DOI:
10.1039/D2QI01949C
(Research Article)
Inorg. Chem. Front., 2023,
10, 201-210
Syntheses of ferrocene-functionalized indium-based metal–organic frameworks for third order nonlinear optical application†
Received
9th September 2022
, Accepted 15th November 2022
First published on 15th November 2022
Abstract
We propose a rigid–flexible synergistic strategy for the syntheses of ferrocene-functionalized indium-based metal–organic frameworks (In-MOFs) through introducing rigid carboxylic acids as auxiliary ligands, where the large cations are used to induce flexible 1,1′-ferrocene dicarboxylic acid (H2FcDCA) in a controllable coordination mode to construct target frameworks. As an illustration of this strategy, a series of In-MOFs based on the H2FcDCA ligand have been successfully synthesized. The introduction of rigid OX2− ligands and large cation template leads to the syntheses of various In-MOFs. Furthermore, introducing functional cations and a ferrocene unit endows them with good third order nonlinear optical properties and redox activity.
Introduction
Metal–organic frameworks (MOFs) have received much attention not only because of their wide applications but also because of their rational design and synthesis.1–11 The design synthesis of 4-connected frameworks is quite important due to their ultrahigh porosity and exceptional stability.6,12 Typically, for example, zeolite imidazole frameworks (ZIFs) are composed of divalent tetrahedral metal centers and imidazole bridged ligands,12–14 and boron imidazolate frameworks (BIFs) are based on monovalent/divalent tetrahedral metal centers, B(imidazole)4 ligands,15,16etc.13,17,18 The interactions between ligands, that is, link–link interaction play an important role in the framework's diversity. In addition, some high-valence metal centers (such as In3+,Ti4+, Zr4+ or Hf4+), especially In3+, have been reported as efficient catalysts in multicomponent reactions,19 and interestingly, they can also act as tetrahedral units (such as InN2(CO2)4, InN4(CO2)4, InN4(CO2)2 or In(CO2)4) by chelating coordination (two or more coordination atoms and forms a chelating ring with a central atom or ion) of organic ligands.20–28 Some indium-based MOFs (In-MOFs) with 4-connected topologies can be reasonably designed and synthesized based on the In3+ tetrahedral center with various ligands.29–33 Considering the local and global charge balance, these frameworks are usually anionic and require cations as a guest, endowing them with ion exchange properties.34–36 At the same time, cations can act as structure direct agents (SDAs) in In-MOFs similar to the organic amines in the formation of zeolites, and they can also act as active units to improve their performance.37,38 Similarly, the introduction of functional units on the ligand can also broaden their applications, for instance, homochiral In-MOFs have been developed by using chiral ligands.39,40 However, considering the diversity of ligands, there is still great potential to construct In-MOFs based on new functional ligands.
1,1′-Ferrocene dicarboxylic acid (H2FcDCA) has been widely studied as a functional ligand due to its excellent reduction–oxidation and photoelectric activity.41–49 Nevertheless, limited by its flexible conformation and uncontrollable coordination mode, a large number of low-dimensional structures and clusters are usually obtained instead of MOFs.50–53
In this work, we propose a rigid–flexible synergistic strategy for the syntheses of In-MOFs, that is, introducing rigid carboxylic acids as auxiliary ligands as well as large cations to induce FcDCA2− to construct target frameworks in a controllable coordination mode. As an illustration of this strategy, a series of In-MOFs based on the H2FcDCA ligand have been successfully synthesized, namely, (TPP)+2[In2(FcDCA)3(OX2−)]·5H2O (1, TPP = tetraphenylphosphine; OX2− = oxalate); (TPP)+[In(FcDCA)2]·5H2O (2); (Hbpy)+[In(FcDCA)(OX2−)]·H2O (3, bpy = 4,4′-bipyridine); (MB)+[InCl(FcDCA)(OX2−)0.5] (4, MB = methylene blue); (TPP)+[InCl(FcDCA)(OX2−)0.5] (5); (TPP)+[InCl2(FcDCA)(H2O)]·H2O (6); and [In(FcDCA)(Im)(H2O)] (7, HIm = imidazole). The introduction of rigid OX2− ligands and a large cation template leads to the syntheses of various frameworks. Furthermore, the presence of functional cations and a ferrocene unit endows them with good third order nonlinear optical properties and redox activity.
Experimental
Materials and instruments
All chemicals were commercially purchased and directly used without further purification. PXRD data were collected on a Rigaku Mini Flex II diffractometer using Cu-Kα radiation (λ = 1.54056 Å) under ambient conditions. The UV-vis diffuse reflection data were recorded at room temperature using a powder sample with BaSO4 as a standard (100% reflectance) on a PerkinElmer Lamda-950 UV spectrophotometer and scanned at 200–800 nm. FT-IR spectra were recorded on KBr pellets on a Bruker VERTEX70 FT-IR spectrometer with the range of 400–4000 cm−1. Thermal stability studies were carried out on a Netschz STA449F3 thermal analysis system with a heating rate of 10 K min−1 under a N2 atmosphere. The gas adsorption isotherms were measured by using ASAP-2020 volumetric adsorption equipment. Fluorescence spectra (FL) and the luminescence decay curves were performed on an Edinburgh FLS1000 fluorescence spectrometer. 1H NMR spectra were recorded on a Bruker AVANCE III spectrometer (400 MHz).
Synthesis of (TPP)+2[In2(FcDCA)3(OX2−)]·5H2O (1)
A mixture of oxalic acid (9.0 mg, 0.10 mmol), TPPBr (42.0 mg, 0.10 mmol), imidazole (7.0 mg, 0.10 mmol), H2FcDCA (27.0 mg, 0.10 mmol), In(NO)3·xH2O (60.0 mg, 0.20 mmol), NaOH (8.0 mg, 0.20 mmol), and H2O (5.0 ml) was added to 23 ml glass vials, respectively, sealed with ultrasound for 5 minutes, and heated in a100 °C oven for 1 day to generate yellow crystals (yield: 8.0%). Elem anal. found: C, 55.3; H, 3.8. Calc. for C86H74Fe3In2O21P2: C, 54.2; H, 3.9%. FTIR (KBr, cm−1): 3480 (v), 3079 (m), 2360 (m), 1646 (s), 1581 (s), 1476 (m), 1453 (m), 1342 (s), 1308 (s), 1187 (s), 1104 (s), 1031 (s), 979 (v), 844 (m), 797 (w), 720 (s), 690 (s), 524 (m) (Fig. S1†). The crystals are decomposed in trifluoroacetic acid and then the test was carried out: 1H NMR (400 MHz, DMSO-d6) δ 7.88 (t, J = 7.0 Hz, 3H, TPP+), 7.74–7.65 (m, 12H, TPP+), 4.66 (s, 4H, FcDCA2−), 4.38 (s, 4H, FcDCA2−) (Fig. S2–S4†).
