an increased wall thickness of 13.6 nm twice that of CNCs with an amorphous structure (Fig. 1d and e) and exhibited exfoliated sheets on the surface of the capsule (Fig. S3c and d†). This might be due to the fluorination reaction which partially excavated the carbon framework and increased the interlayer space, hence making ionic diffusion much faster than that of the traditional carbon structure. Moreover, Fig. S4† shows elemental distribution which confirms that F and C elements are uniformly distributed on the CFNCs without any impurity. To further verify the purity of the as-synthesized CFNCs, an inductively coupled plasma-atom emission spectrometer (ICP-AES) was used which confirms the absence of transition metals (Ni, Co, Fe and Zn) (Table S1†). The X-ray diffraction (XRD) spectra of CFNCs show three peaks, first at 12.2° indexed to (001), indicating a high degree of fluorination. The second peak at ∼25° corresponds to (002) reflecting a 3D curved structure with poor regularity along the stacking direction,20 while the third peak at ∼41° is associated with (100) and attributed to the C–C in-plane length in the capsule reticular system.22 Furthermore, to evaluate the graphitic contents, the Raman spectra were recorded which show two distinct peaks located at ∼1313 and ∼1592 cm−1 corresponding to the D and G bands, respectively, for CNCs (Fig. 1f).24,35 The ID/IG ratio of 1.61 indicates partial-graphitization of CNCs with a large number of defects, while fluorination broke the sp2-hybridized bonds into the sp3-hybridized system to generate a C–F structure, confirmed by an enhanced D-band with a slight shift at ∼1375 cm−1 and a disappeared G-band in the Raman spectrum of CFNCs (Fig. 1g). In fact, the energetic F ions brought the interconnections among the overlapped layers to produce disordered sp3 structures, hence confirming a complete structural change.36 The FT-IR spectra were obtained to further reveal the chemical structures of CFNCs (Fig. 1h). CFNCs exhibited a strong peak at ∼1227 cm−1 and a shoulder peak at ∼1301 cm−1 ascribed to the stretching vibrations of covalent C–F and C–F2 bonds, respectively.37 Likewise, a shoulder at ∼1022 cm−1 is observed in CFNCs, corresponding to the stretching vibrations of semi-ionic C–F bonds.38 The absorption peak of CFNCs-325 at ∼1643 cm−1 is indexed to the stretching vibration of in-plane C
C bonds, while a much weaker absorption peak of CFNC-360 at ∼1650 cm−1 is observed.24
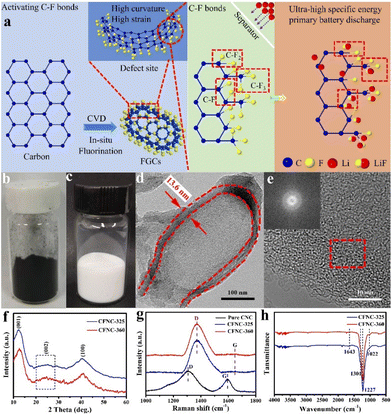 |
| Fig. 1 The formation mechanism, morphologies and characterization of CFNCs. (a) The schematic presentation of CFNC formation with a high curvature by strain force and the diagrammatic representation of the discharging mechanism involved in different types of C–F bonds. (b) The photograph of the as-synthesized CNCs. (c) The photograph of as-synthesized CFNC-360; (d) TEM CFNC-360 with a wall thickness of 13.6 nm, (e) HRTEM image of CFNC-360 (the inset shows an FFT image). (f) XRD spectra of CFNC-325 and CFNC-360. (g) Raman spectra of CNCs, CFNC-325 and CFNC-360. (h) FT-IR spectra of CFNC-325 and CFNC-360. | |
To reveal the thermal stability of CFNCs and the F/C ratio, a thermogravimetric (TG) analysis was performed which shows three-stage weight loss (Fig. 2a). The first weight loss is due to surface adsorbed water or loosely attached functional groups or/and unreacted fluorine, where CFNC-360 showed the highest thermal stability from 30 to 400 °C. The second significant weight loss is due to the separation of fluorine atoms from the capsule framework from 400 to 610 °C. The final weight loss is observed from 610 to 800 °C due to the decomposition of the carbon skeleton, where CFNC-360 exhibited less residual weight implying that a higher degree of fluorination made the carbon skeleton weaker. The residual weight of CFNC-325, CFNC-340, CFNC-350, CFNC-360, CFNC-370 and CFNC-380 is 39.36%, 35.14%, 29.77%, 28.12%, 29.49% and 31.51%, respectively. The F/C ratio of each sample was estimated by the weight change in the second stage using eqn (1). The maximum F/C ratio of 1.61 was obtained while the reaction temperature was optimized at 360 °C. When the fluorination temperature is high, significant pyrolysis of already grafted fluorine-containing groups takes place and the high temperature supports the rearrangement reactions passed onto the surface of the carbon matrix, which causes partial degradation of the surface layer.39 The pyrolysis and rearrangement of the surface of the carbon matrix reduce the relative fluorine content and F/C ratio, which leads to a volcanic-type F/C ratio. The F/C ratios of all samples are shown in Fig. 2b.
| 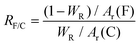 | (1) |
where is the F/C ratio,
WR is the residual weight at the end of the second stage, and
Ar(F) and
Ar(C) are relative atomic masses of F and C, respectively.