Synthesis of (TPP)+[In(FcDCA)2]·5H2O (2)
A mixture of L-tartaric acid (15.0 mg, 0.10 mmol), TPPBr (42.0 mg, 0.10 mmol), H2FcDCA (27.0 mg, 0.10 mmol), In(NO)3·xH2O (60.0 mg, 0.20 mmol), NaOH (8.0 mg, 0.20 mmol), and H2O (5 ml) was added to 23 ml glass vials, respectively, sealed with ultrasound for 5 minutes, and heated in a 100 °C oven for 1 day and yellow crystals were obtained (yield: 25.0%). Elem anal. found: C, 53.1; H, 4.2. Calc. for C48H46Fe2InO13P: C, 52.9; H, 4.4%. FTIR (KBr, cm−1): 3450 (v), 3084 (m), 2356 (m), 1650 (s), 1576 (s), 1490 (m), 1447 (m), 1373 (m), 1338 (s), 1182 (s), 1091 (s), 1013 (s), 983 (v), 820 (m), 736 (s), 723 (s), 680 (s), 515 (m). 1H NMR (400 MHz, DMSO-d6) δ 7.86 (t, J = 7.0 Hz, 3H, TPP+), 7.73–7.64 (m, 12H, TPP+), 4.65 (s, 4H, FcDCA2−), 4.37 (s, 4H, FcDCA2−) (Fig. S5†).
Synthesis of (Hbpy)+[In(FcDCA)(OX2−)]·H2O (3)
A mixture of oxalic acid (9.0 mg, 0.1 mmol), 4,4-bipyridine (bpy, 16.0 mg, 0.10 mmol), H2FcDCA (27.0 mg, 0.10 mmol), In(NO)3·xH2O (60.0 mg, 0.20 mmol), NaOH (8.0 mg, 0.20 mmol), and H2O (5 ml) was added to 23 ml glass vials, respectively, sealed with ultrasound for 5 minutes, and heated in a 100 °C oven for 1 day and yellow crystals were obtained (yield: 16.0%). Elem anal. found: C, 43.9; H, 3.5; N, 4.1. Calc. for C24FeInN2O9H19: C, 44.3; H, 3.2; N, 4.3%. FTIR (KBr, cm−1): 3482 (v), 3105 (v), 2343 (m), 1685 (s), 1620 (v), 1567 (w), 1473 (s), 1382 (m), 1317 (s), 1178 (s), 1087 (s), 1005 (v), 800 (s), 693 (s), 576 (s), 490 (s). 1H NMR (400 MHz, DMSO-d6) δ 9.13 (s, 6H, bpy), 8.55 (s, 6H, bpy), 4.65 (s, 4H, FcDCA2−), 4.37 (s, 4H, FcDCA2−) (Fig. S6†).
Synthesis of (MB)+[InCl(FcDCA)(OX2−)0.5] (4)
A mixture of oxalic acid (9.0 mg, 0.10 mmol), MB (C16H18ClN3S, 32.0 mg, 0.10 mmol), H2FcDCA (27.0 mg, 0.10 mmol), In(NO)3·xH2O (60.0 mg, 0.20 mmol), NaOH (8.0 mg, 0.20 mmol), and H2O (5 ml) was added to 23 ml glass vials, respectively, sealed with ultrasound for 5 minutes, and heated in a 100 °C oven for 3 days and yellow crystals were obtained (yield: 31.0%). Elem anal. found: C, 45.9; H, 3.8; N, 5.2. Calc. for C29H26ClFeInN3O6S: C, 46.1; H, 3.6; N, 5.3%. FTIR (KBr, cm−1): 2356 (m), 1646 (v), 1529 (s), 1476 (s), 1430 (m), 1384 (m), 1325 (s), 1234 (s), 1135 (s), 1040 (s), 940 (m), 879 (w), 797 (m), 670 (s), 494 (s), 430 (s). Methylene blue is an alkaline dye that may decompose in trifluoroacetic acid. There is no characteristic peak of methylene blue in 1H NMR but there is a characteristic peak of the FcDCA2− ligand. 1H NMR (400 MHz, DMSO-d6) δ 4.66 (s, 4H, FcDCA2−), 4.38 (s, 4H, FcDCA2−) (Fig. S7†).
Synthesis of (TPP)+[InCl(FcDCA)(OX2−)0.5] (5)
A mixture of oxalic acid (OX; 2.0 mg, 0.02 mmol), TPPBr (42.0 mg, 0.10 mmol), H2FcDCA (27.0 mg, 0.10 mmol), InCl3·4H2O (60 mg, 0.20 mmol), NaOH (16.0 mg, 0.40 mmol), and H2O (4 ml) was added to 23 ml glass vials, respectively, sealed with ultrasound for 5 minutes, and heated in a 100 °C oven for 7 days to generate several yellow plate crystals of 5 and a large amount of unidentified precipitate. Their crystal structures are described below.
Synthesis of (TPP)+[InCl2(FcDCA)(H2O)]·H2O (6)
A mixture of TPPBr (42.0 mg, 0.10 mmol), H2FcDCA (27.0 mg, 0.10 mmol), InCl3·4H2O (60 mg, 0.20 mmol), NaOH (16.0 mg, 0.40 mmol), and H2O (4 ml) was added to 23 ml glass vials respectively, sealed with ultrasound for 5 minutes, and heated in a 100 °C oven for 7 days to generate several yellow plate crystals of 6 and a large amount of unidentified precipitate. Their crystal structures are described below.
Synthesis of [In(FcDCA)(Im)(H2O)] (7)
A mixture of TPPBr (42.0 mg, 0.10 mmol), imidazole (7.0 mg, 0.10 mmol), H2FcDCA (27.0 mg, 0.10 mmol), In(NO)3·xH2O (60.0 mg, 0.20 mmol), 5 ml of formamide and polyethylene glycol (v
:
v, 1
:
1) was added to 23 ml glass vials, respectively, sealed with ultrasound for 5 minutes, and heated in a 100 °C oven for 3 days and yellow crystals were obtained (yield: 20%). Elem anal. found: C, 37.6; H, 2.5; N, 6.1. Calc. for C15H13FeInN2O5: C, 37.4; H, 2.7; N, 5.9%. FTIR (KBr, cm−1): 3460 (v), 3310 (s), 3150 (m), 1675 (s), 1496 (m), 1393 (s), 1347 (s), 1238 (s), 1195 (m), 1078 (s), 1031 (m), 944 (s), 823 (m), 801 (m), 654 (s), 597 (m), 550 (s), 494 (s). 1H NMR (400 MHz, DMSO-d6) δ 7.76 (s, 1H, Im−), 7.26 (s, 2H, Im−), 4.64 (s, 4H, FcDCA2−), 4.36 (s, 4H, FcDCA2−) (Fig. S8†).
X-Ray crystallographic analysis
Single crystal X-ray diffraction data of porous materials were collected using a Hybrid Pixel Array detector equipped with Ga-Kα radiation (λ = 1.3405 Å) at about 298 K and 100 K. The structures were solved by dual-direct methods using ShelXT and refined by the full-matrix least-squares technique based on F2 using SHELXL.54 Non-hydrogen atoms were refined anisotropically. Hydrogen atoms were added theoretically, riding on the concerned atoms and refined with fixed thermal factors. All absorption corrections were performed using the multi-scan program.
Z-scan measurements
The Z-scan technique was applied to investigate the third order nonlinear optical (NLO) behaviour of the samples with an output wavelength of 532 nm. A nanosecond light source irradiated by a PL2250 laser (EKSPLA) was a Q-switched Nd:YAG pulsed laser system with a repetition frequency of 10 HZ. For the practical application of NLO, the hybrid transparent and flexible films were prepared by ultrasonic stripping and dispersing crystal materials into the polydimethylsiloxane (PDMS) matrix by the literature reported method.55
Results and discussion
Single crystal X-ray diffraction analysis shows that compound 1 crystallizes in the monoclinic C2/c space group. The asymmetric unit of 1 contains two In(III) ions, and three FcDCA2−, one oxalate, two cationic guest TPP+ and five guest water molecules. Each In(III) ion is 8-coordinated with eight carboxyl oxygen atoms of three FcDCA2− ligands and one OX2− ligand (Fig. 1a). The In–O bond distance ranges from 2.154 Å to 2.572 Å (Table S2†). Among the three FcDCA2− ligands, carboxyl torsion angle of two FcDCA2− ligands is 152.306°, and the other one is 180° (Fig. 1b). Two oxygen atoms of the same carboxyl group of the three FcDCA2− ligands are coordinated to the indium metal in the form of chelate coordination. The OX2− ligand also acts as a bridging ligand to coordinate on two In atoms.
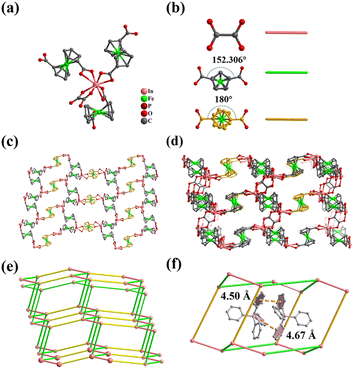 |
| Fig. 1 (a) Coordination environment of the In atom in 1; (b) the OX2− ligand and FcDCA2− ligands with different torsion angles are simplified as linkers; (c) the 2D layer is constructed from In atoms and FcDCA2− ligands; (d) the 3D pillar-layered framework of 1; (e) the dia topology of 1; and (f) π⋯π interaction of two TPP+ in one dia cage. | |
The main structural feature of 1 is its zeolitic framework. Firstly, each In atoms is linked by three FcDCA2− ligands to form a 2D honeycomb-like layer along the bc plane (Fig. 1c). Then these layers are pillared by OX2− ligands to form a pillar-layered 3D framework (Fig. 1d). As expected, each In atom acts as a 4-connected node, and the whole framework can be simplified into the dia topology (Fig. 1e).56 Each dia cage of 1 is filled with two TPP+ with weak π⋯π interactions to balance the charge (Fig. 1f), making it nonporous.
2 has been synthesized by using flexible L-tartaric acid to replace rigid OX2− under similar conditions. Only FcDCA2− ligands are observed in 2 which may be attributed to the weak coordination ability of L-tartaric acid. Similarly, each In(III) ion of 2 is coordinated by four FcDCA2− ligands as a 4-connected node (Fig. 2a), but it is connected by four FcDCA2− linkers to form a 2D layer (Fig. 2b) with sql topology. These layers are stacked in the ABAB mode (Fig. 2c) and the porosity of 2 is 10.8%. TPP+ cations are located between layers. There are weak π⋯π interactions between TPP+ cations (Fig. 2d). Comparing these two compounds, we found that in the presence of large TPP+ cations, flexible FcDCA2− ligands and rigid OX ligands cooperate to form a 3D framework (1), while flexible FcDCA2− ligand tends to form the 2D layer (2) instead of the 3D framework, which is similar to the layer previously obtained with protonated 4,4-bipyridine cations.51 This is may be attributed to the difference in the torsion angle. Two carboxyl groups of FcDCA2− ligands form torsion angles of 143.535° and 157.248° in 2, which is smaller than that of 1.
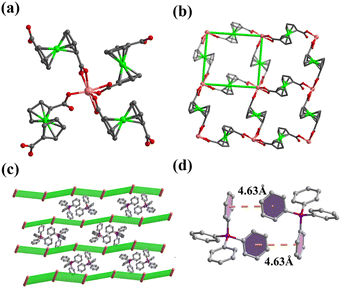 |
| Fig. 2 (a) Coordination environment of In atom in 2; (b) the 2D layer of 2 based on In atoms and FcDCA2− ligands; (c) packing structure of 2; and (d) π⋯π interaction of TPP+ in 2. | |
To test the universality of this rigid–flexible synergy strategy, compounds 3 to 7 have been synthesized through changing cations and rigid ligands.