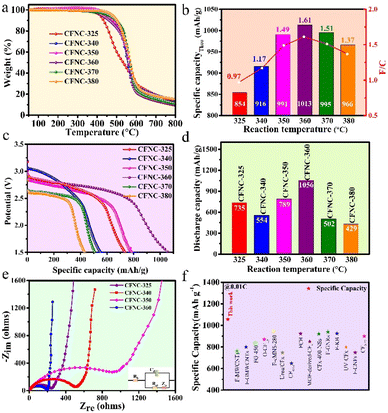 |
| Fig. 2 The thermal stability and performance of CFNCs as a cathode in a lithium primary battery system. (a) TG curves for CFNCs synthesized at different reaction temperatures. (b) The dependence of reaction temperature on the F/C ratio achieved by the TG test and the corresponding theoretical specific capacity histogram. (c) The galvanostatic discharge curves of CFNCs synthesized at different temperatures at 0.01C. (d) The delivering specific capacity of CFNCs synthesized at different temperatures for a Li/CFx battery. (e) Electrochemical impedance spectroscopy (EIS) curves for CFNCs synthesized at a temperature range from 325 °C to 360 °C. (f) Comparison of the specific capacity for CFNC-360 with various art exemplar fluorinated carbon active materials (Table S4†). | |
Taking advantage of the unique structure and composition of CFNCs, they were tested as cathode materials for a lithium primary battery. It is worth mentioning that the F/C ratio of 1.61 can deliver a theoretical capacity of 1013 mA h g−1 (Fig. 2b and Table S2†), showing that attaining a functional higher ratio of F can extend the energy density of primary batteries. The galvanostatic discharge curves of the as-synthesized CFNCs at different reaction temperatures show their typical performance (Fig. 2c). Interestingly, different samples deliver different capacities, for example CFNC-325, CFNC-340, CFNC-350 and CFNC-360 delivered 735, 554, 789 and 1056 mA h g−1, respectively, at a discharge rate of 0.01C, confirming that the ratio of F/C and chemical structure played a key role (Fig. 2d). The excellent performance of CFNC-360 is because of the high F/C ratio (1.61) that provides more active sites for binding Li+ ions and strain-induced conjugate reconstruction activated C–F bonds. When the CFNCs possessed the C–C honeycomb layer, the isolated C–F bonds were adjacent to the conjugated structure. The conjugated structure will significantly reduce the stability of the C–F and C–F2 bonds.37 Moreover, the open-ended capsules provided wide channels to facilitate the mass transport and diffusion of Li+ ions for enhanced electrochemical performance.40 To observe the impact of chemical stability, samples prepared at higher temperatures were also tested which showed 502 mA h g−1 for CFNC-370 and 429 mA h g−1 for CFNC-380 clearly showing that both the F/C ratio and structural stability of cathode materials are important to achieve maximum performance. Moreover, CFNC-360 delivers the highest energy density of 2487 W h kg−1 (Fig. S5†), attributed to the reasonable number of hybridized SP2 C
C and SP3 C–C bonds, a suitable number of C–F bonds, and an abundance of electrochemically active semi-ionic C–F bonds. Electrochemical impedance spectroscopy (EIS) was utilized to probe the role of structure and morphology in performance as shown in Fig. 2e. It is found that the conductivity decreases as the synthesis temperature increases from 325 to 350 °C as CFNC-325 possesses a lower charge-transfer resistance (Rct), as a lower degree of fluorination still leaves partially hybridized carbon (Table S3†). However, the best Rct value is achieved for CFNC-360 where the optimized structure helps in maintaining good conductivity.41 A mixed trend is observed for ionic diffusion as the Warburg impedance (Zw) decreases as we move from 325 to 340 °C and then slightly increases at 350 °C and again decreases for the sample prepared at 360 °C, following the same fashion as observed for the discharge capacity for these samples. This shows that CFNC-360 has optimized electroconductivity and diffusivity of Li+ to deliver maximum specific capacity, which results in the best energy density value of 2487 W h kg−1 compared to other samples prepared at different temperatures. Most importantly, when the discharge capacity is compared with the recently published literature, CFNC-360 stands best as a cathode of a primary Li/CFx battery (Fig. 2f and Table S4†). A 3-day period OCV stability test to confirm the as-synthesized CFNC cathode materials and the as-designed Li/CFx primary battery has small self-discharge behavior. The OCV stability test confirms that the as-synthesized CFNC cathode materials and the as-designed Li/CFx primary battery show negligible self-discharge behavior as shown in Fig. S6.†
The nature of the C–F bond plays a significant role in delivering high capacity. The high capacity is ascribed to the highest semi-ionic C–F bonds and the partial extra-activated C–F2 bonds provide more F atoms that could participate in the discharge reaction. Therefore, X-ray photoelectron spectroscopy (XPS) was used to probe different bonding situations in CFNCs synthesized at different temperatures (Fig. S7†) and the corresponding ratios of different bonds are provided in Table S5.† The deconvoluted C 1s spectrum of CFNC-325 showed peaks at 284.7 and 286.2 eV indexed to sp3 (C–C, 21%) and sp2 (C
C, 14.3%) bonds,42 respectively, indicating the presence of a graphitic structure similar to that of pure GCs (Fig. 3a). An additional four peaks appeared at 288.9 eV, 289.8 eV, 290.5 eV and 291.7 eV ascribed to semi-ionic C–F (11.2%) and covalent C–F (34.1%), C–F2 (9.9%) and C–F3 (9.5%) bonds, respectively.43 Particularly, the CF2 and CF3 groups are produced at the defects and edges of CFNCs. Hence, high graphitic carbon content resulted in good conductivity and low polarization but poor performance due to the low ratio of activated semi-ionic C–F, which are consistent with the EIS finding. The C 1s signal of CFNC-340 consists of six peaks centered at 284.2 eV (C–C, 14.6%), 285.3 eV (C