As mentioned above, we previously reported the FcDCA2− based 2D layer with protonated 4,4-bipyridine cations in the presence of 4,4-bipyridine.51 In this work, compound 3 with a 3D framework is obtained by using 4,4-bipyridine to replace TPPBr in 1. The asymmetric unit of 3 contains an indium(III) ion, one FcDCA2−, one OX2−, one protonated Hbpy+ cation and one guest water molecule. Each In(III) ion is 8-coordinated by two FcDCA2− ligands and two OX2− ligands (Fig. 3a). The In–O bond length ranges from 2.201 Å to 2.582 Å (Table S4†). The torsion angle between two carboxyl groups of the FcDCA2− is 143.419° which is close to that in 1.
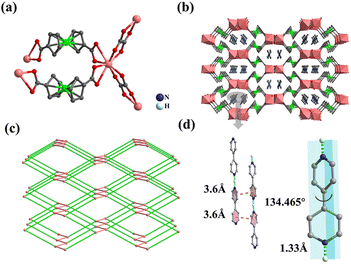 |
| Fig. 3 (a) Coordination environment of the In atom in 3; (b) 3D framework of 3; (c) the dia topology of 3, FcDCA2− linker: green; OX2− linker: pink; and (d) the π⋯π and hydrogen-bonding interactions of the bipyridine cations in 3. | |
In 3, both ligands act as bridging ligands to connect In atoms to form a dia-type 3D framework (Fig. 3b and c) containing 1D channel along the b-axis, which is filled with Hbpy+ cations. Interestingly, the Hbpy+ cations formed a 1D chain through H-bond interactions (Fig. 3d). In addition, strong π⋯π interaction between adjacent pyridine rings is also observed. Each Hbpy+ has a certain degree of torsion, and the torsion angle is 134.465°. Compared with 1, the ratio of the FcDCA2− ligand to oxalic acid changes from 3
:
1 to 2
:
2, and the presence of smaller Hbpy+ cations improves the porosity of 3 (20%).
When larger methylene blue is introduced into the reaction system, 1D ladder-like 4 is obtained, which indicates that the appropriate template size is also important. In 4, each In atom is coordinated by two FcDCA2−, one OX2− and one Cl anion (Fig. 4a). The In atom is connected by FcDCA2− to form a 1D chain, which is linked by OX2− to form a ladder-like structure. The Cl anions are pendent on the ladder which hinders the formation of the framework (Fig. 4b). The guest MB+ of 4 is stuck in the middle of each ladderlike chain with a strong π⋯π interaction (Fig. 4c and d). In addition, methylene blue with a strong absorption chromophore makes 4 fluorescent (Fig. S9†).
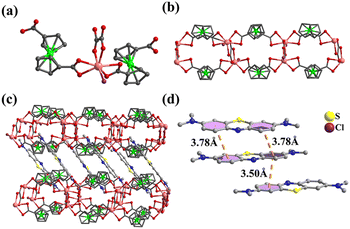 |
| Fig. 4 (a) Coordination environment of In atom in 4; (b) the 1D ladder of 4; (c) packing structure of 4; and (d) π⋯π interaction between MB in 4. | |
It should be noted that the introduction of a Cl anion from MB is not conducive to the formation of the framework. The employment of InCl3·4H2O to replace In(NO)3·xH2O also leads to similar results, 5 and 6. The coordination environment and structure of 5 is the same as 4 (Fig. 5a, c and e). The difference is that the guest of 5 is TPP+ with a weak π⋯π interaction (Fig. 5g). In 6, one OX2− is replaced by Cl− and one coordinated water (Fig. 5b). Therefore, each In atom is connected by FcDCA2− to form a 1D chain (Fig. 5d). Hydrogen bonds (O4–H4B⋯O6, the angle of the O–H⋯O is 144.5°, the distance of O⋯O is 2.773 Å (Table S8†)) between coordinating water and carboxylate oxygen of the FcDCA2− ligand are observed, which link adjacent chains to form a 2D layer (Fig. 5f). TPP+ cations lie between these layers with weak π⋯π interactions (Fig. 5h).
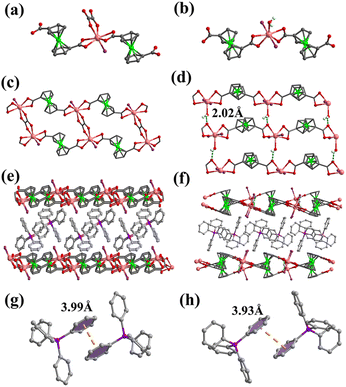 |
| Fig. 5 Structure comparison of 5 and 6: coordination environment of In atoms in 5 (a) and 6 (b); the 1D ladder of 5 (c); and hydrogen bonded 2D layer of 6 (d); packing structure of 5 (e) and 6 (f); and the π⋯π interactions of TPP+ in 5 (g) and 6 (h). | |
Besides OX2−, imidazole commonly used in ZIFs is also chosen as a rigid auxiliary ligand to check the possibility. By adding imidazole molecules to the solvothermal system, 7 is synthesized. The In atom of 7 is 7-coordinated by four oxygen atoms from two FcDCA2− ligands, two nitrogen atoms from two imidazole ligands and one coordinated water (Fig. 6a). Two carboxylates of the FcDCA2− ligand form a small torsion angle of 50.423° and two twisted FcDCA2− ligands coordinate on two In atoms to generate one In2(FcDCA)2 unit (Fig. 6b), which is similar to the former results.51 Differently, the In2(FcDCA)2 unit is bridged by four imidazole ligands to form a 2D layer with sql topology (Fig. 6c and d). The porosity of 7 is 24.7%. The permanent porosity of 2, 3 and 7 was confirmed by the reversible CO2-adsorption measurement at 195 K (Fig. S10†). Tentatively, we think that the huge differences between the imidazole ligand and carboxylic acid make them mismatched and the target framework cannot be obtained.
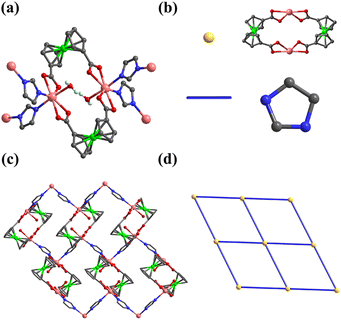 |
| Fig. 6 (a) Coordination environment of In atom in 7; (b) the In2(FcDCA)2 unit as 4-connected node (yellow) and im ligands as the linker (blue); (c) 2D layer of 7; and (d) the sql topology of 7. | |
Here we make a brief summary of the synthesis and structure:
(1) the rigid–flexible synergy strategy is effective for the synthesis of ferrocene-functionalized In-MOFs;
(2) rigid carboxylic acids will be good auxiliary ligands than that of the flexible one and azolate ligands;
(3) cations of suitable size can have positive effects;
(4) compared with chloride, indium nitrate may be more suitable for the synthesis of In-MOFs.
Based on these findings, more exploratory synthesis toward In-MOFs with 4-connected zeolite topologies is under way.
The phase purity of samples 1 to 4 and 7 can be confirmed by powder X-ray diffraction (PXRD) (Fig. S11†). In order to study the thermal stability of these complexes, we carried out thermal analysis (TGA) of compounds 1 to 4 and 7. TGA curve shows that both 1 and 2 have two obvious loss steps: the weight loss between room temperature and ∼110 °C corresponds to the removal of free water molecules in the crystal. The crystal material between ∼110 and ∼310 °C is stable and then starts to decompose (Fig. S12†). Thermogravimetric analysis of 3 exhibits that the first weight loss ratio from room temperature to 100 °C is 8.31%, which may be the loss of water molecules. The crystal material between 100 and 210 °C is stable. After 210 °C, 3 starts to decompose and the OX2− may be removed first (6.77%). The thermal stability of 4 is not very good, which decomposes from the beginning of heating and the first continuous weight loss may occur for OX2−. Thermogravimetric analysis of 7 exhibits that the first continuous weight loss ratio from room temperature to 160 °C is 2.40%, which may be ascribed to the loss of free high boiling point solvent guest. And then 7 starts to decompose, in which Im− is removed first (13.60%).
Third order nonlinear optical measurement
It is well-known that the ferrocene unit with an electron rich ring conjugation system and large cations with π⋯π stacking may exhibit nonlinear optical effects.57–62 Therefore we further studied the third order nonlinear optical (NLO) properties of compounds 1 to 4 and 7. In order to ensure structural stability, the crystals of these materials were dispersed in the PDMS thin film. By using Z-scan technology and a 532 nm nanosecond laser, PDMS thin films were found to have no detectable NLO response. Under the same test conditions, the minimum normalized transmittance (Tmin) of 1 to 4 and 7 at the focal point (Z = 0) showed the following order: 1 > 2 > 4 > 3 > 7 (Fig. 7a and b). Compounds 1 to 4 and 7 exhibited significant nonlinear optical response and reverse saturated absorption (RSA).63 We believe that the clear RSA response of these crystal materials is mainly attributed to the FcDCA2− ligands with nonlinear optical properties and the π⋯π stacking between the guests. Compared with 4 and 7, the RSA response of 1 and 2 is slightly enhanced, the reason may be that the number of FcDCA2− ligands in each unit cell is the largest. Compared with 7, 3 and 4 has a slightly stronger response, which may be due to the strong π⋯π interaction of guest stacking that increases the electron cloud density, which is conducive to the excited transition of electrons. The performance of 4 is better than 3 because the π⋯π distance of the MB+ stacking is closer and the π⋯π. interaction is slightly stronger (Fig. 3d and 4d). The plots of output fluence versus input fluence calculated from the corresponding Z-scan curves reveal a typical optical limiting (OL) behaviour (Fig. 7c). To evaluate the NLO responses quantitatively, nonlinear absorption coefficients (β) of 1 to 4 and 7 were calculated by fitting nonlinear related equations, and the corresponding values of β were estimated to be ∼2.8 × 10−9, ∼2.1 × 10−9, ∼1.7 × 10−9, ∼0.7 × 109, and ∼0.3 × 10−10 m W−1 (Fig. 7d), respectively, where the positive values imply the RSA behaviour of the samples.
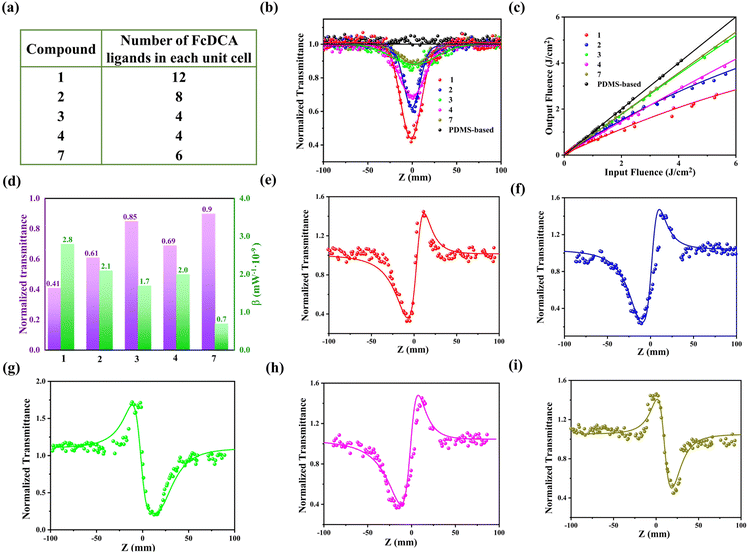 |
| Fig. 7 (a) number of FcDCA ligands in each unit cell; (b) the open-aperture Z-scan spectrum of 1 to 4, 7 and PDMS; (c) the curves of output fluence versus input fluence of 1 to 4, 7 and PDMS; (d) comparison of nonlinear transmittance and nonlinear absorption coefficients (β); nonlinear refractive response of 1 (e), 2 (f) and 4 (h) shows valley peak trend indicating their self-focusing behavior; 3 (g) and 7 (i) show a clear peak-to-trough trend, indicating their self-defocusing behavior. | |
In addition, we performed closed-aperture Z-scan measurement of these MOFs (Fig. 7e–i). χ values were calculated by the formula55 and the order is from ∼2.03 × 10−10 to ∼6.77 × 10−10 esu (Table 1). The results indicate the relatively low Tmin (∼0.41) and third-order NLO susceptibility of 1 in comparison with other MOF-based materials (Table 2), thus making this MOF a particularly attractive third-order NLO material.