C, 7.5%), 289.2 eV (semi-ionic C–F, 10.6%), 289.7 eV (covalent C–F, 44%), 291.3 eV (C–F2, 21%) and 291.7 eV (C–F3, 2.3%), showing that the graphitic content reduced but there was greater covalent fluoride bonding (Fig. S8†). The lower semi-ionic C–F and higher C–F2 bonds are detected in CFNC-340. Although the F content of CFNC-340 is high, the lower semi-ionic C–F content means that fewer F atoms could participate in the discharge reaction, resulting in an insufficient discharge reaction and low discharge capacity. On further increase of temperature to 350 °C, the graphitic contents diminished and more fluoride bonds formed as the C 1s spectrum deconvoluted to four peaks at 289.8 eV (semi-ionic C–F, 34.4%), 290.1 eV (covalent C–F, 34.5%), 290.6 eV (C–F2, 17.3%) and 291.9 eV (C–F3, 13.8%), (Fig. S7b†). Although the proportion of semi-ionic C–F bonds increases, theoretically delivering the largest specific energy, the low electroconductibility and maximum content of inactivated C–F3 bonds limit their performance. As the reaction temperature reaches 360 °C, the C 1s signal presents C–C (7.3%), semi-ionic C–F (33.6%), covalent C–F (37.2%), C–F2 (15.1%) and C–F3 (6.8%) bonds at 284.6 eV, 289.7 eV, 290.3 eV, 291.6 eV, and 292.1 eV, respectively (Fig. 3b). The C–C bond was again detected on the surface of CFNCs-360 which increased its conductivity and the presence of the highest proportion of activated C–F bonds (70.8%) and low semi-activated C–F2 results in the largest specific energy. With a further increase in reaction temperature to 370 °C, the F content of CFNC-370 deteriorates. The C 1s spectrum showed five peaks fitted at 284.3, 285.3, 289.4, 290.0 and 291.2 eV, corresponding to the C–C (9.4%), C
C (6.1%), semi-ionic C–F (29.9%), covalent C–F (25.5%) and C–F2 (29.2%) bonds, respectively (Fig. S7c†). On increasing the synthesis temperature to 380 °C, the inactivated C–F3 bond gradually turned into semi-activated C–F2 and activated C–F bonds along with the appearance of a graphitic component. The reduced semi-ionic C–F bonds and most semi-activated C–F2 bonds convert to large amounts of C–C bonds and covalent C–F bonds. This conclusion is drawn based on the fitted peak positions at 284.2, 285.5, 288.1, 289.5 and 291.3 eV corresponding to C–C (41.7%), C
C (7.2%), semi-ionic C–F (3.4%), covalent C–F (36.4%) and C–F2 (11.3%) bonds, respectively (Fig. 3c).
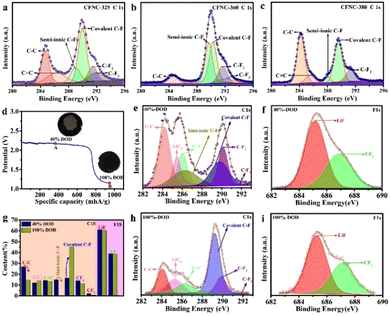 |
| Fig. 3 High-resolution XPS spectra of C 1s for the as-synthesized CFNCs prepared at different reaction temperatures: (a) 325 °C; (b) 360 °C; and (c) 380 °C. (d) Galvanostatic discharge curve at a discharge rate of 0.01C for CFNC-360 with two different DOD (as-prepared cell without an SP conductive agent); (e) high-resolution XPS spectra of C 1s for cathode materials after 40%DOD; (f) high-resolution XPS spectra of F 1s for cathode materials after 40%DOD; (g) the content change of different bonding types and relative proportions of the bonds after different DOD; (h) high-resolution XPS spectra of C 1s for cathode materials after 100%DOD; and (i) high-resolution XPS spectra of F 1s for cathode materials after 100%DOD. | |
In a nutshell, the proportion of activated C–F bonds (semi-ionic and covalent C–F) varies between 45% and 70% (first increase with the synthesis temperature up to 360 °C and then decrease), which plays a dominant role in improving the performance of CFNCs-360. The F/C ratio was also determined by XPS which falls between 0.92 and 1.47 at different synthesis temperatures as shown in Table S6.† It is found that at lower temperatures, the value of the F/C ratio is consistent with the TG results, but at a higher temperature it deviates from the TG findings which might be due to the decomposition of C–F bonds under X-ray irradiation. To probe the contribution of CF2 groups towards high energy delivered by CFNCs, depth-of-discharge (DOD) test and ex situ XPS studies were carried out. Here, the as-prepared cell only contains active materials (CFx) and adhesives (PVDF) to eliminate the influence of conductive carbon black on the XPS carbon peak. A cathode collector extracted from a cell at 40% and 100% discharge, with visuals presented in the inset of Fig. 3d, clearly shows an unreacted region in the center for 40% and complete black carbon over 100% discharge. The XPS spectra in Fig. 3e–i reveal that with increasing discharge time, the content of semi-ionic C–F bonds rapidly degenerates from 15% to 0.01% and an increment from 16.3% to 45% in covalent C–F bonds occurred. Therefore, reduction in CF2 and CF3 groups shows that these are the active components in delivering energy density beyond theoretical limits. Moreover, the content of LiC6 has a slight increase from 12% to 14% when the discharge completes, which means that an increase in energy density occurs by forming the graphite intercalation compounds (GICs) when Li+ inserts into the graphite layers.44,45
DFT and computational details
Thermodynamic studies revealed that –CF2 and –CF3 groups are less reactive than –CF as higher energy is required to break strong bonds; however, as shown above, we found that these groups played a key role in delivering energy densities even beyond theoretical prediction, showing the active nature of these groups.34,46 Therefore, DFT calculations were performed to understand the activity and contribution of the –CF2 group. Considering the structure of CFNCs and the F/C ratio, a chair configuration is found most suitable with the lowest energy having sheets consisting of 24 carbon and 36 fluorine atoms. Therefore, three chair structure species of C24F36, C32F46 and C33F47 were built to model the CFx materials with different sizes and defects as shown in Fig. 4a, c and e, respectively. Moreover, the same three species with only one –CF2 group were also built as shown in Fig. 4b, d, and f, respectively, considering that the progressing reaction with Li will consume most of the F atoms. After obtaining a stable structure to probe the activity of –CF2 groups, the absorption energy of Li ions reacting with F atoms on six theoretical models is calculated. The performance of the high energy density primary batteries of Li/CFx depends on the value of x strongly, so the interaction energy between the Li and CFx layers is a very important parameter. In order to quantitatively describe the interactions between the adsorbate and F atoms, we adopt a common definition of the binding energies based on eqn (2):47 | ECnFmLi = E(Li) + E(CnFm) − E(CnFmLi) | (2) |
where, n and m represent the number of C and F atoms in the fluorinated graphene fragment. ECnFmLi is the binding energy of Li+ on CnFm species. E(Li) + E(CnFm) is the energy of the Li/CFx system when a Li atom is 10 Å away from CnFm, and E(CnFmLi) is the energy when the system is optimized to a stable structure.