Table 1 Linear and NLO data of 1 to 4 and 7. Tmin: linear transmittance; β: nonlinear coefficient; n2: nonlinear refractive coefficients; Im χ(3): the imaginary parts of the third-order susceptibility; Re χ(3): the real parts of the third-order susceptibility; χ(3): the absolute value of the third-order susceptibility
Samples |
T
min
|
β (×10−9 m W−−1) |
n
2 (×10−16 m2 W−1) |
Im χ(3) (×10−11 esu) |
Re χ(3) (×10−10 esu) |
χ
(3) (×10−10 esu) |
1
|
∼0.41 |
∼2.80 |
∼4.40 |
∼2.51 |
∼6.77 |
∼6.77 |
2
|
∼0.61 |
∼2.10 |
∼4.10 |
∼2.34 |
∼5.08 |
∼5.59 |
3
|
∼0.85 |
∼1.70 |
∼−3.10 |
∼−1.77 |
∼−4.11 |
∼4.47 |
4
|
∼0.69 |
∼2.00 |
∼3.72 |
∼2.12 |
∼4.84 |
∼5.28 |
7
|
∼0.90 |
∼0.70 |
∼−2.00 |
∼−1.14 |
∼−1.69 |
∼2.03 |
Table 2
β value and normalized transmittance (Tmin) of some related crystal materials
Compound |
T
min
|
β (×10−9 m W−−1) |
χ
(3) (×10−10 esu) |
Ref. |
ZnTPyP(Cu) |
∼0.61 |
∼5.7 × 103 |
∼5.43 × 103 |
55
|
ZnTPyP(Ni) |
∼0.81 |
∼1.8 × 103 |
∼4.07 × 103 |
55
|
[(Tp*WS3Cu3)2(Btta)3] |
0.71 |
2 × 10−4 |
2 × 10−9 |
66
|
{[(Tp*WS3Cu3)2(Petta)3]BF4} |
0.67 |
3 × 10−4 |
2 × 10−9 |
66
|
[Tp*W(μ3-S)3(μ3-S′)Cu3]4 |
0.8 |
1 × 10−3 |
1.8 × 10−9 |
66
|
[Et4N]{(Tp*WS3Cu3)[Cu2(CN)4.5]}2·2PhCH2CN |
0.64 |
6 × 10−6 |
2.2 × 10−9 |
66
|
[NH4][WS4Cu4(dpypy)Cu(CN)4]·DMF |
0.8 |
0.08 |
3.4 × 10−18 |
67
|
[NH4][WS4Cu4(tpb)Cu2(CN)5]·DMF |
0.92 |
0.012 |
7.4 × 10−19 |
67
|
1
|
∼0.41 |
∼2.8 |
∼6.77 |
This work |
2
|
∼0.61 |
∼2.1 |
∼5.59 |
This work |
3
|
∼0.85 |
∼1.7 |
∼4.47 |
This work |
4
|
∼0.69 |
∼2.0 |
∼5.28 |
This work |
7
|
∼0.9 |
∼0.7 |
∼2.03 |
This work |
Photocurrent measurement
Besides the optical limiting response, the presence of a ferrocene unit drives us to study its photo- and redox-activity. The UV-visible diffuse reflectance results show that compounds 1 to 4 and 7 have strong light absorption in the visible light region and the ultraviolet light region, which are consistent with their colours (Fig. S13–S17†). Their photocurrent responses are studied under visible light irradiation (Fig. S18†). In order to eliminate errors caused by uneven film thickness, a back illumination method is used. During the test, on–off cycle irradiation from a xenon lamp was used (with an interval of 10 s) with a manual shield, and the voltage was maintained at 0.2 V. Obvious photocurrent directions were observed for compounds 1 to 4 and 7. On the one hand, the photocurrent generated rapidly and remained stable, and the intensity did not decrease significantly, indicating that they exhibit a good photoelectric response and high photophysical stability. On the other hand, we find that the response effects exhibit the following tendency: 4 > 2 > 7 > 1 ≈ 3. The difference in the photocurrent response intensity of compounds 1, 2, 3 and 7 does not exceed 0.1 μA cm−−2, which may be the result of the combined action of the molecular structure, light absorption and effective charge transfer.64,65 The relatively strong photocurrent response of compound 4 may be due to the introduction of a strong chromophore methylene blue guest, which enhances the light absorption of compound 4 and reduces the band gap (Fig. S16†).
Redox activity
Their redox activity is also studied. In the reversible redox process, the ferrocene building block ([FeII(Cp)2]) is oxidized to ferrocenium ion ([FeIII(Cp)2]+) and subsequently reduced to [FeII((Cp)2]. Compounds are coated on FTO conductive glass (20th cycle). Different compounds have different signal intensities. 1 to 4 and 7 can detect a reversible redox process (the 20th cycle), with an oxidation peak of 0.505 V–0.534 V and a reduction peak of 0.404 V–0.410 V (Fig. S19†). This redox process can be assigned to the reversible oxidation [FeII(Cp)2]/[FeIII(Cp)2]+ of FcDCA2−.
Conclusions
In this work, we demonstrate the rigid–flexible synergistic strategy for the syntheses of ferrocene-functionalized indium-based MOFs (In-MOFs) through the introduction of rigid carboxylic acids as auxiliary ligands as well as large cations to induce flexible 1,1′-ferrocene dicarboxylic acid (H2FcDCA) to construct target frameworks. The target compounds exhibit various frameworks and good third order nonlinear optical properties and redox activity. Therefore, the present work offers a valuable guidance for the development of In-MOFs exhibiting optical properties and redox activity.
Conflicts of interest
There are no conflicts to declare.
Acknowledgements
We gratefully acknowledge the financial support from NSFC (21971241 and 21871050).
References
- J.-B. Lin, T. T. T. Nguyen, R. Vaidhyanathan, J. Burner, J. M. Taylor, H. Durekova, F. Akhtar, R. K. Mah, O. Ghaffari-Nik, S. Marx, N. Fylstra, S. S. Iremonger, K. W. Dawson, P. Sarkar, P. Hovington, A. Rajendran, T. K. Woo and G. K. H. Shimizu, A scalable metal-organic framework as a durable physisorbent for carbon dioxide capture, Science, 2021, 374, 1464–1469 CrossRef CAS PubMed.