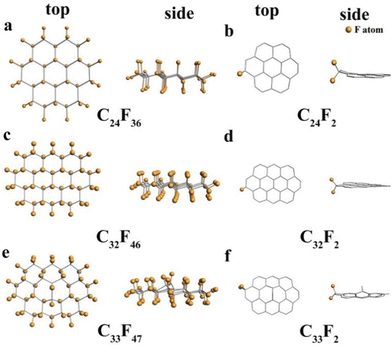 |
| Fig. 4 The structures of the fluorinated graphene fragments in top and side views. (a) C24F36; (b) C24F2; (c) C32F46; (d) C32F2; (e) C33F47; and (f) C33F2. | |
All the theoretical calculations are carried out in the solvent medium considering the presence of electrolytes in battery systems which is also proved by the outcome presented in Fig. 5a. As the calculations show that the binding energies of Li and C24F36 in the gas phase (C24F36Li-g) are very different from those in the solvent environment (C24F36Li-s) as shown in Table S7.† The calculated binding energy of 2.36 eV in the solvent is close to the experimental results as the Li-CFNC cell showed a discharge plateau of 2.5 V (Fig. 2d). Moreover, as explained in the Experimental section three different functionals were used to calculate the binding energies (Fig. 5b), which showed slight differences; therefore, PBE is followed for the rest of the calculations and considered the most reliable one. Typically, F atoms prefer to bond with the edge carbon atoms of the chair-type graphene edge plane (e-) and basal plane (b-); hence, e-C24F36Li, b-C24F36Li, e-C32F46Li, b-C32F46Li, and e-D-C33F47Li species were chosen to calculate the binding energy transformation, shown in Fig. 5c. The calculated binding energies of Li ions and LiF with different CnFm species are listed in Table 1.
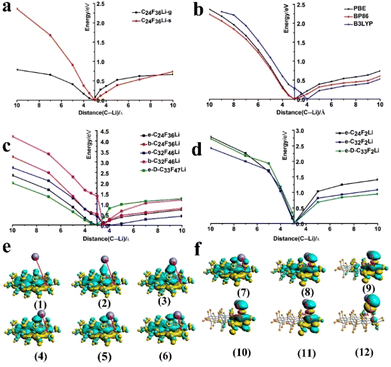 |
| Fig. 5 (a) Binding energy transformation of a Li atom attacking CF in two environments including air and solution for C24F36Li supercell structures. (b) Binding energy transformation of a C24F36 supercell structure calculated using PBE, BP86 and B3LYP functionals, respectively. Binding energy transformation of the Li atom attacking an F atom at different positions for different supercells. (c) Binding energy transformation of the Li atom attacking CF during the production of LiF. (d) Each image shows the C24F36Li system, the node of the stick represents a C atom, orange pellets represent an F atom, purple pellets represent Li+, and the red two-way snip lines indicate the distance between C and Li atoms. (e and f) The LUMO for the charge transformation in the e-C24F36Li system; blue/yellow contours correspond to accumulation/depletion of electron density. | |
Table 1 The binding energies of Li ions and LiF with different CnFm species, where E1 is the binding energy of Li+, E2 is the binding energy of LiF, and ΔE = E1 − E2 presents the energy change during the whole reaction process
Structure |
E1 [eV] |
E2 [eV] |
ΔE [eV] |
e-C24F36Li |
2.37 |
0.74 |
1.63 |
b-C24F36Li |
3.26 |
0.80 |
2.46 |
e-C32F46Li |
2.73 |
0.43 |
2.3 |
b-C32F46Li |
4.22 |
1.21 |
3.01 |
e-D-C33F47Li |
2.00 |
1.26 |
0.74 |
|
e-C24F2Li |
2.79 |
1.41 |
1.38 |
e-C32F2Li |
2.43 |
1.08 |
1.35 |
e-C33F2Li |
2.73 |
0.95 |
1.78 |
Specifically, when the Li atom is 10 Å away from the edge and basal planes, the absorbing energy is 2.37 and 3.26 eV for e-C24F36Li (black line) and b-C24F36Li (red line) systems, respectively, showing that F atoms bonded to the basal plane take more energy than the edge plane ones for the C24F36 structure. Similarly, for a larger structure (C32F46), the binding energy of Li+ is 2.73 eV and 4.22 eV for e-C32F46Li (blue line) and b-C32F46Li (pink line) systems, respectively, showing that increasing system size required more energy. However, it is found that defects help in lowering the binding energy as calculated Li+ binding energy for the fluorinated graphene system with defects (e-D-C33F47Li, green line) is only 2.00 eV, while the capturing energy for F atoms to generate LiF is 1.26 eV (highest) and 0.43 eV (lowest) for e-D-C33F47Li and e-C32F46Li, respectively. The consumption of F atoms during the discharge process reduces the degree of fluorination on carbon fragments, including –CF2 and –CF3 groups. Therefore, three different size structures (C24F2, C32F2, and C33F2) having only one –CF2 group on each were constructed to explore the activity of the –CF2 group. The resulting three energy systems referred to as e-C24F2Li, e-C32F2Li and e-D-C33F2Li showed Li+ binding energy of 2.79, 2.43 and 2.73 eV, respectively (Fig. 5d). Meanwhile, in the last stage of the reaction for each system, the difference between the values of E1 and E2 is approximately 1.5 eV. The LUMO shows the charge transformation for the e-C24F36Li system when the Li atoms are close to or far enough from the F atoms on the –CF2 group, as indicated in Fig. 5e and f. The process from (1) to (6) and (7) to (12) shows that the F atom is attacked by Li+ ions and captured by Li+ ions, respectively, corresponding to the black line on the left and right of Fig. 5c.
Besides, the binding energy transformation of the Li atom attacking the C–F2 bond is used to verify the activity of C–F2 bonds on the as-synthesized CFNCs. The weakening of the C–F bonding covalence caused by the curvature was consistent with A. Hamwi's work.48 Basically, the curvature results in strain in the carbon lattice as revealed by the pyramidalization and misalignment of the π-orbitals. During fluorination, the C–F bond formation requires the change of carbon hybridization from sp2 to sp3. The curvature prevents the formation of a pure sp3 hybridization for the carbon atom, since it requires an important local strain. The residual sp2 hybridized orbitals imply a weakening of the overlapping of the hybridized lobes of carbon and the fluorine atomic orbitals. This covalence weakening affects the C–F bond strength and leads to a lack of robustness of the C–F interaction within fluoride carbon. In the current work, we mainly emphasized that the C–F2 bonds were activated by the curvature because of the unique 3D structure of fluoride carbon capsules (CFNCs) because the presence of a large number of carbon and fluorine atoms in the CFNCs provides a huge calculation model for CFNCs. Therefore, it is difficult to truly calculate the C–F bond ionicity by constructing the CFNCs due to the capacity limit of computing. The influence of curvature on the C–F bond ionicity is principally interlinked to the tubular structure. Therefore, to verify the change of C–F ionicity caused by the curvature, we realized the model of planar graphene and single-wall carbon nanotubes using the DMol3 module to calculate the thermal stability of the C–F2 bonds with different curvatures (Fig. S9†). According to the DFT calculations, the dissociation energy of C–F2 bonds on planar graphene and carbon tubes is 143.66 and 105.81 kcal mol−1, respectively, as shown in Table S8.† The dissociation energy of C–F2 binding on carbon tubes is much lower than that on planar graphene, meaning that the curved structure is conducive to activating the C–F2 bonds. Moreover, the dissociation energy of C–F2 bonds binding with a curved structure is close to the reported bond dissociation energy (105.4 kcal mol−1) of common C–F covalent bonds,49 which indicates that the as-synthesized CFNCs derive the discharge process very efficiently to deliver an ultra-high capacity and energy density beyond theoretical limits.
Conclusions
In summary, we have developed a chemical route to synthesize unique CFNCs with remarkable electrochemical properties. The CFNCs possess key structural features such as curved walls composed of smaller graphitic fragments providing additional voids in the walls, which results in high strain, hence making the C–F bond more active. Furthermore, CFNCs bear hollow and open-ended structures allowing easy access to the electrolyte and reservoirs to store electrochemical reaction by-products as well as weaken the C–F covalence by decreasing the overlap between carbon and fluorine orbitals. Chemical analysis confirmed the existence of higher contents of semi-ionic C–F, C–F2 and C–F3 bonds, while low contents of covalent C–F bonds help in achieving high capacity. Consequently, CFNC-360 delivered a high specific capacity of 1056 mA h g−1 together with an energy density of 2487 W h kg−1 at a discharge rate of 0.01C. The high performance is attributed to rich edges and active sites in 3D heterocyclic and high curvature structures which not only improve the F/C ratio but also favor effective electrochemical reaction. The DFT calculations delineate that a higher C/F ratio results in an increased capacity as it pushes the theoretical limits high. Furthermore, DFT calculations showed that –CF2 species remained active and the activity of the F atoms bonded to basal planes is higher than that at the edge. Moreover, the desorption and mobility of LiF on the fluorinated graphene fragments are conducive to discharge. Hence, the CFNC based cathode is the best replacement for existing ones for large scale applications of primary Li/CFx batteries, and can be further tuned to realize secondary Li/CFx batteries due to its versatile chemistry.
Author contributions
Yifan Liu: writing – original draft and data curation; Hongyan Zhang and Baoshan Wu: formal analysis, Jianyi Ma: creation of models and DFT calculations; Guoyun Zhou and Huakun Liu: writing – review & editing; and Nasir Mahmood and Xian Jian: conceptualization, project administration and funding acquisition.