- Y. Su, K. I. Otake, J. J. Zheng, S. Horike, S. Kitagawa and C. Gu, Separating water isotopologues using diffusion-regulatory porous materials, Nature, 2022, 611, 289–294 CrossRef CAS PubMed.
- N. Li, Z. Chang, M. Zhong, Z.-X. Fu, J. Luo, Y.-F. Zhao, G.-B. Li and X.-H. Bu, Functionalizing MOF with redox-active tetrazine moiety for improving the performance as cathode of Li–O2 batteries, CCS Chem., 2021, 3, 1297–1305 CrossRef CAS.
- G. Cai, P. Yan, L. Zhang, H.-C. Zhou and H.-L. Jiang, Metal-organic framework-based hierarchically porous materials: Synthesis and applications, Chem. Rev., 2021, 121, 12278–12326 CrossRef CAS.
- T. He, X.-J. Kong, Z.-X. Bian, Y.-Z. Zhang, G.-R. Si, L.-H. Xie, X.-Q. Wu, H. Huang, Z. Chang, X.-H. Bu, M. J. Zaworotko, Z.-R. Nie and J.-R. Li, Trace removal of benzene vapour using double-walled metal-dipyrazolate frameworks, Nat. Mater., 2022, 21, 689–695 CrossRef CAS.
- J. Yang, Y.-B. Zhang, Q. Liu, C. A. Trickett, E. Gutierrez-Puebla, M. A. Monge, H. Cong, A. Aldossary, H. Deng and O. M. Yaghi, Principles of designing extra-large pore openings and cages in zeolitic imidazolate frameworks, J. Am. Chem. Soc., 2017, 139, 6448–6455 CrossRef CAS PubMed.
- Y.-X. Tan, F. Wang and J. Zhang, Design and synthesis of multifunctional metal-organic zeolites, Chem. Soc. Rev., 2018, 47, 2130–2144 RSC.
- S. Krause, N. Hosono and S. Kitagawa, Chemistry of soft porous crystals: structural dynamics and gas adsorption properties, Angew. Chem., Int. Ed., 2020, 59, 15325–15341 CrossRef CAS PubMed.
- Y. Wang, S. Wang, Z.-L. Ma, L.-T. Yan, X.-B. Zhao, Y.-Y. Xue, J.-M. Huo, X. Yuan, S.-N. Li and Q.-G. Zhai, Competitive coordination-Oriented monodispersed ruthenium sites in conductive MOF/LDH hetero-nanotree catalysts for efficient overall water splitting in alkaline media, Adv. Mater., 2022, 34, 2107488 CrossRef CAS.
- Y. Zhu, J. Gu, X. Yu, B. Zhang, G. Li, J. Li and Y. Liu, The multifunctional design of metal-organic framework by applying linker desymmetrization strategy: synergistic catalysis for high CO2-epoxide conversion, Inorg. Chem. Front., 2021, 8, 4990–4997 RSC.
- X.-X. Wu, J.-X. Wei, T. Zhang, Y. Yang, Q. Liu, X. Yan and Y. Tang, Novel synthesis of in situ CeOx nanoparticles decorated on CoP nanosheets for highly efficient electrocatalytic oxygen evolution, Inorg. Chem. Front., 2021, 8, 4440–4447 RSC.
- H. Wang, X. Pei, M. J. Kalmutzki, J. Yang and O. M. Yaghi, Large cages of zeolitic imidazolate frameworks, Acc. Chem. Res., 2022, 55, 707–721 CrossRef CAS PubMed.
- J.-P. Zhang, Y.-B. Zhang, J.-B. Lin and X.-M. Chen, Metal azolate frameworks: from crystal engineering to functional materials, Chem. Rev., 2012, 112, 1001–1033 CrossRef CAS.
- S. Guo, H.-Z. Li, Z.-W. Wang, Z.-Y. Zhu, S.-H. Zhang, F. Wang and J. Zhang, Syntheses of new zeolitic imidazolate frameworks in dimethyl sulfoxide, Inorg. Chem. Front., 2022, 9, 2011–2015 RSC.
- H.-X. Zhang, M. Liu, T. Wen and J. Zhang, Synthetic design of functional boron imidazolate frameworks, Coord. Chem. Rev., 2016, 307, 255–266 CrossRef CAS.
- H.-X. Zhang, Q.-L. Hong, J. Li, F. Wang, X. Huang, S. Chen, W. Tu, D. Yu, R. Xu, T. Zhou and J. Zhang, Isolated square-planar copper center in boron imidazolate nanocages for photocatalytic reduction of CO2 to CO, Angew. Chem., Int. Ed., 2019, 58, 11752–11756 CrossRef CAS PubMed.
- M. Eddaoudi, D. F. Sava, J. F. Eubank, K. Adil and V. Guillerm, Zeolite-like metal-organic frameworks (ZMOFs): Design, synthesis, and properties, Chem. Soc. Rev., 2015, 44, 228–249 RSC.
- Y. Sun, C. Zhuo, F. Wang and J. Zhang, Synthesis of an interrupted AST–Type zeolitic imidazolate framework, Chin. J. Chem., 2020, 38, 449–452 CrossRef CAS.
- P. Brandao, A. J. Burke and M. Pineiro, A decade of indium-catalyzed multicomponent reactions (MCRs), Eur. J. Org. Chem., 2020, 5501–5513 CrossRef CAS.
- Z.-Z. Lin, F.-L. Jiang, L. Chen, C.-Y. Yue, D.-Q. Yuan, A.-J. Lan and M.-C. Hong, A highly symmetric porous framework with multi-intersecting open channels, Cryst. Growth Des., 2007, 7, 1712–1715 CrossRef CAS.
- Y. Liu, V. C. Kravtsov, D. A. Beauchamp, J. F. Eubank and M. Eddaoudi, 4-Connected metal–organic assemblies mediated via heterochelation and bridging of single metal Ions: Kagomé Lattice and the M6L12 Octahedron, J. Am. Chem. Soc., 2005, 127, 7266–7267 CrossRef CAS.