Conflicts of interest
There are no conflicts to declare.
Acknowledgements
The present work was financially supported by the National Natural Science Foundation of China (No. 51972045), the Fundamental Research Funds for the Chinese Central Universities, China (No. ZYGX2019J025), and Sichuan Science and Technology Program (No. 2020JDRC0015 and 2020JDRC0045). The authors would like to acknowledge the Vice-Chancellor fellowship scheme at RMIT University, the ARC Centre of Excellence in Future Low-Energy Electronics Technologies (FLEET) (CE170100039), the RMIT Micro Nano Research Facility (MNRF) in the Victorian node of the Australian National Fabrication Facility (ANFF) and the RMIT Microscopy and Microanalysis Facility (RMMF).
References
- J. L. Wang, M. H. Sun, Y. Liu, J. F. Lin, L. F. Wang, Z. Xu, W. L. Wang, Z. Z. Yuan, J. C. Liu and X. D. Bai, Unraveling nanoscale electrochemical dynamics of graphite fluoride by in situ electron microscopy: key difference between lithiation and sodiation, J. Mater. Chem. A, 2020, 8, 6105–6111 RSC.
- Z. Fang, Y. Peng, X. Zhou, L. Zhu, Y. G. Wang, X. L. Dong and Y. Y. Xia, Fluorinated Carbon Materials and the Applications in Energy Storage Systems, ACS Appl. Energy Mater., 2022, 5, 3966–3978 CrossRef CAS.
- X. Y. Chen, K. Fan, Y. Liu, Y. Li, X. Y. Liu, W. Feng and X. Wang, Recent Advances in Fluorinated Graphene from Synthesis to Applications: Critical Review on Functional Chemistry and Structure Engineering, Adv. Mater., 2022, 34, 2101665 CrossRef CAS.
- L. Wang, J. Qiu, X. Wang, L. Chen, G. Cao, J. Wang, H. Zhang and X. He, Insights for understanding multiscale degradation of LiFePO4 cathodes, eScience, 2022, 2, 125–137 CrossRef.
- P. Y. Chen, C. Jiang, J. Jiang, J. Zou, Q. W. Ran, X. Wang, X. B. Niu and L. P. Wang, Fluorinated Carbons as Rechargeable Li-Ion Battery Cathodes in the Voltage Window of 0.5–4.8 V, ACS Appl. Mater. Interfaces, 2021, 13, 30576–30582 CrossRef CAS PubMed.
- N. Sharma, M. Dubois, K. Guerin, V. Pischedda and S. Radescu, Fluorinated (Nano)Carbons: CFx Electrodes and CFx-Based Batteries, Energy Technol., 2021, 9, 2000605 CrossRef CAS.
- Z. Y. Luo, D. W. Chen, X. Wang, J. Y. Huang, Y. Pan, W. X. Lei and J. A. Pan, Accordion-Like Fluorinated Graphite Nanosheets with High Power and Energy Densities for Wide-Temperature, Long Shelf-Life Sodium/Potassium Primary Batteries, Small, 2021, 17, 2008163 CrossRef CAS PubMed.
- Y. Zhang, X. Li, L. Fan, Y. Shuai and N. Zhang, Ultrathin and super-tough membrane for anti-dendrite separator in aqueous zinc-ion batteries, Cell Rep. Phys. Sci., 2022, 3, 100824 CrossRef CAS.
- K. Yang, Z. Q. Shan, X. S. Liu and S. R. Wang, Effect of MnO2 on expansion force inhibition and electrical properties of Li/CFx battery, Mater. Lett., 2022, 309, 131421 CrossRef CAS.
- J. Ban, X. X. Jiao, Y. Y. Feng, J. Xue, C. He and J. X. Song, All-Temperature, High-Energy-Density Li/CFx Batteries Enabled by a Fluorinated Ether as a Cosolvent, ACS Appl. Energy Mater., 2021, 4, 3777–3784 CrossRef CAS.
- L. T. Chen, X. Y. Yang, X. Q. Wang, G. F. Hu, R. L. Zhang, N. S. Weng and J. S. Zhao, High-Efficiency Electrocatalyst Phthalocyanine in Li/SOCl2 Batteries: From Experimental to Theoretical Investigation, J. Electrochem. Soc., 2021, 168, 120505 CrossRef CAS.
- B. Sayahpour, H. Hirsh, S. Bai, N. B. Schorr, T. N. Lambert, M. Mayer, W. Bao, D. Y. Cheng, M. H. Zhang, K. Leung, K. L. Harrison, W. K. Li and Y. S. Meng, Revisiting Discharge Mechanism of CFx as a High Energy Density Cathode Material for Lithium Primary Battery, Adv. Energy Mater., 2022, 12, 2103196 CrossRef CAS.
- Q. Li, W. R. Xue, X. R. Sun, X. Q. Yu, H. Li and L. Q. Chen, Gaseous electrolyte additive BF3 for high-power Li/CFx primary batteries, Energy Storage Mater., 2021, 38, 482–488 CrossRef.
- C. Jiang, B. J. Wang, Z. R. Wu, J. L. Qiu, Z. P. Ding, J. Zou, S. L. Chen, P. Gao, X. B. Niu, L. P. Wang and H. Li, Electrolyte-assisted dissolution-recrystallization mechanism towards high energy density and power density CF cathodes in potassium cell, Nano Energy, 2020, 70, 104552 CrossRef CAS.
- W. Feng, P. Long, Y. Y. Feng and Y. Li, Two-Dimensional Fluorinated Graphene: Synthesis, Structures, Properties and Applications, Adv. Sci., 2016, 3, 1500413 CrossRef PubMed.
- C. Peng, L. C. Kong, Y. Li, H. Y. Fu, L. D. Sun, Y. Y. Feng and W. Feng, Fluorinated graphene nanoribbons from unzipped single-walled carbon nanotubes for ultrahigh energy density lithium-fluorinated carbon batteries, Sci. China Mater., 2021, 64, 1367–1377 CrossRef CAS.
- J. Hou, X. Yang, X. Fu, D. Zou, J. Ma, Y. Peng, Y. Liu and X. Jian, Highly oriented fluorinated carbon nanotube arrays for high specific capacity lithium primary battery, J. Alloys Compd., 2022, 923, 166452 CrossRef CAS.
- Y. Ahmad, M. Dubois, K. Guérin, A. Hamwi and W. Zhang, Pushing the theoretical limit of Li–CFx batteries using fluorinated nanostructured carbon nanodiscs, Carbon, 2015, 94, 1061–1070 CrossRef CAS.
- Z. Y. Luo, X. Wang, D. W. Chen, Q. H. Chang, S. H. Xie, Z. S. Ma, W. X. Lei, J. A. Pan, Y. Pan and J. Y. Huang, Ultrafast Li/Fluorinated Graphene Primary Batteries with High Energy Density and Power Density, ACS Appl. Mater. Interfaces, 2021, 13, 18809–18820 CrossRef CAS.
- X. X. Yang, G. J. Zhang, B. S. Bai, Y. Li, Y. X. Li, Y. Yang, X. Jian and X. W. Wang, Fluorinated graphite nanosheets for ultrahigh-capacity lithium primary batteries, Rare Met., 2021, 40, 1708–1718 CrossRef CAS.
- R. X. Zhou, Y. Li, Y. Y. Feng, C. Peng and W. Feng, The electrochemical performances of fluorinated hard carbon as the cathode of lithium primary batteries, Compos. Commun., 2020, 21, 100396 CrossRef.
- Y. Matsuo, J. Inamoto, H. Yamamoto, K. Matsumoto and R. Hagiwara, Discharge Characteristic of Fluorinated Graphene-like Graphite as a Cathode of Lithium Primary Battery, Electrochemistry, 2020, 88, 437–440 CrossRef CAS.
- J. Hou, F. Cao, H. Xu, J. Fu, R. Ali, Y. Liu and X. Jian, Constructing carbon-decorated CFx nanocapsule by atomic layer deposition and catalytic chemical vapor deposition for high-capacity lithium primary battery, Appl. Surf. Sci., 2022, 596, 153570 CrossRef CAS.
- J. Ma, Y. F. Liu, Y. Peng, X. X. Yang, J. Hou, C. Liu, Z. W. Fang and X. Jian, UV-radiation inducing strategy to tune fluorinated carbon bonds delivering the high-rate Li/CFx primary batteries, Composites, Part B, 2022, 230, 109494 CrossRef CAS.
- K. Leung, N. B. Schorr, M. Mayer, T. N. Lambert, Y. S. Meng and K. L. Harrison, Edge-Propagation Discharge Mechanism in CFx Batteries—A First-Principles and Experimental Study, Chem. Mater., 2021, 33, 1760–1770 CrossRef CAS.
- G. Chen, H. Zhou, S. Zhang, Z. Zhang, T. Feng, Z. Xu and M. Wu, Surface De-Fluorination and Bond Modification of CF x by High-Density Hydrogen Plasma Processing, ACS Appl. Energy Mater., 2021, 4, 8615–8620 CrossRef CAS.
- Z. Luo, J. Wan, W. Lei, Y. Zhao, Y. Pan and Z. Ma, A simple strategy to synthesis CFx@MnO2-nanowires composite cathode materials for high energy density and high power density primary lithium batteries, Mater. Technol., 2020, 35, 836–842 CrossRef CAS.
- H. M. Zhang, P. Xiao, J. Y. Shi, C. Wang, J. L. Wang, Q. J. Wang, X. T. Chen and B. Shi, Silver-Modified Carbon Fluoride as the Cathode Material for Pouch-Type Primary Lithium Batteries, J. Electron. Mater., 2021, 50, 4075–4082 CrossRef CAS.
- G. T. Chen, H. P. Zhou, S. Zhang, Z. D. Zhang, T. T. Feng, Z. Q. Xu and M. Q. Wu, Surface De-Fluorination and Bond Modification of CFx by High-Density Hydrogen Plasma Processing, ACS Appl. Energy Mater., 2021, 4, 8615–8620 CrossRef CAS.
- B. J. Walder and T. M. Alam, Modes of Disorder in Poly(carbon monofluoride), J. Am. Chem. Soc., 2021, 143, 11714–11733 CrossRef CAS.
- W. Zhang, M. Dubois, K. Guerin, P. Bonnet, H. Kharbache, F. Masin, A. P. Kharitonov and A. Hamwi, Effect of curvature on C-F bonding in fluorinated carbons: from fullerene and derivatives to graphite, Phys. Chem. Chem. Phys., 2010, 12, 1388–1398 RSC.
- Y. Q. Zhang, Y. K. Zhu, D. N. Lan, S. H. Pun, Z. Zhou, Z. Wei, Y. Wang, H. K. Lee, C. Lin, J. P. Wang, M. A. Petrukhina, Q. Li and Q. Miao, Charging a Negatively Curved Nanographene and Its Covalent Network, J. Am. Chem. Soc., 2021, 143, 5231–5238 CrossRef CAS PubMed.
- H. Wang, S. Nie, H. Li, R. Ali, J. A. Fu, H. J. Xiong, J. Li, Z. Q. Wu, W. M. Lau, N. Mahmood, R. Jia, Y. F. Liu and X. Jian, 3D Hollow Quasi-Graphite Capsules/Polyaniline Hybrid with a High Performance for Room-Temperature Ammonia Gas Sensors, ACS Sens., 2019, 4, 2343–2350 CrossRef CAS PubMed.
- R. J. Fan, B. Yang, Z. W. Li, D. D. Ma, W. D. Yuan, J. Y. Ma and H. S. Ren, First-principles study of the adsorption behaviors of Li atoms and LiF on the CFx(x = 1.0, 0.9, 0.8, 0.5, similar to 0.0) surface, RSC Adv., 2020, 10, 31881–31888 RSC.
- P. V. Bakharev, M. Huang, M. Saxena, S. W. Lee, S. H. Joo, S. O. Park, J. C. Dong, D. C. Camacho-Mojica, S. Jin, Y. Kwon, M. Biswal, F. Ding, S. K. Kwak, Z. Lee and R. S. Ruoff, Chemically induced transformation of chemical vapour deposition grown bilayer graphene into fluorinated single-layer diamond, Nat. Nanotechnol., 2020, 15, 59–66 CrossRef CAS PubMed.
- C. Cavallari, S. Radescu, M. Dubois, N. Batisse, H. Diaf and V. Pischedda, Tuning C-F Bonding of Graphite Fluoride by Applying High Pressure: Experimental and Theoretical Study, J. Phys. Chem. C, 2020, 124, 24747–24755 CrossRef CAS.
- Y. Li, J. Cheng, X. Wang, Y. Liu and X. Liu, Thermal stability of C–F/C(–F)2 bonds in fluorinated graphene detected by in situ heating infrared spectroscopy, Phys. Chem. Chem. Phys., 2021, 23, 26853–26863 RSC.
- G. X. Zhang, M. Colin, X. H. Yang, S. H. Sun, J. P. Dodelet and M. Dubois, C-F bonding in fluorinated N-Doped carbons, Appl. Surf. Sci., 2022, 577, 151721 CrossRef CAS.
- V. E. Diyuk, A. N. Zaderko, L. M. Grishchenko, S. Afonin, R. Mariychuk, O. Y. Boldyrieva, V. A. Skryshevsky, M. Kanuchova and V. V. Lisnyak, Surface chemistry of fluoroalkylated nanoporous activated carbons: XPS and F19 NMR study, Appl. Nanosci., 2022, 12, 637–650 CrossRef CAS.
- Z. Jiang, Y. H. Li, C. Han, Z. Q. Huang, X. W. Wu, Z. X. He, W. Meng, L. Dai and L. Wang, Raising Lithium Storage Performances of NaTi2(PO4)3 by Nitrogen and Sulfur Dual-Doped Carbon Layer, J. Electrochem. Soc., 2020, 167, 020550 CrossRef CAS.
- M. E. Zhong, J. D. Guan, Q. J. Feng, X. W. Wu, Z. B. Xiao, W. Zhang, S. Tong, N. Zhou and D. X. Gong, Accelerated polysulfide redox kinetics revealed by ternary sandwich-type S@Co/N-doped carbon nanosheet for high-performance lithium-sulfur batteries, Carbon, 2018, 128, 86–96 CrossRef CAS.
- X. N. Chen, X. H. Wang and D. Fang, A review on C1s XPS-spectra for some kinds of carbon materials, Fullerenes, Nanotubes, Carbon Nanostruct., 2020, 28, 1048–1058 CrossRef CAS.
- C. R. Brundle, B. V. Crist and P. S. Bagus, Accuracy limitations for composition analysis by XPS using relative peak intensities: LiF as an example, J. Vac. Sci. Technol., A, 2021, 39, 013202 CrossRef CAS.
- J. T. Xu, Y. H. Dou, Z. X. Wei, J. M. Ma, Y. H. Deng, Y. T. Li, H. K. Liu and S. X. Dou, Recent Progress in Graphite Intercalation Compounds for Rechargeable Metal (Li, Na, K, Al)-Ion Batteries, Adv. Sci., 2017, 4, 1700146 CrossRef.
- Y. Z. Cai, D. Q. Huang, Z. L. Ma, H. Q. Wang, Y. G. Huang, X. W. Wu and Q. Y. Li, Construction of highly conductive network for improving electrochemical performance of lithium iron phosphate, Electrochim. Acta, 2019, 305, 563–570 CrossRef CAS.
- J. Park, C. W. Lee, J. H. Park, S. H. Joo, S. K. Kwak, S. Ahn and S. J. Kang, Capacitive Organic Anode Based on Fluorinated-Contorted Hexabenzocoronene: Applicable to Lithium-Ion and Sodium-Ion Storage Cells, Adv. Sci., 2018, 5, 1801365 CrossRef.
- F. Rao, Z. Wang, B. Xu, L. Chen and C. Ouyang, First-Principles Study of Lithium and Sodium Atoms Intercalation in Fluorinated Graphite, Engineering, 2015, 1, 243–246 CrossRef CAS.
- W. Zhang, M. Dubois, K. Guerin, P. Bonnet, H. Kharbache, F. Masin, A. P. Kharitonov and A. Hamwi, Effect of curvature on C-F bonding in fluorinated carbons: from fullerene and derivatives to graphite, Phys. Chem. Chem. Phys., 2010, 12, 1388–1398 RSC.
- D. O'Hagan, Understanding organofluorine chemistry. An introduction to the C-F bond, Chem. Soc. Rev., 2008, 37, 308–319 RSC.
|
This journal is © the Partner Organisations 2023 |