- J. A. Brant, Y. Liu, D. F. Sava, D. Beauchamp and M. Eddaoudi, Single-metal-ion-based molecular building blocks (MBBs) approach to the design and synthesis of metal–organic assemblies, J. Mol. Struct., 2006, 796, 160–164 CrossRef CAS.
- Y.-L. Liu, V. Kravtsov, R. Larsen and M. Eddaoudi, Molecular building blocks approach to the assembly of zeolite-like metal-organic frameworks (ZMOFs) with extra-large cavities, Chem. Commun., 2006, 1488–1490 RSC.
- Y.-X. Tan, Y.-P. He, M. Wang and J. Zhang, A water-stable zeolite-like metal-organic framework for selective separation of organic dyes, RSC Adv., 2014, 4, 1480–1483 RSC.
- Y. Yuan, J. Li, X. Sun, G. Li, Y. Liu, G. Verma and S. Ma, Indium–organic frameworks based on dual secondary building units featuring halogen-decorated channels for highly effective CO2 fixation, Chem. Mater., 2019, 31, 1084–1091 CrossRef CAS.
- S.-L. Hou, J. Dong, X.-L. Jiang, Z.-H. Jiao and B. Zhao, A noble-metal-free metal-organic framework (MOF) catalyst for the highly efficient conversion of CO2 with propargylic alcohols, Angew. Chem., Int. Ed., 2019, 58, 577–581 CrossRef CAS PubMed.
- S. E. Springer, J. J. Mihaly, N. Amirmokhtari, A. B. Crom, M. Zeller, J. I. Feldblyum and D. T. Genna, Framework isomerism in a series of btb-containing In-derived metal-organic frameworks, Cryst. Growth Des., 2019, 19, 3124–3129 CrossRef CAS.
- Y. Han, H. Zheng, K. Liu, H. Wang, H. Huang, L.-H. Xie, L. Wang and J.-R. Li,
In situ ligand formation-driven preparation of a heterometallic metal-organic framework for highly selective separation of light hydrocarbons and efficient mercury adsorption, ACS Appl. Mater. Interfaces, 2016, 8, 23331–23337 CrossRef CAS PubMed.
- Y. Liu, V. Kravtsov and M. Eddaoudi, Template-directed assembly of zeolite-like
metal-organic frameworks (ZMOFs): a usf-ZMOF with an unprecedented zeolite topology, Angew. Chem., Int. Ed., 2008, 47, 8446–8449 CrossRef CAS PubMed.
- S. Chen, J. Zhang, T. Wu, P. Feng and X. Bu, Multiroute synthesis of porous anionic frameworks and size-tunable extraframework organic cation-controlled gas sorption properties, J. Am. Chem. Soc., 2009, 131, 16027–16029 CrossRef CAS PubMed.
- S.-T. Zheng, J.-T. Bu, Y. Li, T. Wu, F. Zuo, P. Feng and X. Bu, Pore space partition and charge separation in cage-within-cage indium-organic frameworks with high CO2 uptake, J. Am. Chem. Soc., 2010, 132, 17062–17064 CrossRef CAS.
- S.-T. Zheng, F. Zuo, T. Wu, B. Irfanoglu, C. Chou, R. A. Nieto, P. Feng and X. Bu, Cooperative assembly of three-ring-based zeolite-type metal-organic frameworks and Johnson-type dodecahedra, Angew. Chem., Int. Ed., 2011, 50, 1849–1852 CrossRef CAS.
- S.-T. Zheng, C. Mao, T. Wu, S. Lee, P. Feng and X. Bu, Generalized synthesis of zeolite-type metal-organic frameworks encapsulating immobilized transition-metal clusters, J. Am. Chem. Soc., 2012, 134, 11936–11939 CrossRef CAS PubMed.
- J. Yu, Y. Cui, C. Wu, Y. Yang, Z. Wang, M. O'Keeffe, B. Chen and G. Qian, Second-order nonlinear optical activity induced by ordered dipolar chromophores confined in the pores of an anionic metal-organic framework, Angew. Chem., Int. Ed., 2012, 51, 10542–10545 CrossRef CAS PubMed.
- J. E. Farid Nouar, J. F. Eubank, P. Forster and M. Eddaoudi, Zeolite-like metal-organic frameworks (ZMOFs) as hydrogen storage platform: lithium and magnesium Ion-exchange and H2-(rho-ZMOF) interaction studies, J. Am. Chem. Soc., 2009, 131, 2864–2870 CrossRef PubMed.
- Q. Li, D.-X. Xue, Y.-F. Zhang, Z.-H. Zhang, Z. Gao and J. Bai, A dual-functional indium-organic framework towards organic pollutant decontamination via physically selective adsorption and chemical photodegradation, J. Mater. Chem. A, 2017, 5, 14182–14189 RSC.
- J. Zhang, S. Chen and X. Bu, Multiple functions of ionic liquids in the synthesis of three-dimensional low-connectivity homochiral and achiral frameworks, Angew. Chem., Int. Ed., 2008, 47, 5434–5437 CrossRef CAS PubMed.
- Y. L. Mohamed, H. Alkordi, R. W. Larsen, J. F. Eubank and M. Eddaoudi, Zeolite-like metal-organic frameworks as platforms for applications: On metalloporphyrin-based catalysts, J. Am. Chem. Soc., 2008, 130, 12639–12641 CrossRef.
- J. Zhang, S. Chen, T. Wu, P. Feng and X. Bu, Homochiral crystallization of microporous framework materials from achiral precursors by chiral catalysis, J. Am. Chem. Soc., 2008, 130, 12882–12883 CrossRef CAS PubMed.
- X. Chen, H. Jiang, X. Li, B. Hou, W. Gong, X. Wu, X. Han, F. Zheng, Y. Liu, J. Jiang and Y. Cui, Chiral phosphoric acids in metal-organic frameworks with enhanced acidity and tunable catalytic selectivity, Angew. Chem., Int. Ed., 2019, 58, 14748–14757 CrossRef CAS.
- Z. Deng, C. Fang, X. Ma, X. Li, Y.-J. Zeng and X. Peng, One stone two birds: Zr-Fc metal-organic framework nanosheet for synergistic photothermal and chemodynamic cancer therapy, ACS Appl. Mater. Interfaces, 2020, 12, 20321–20330 CrossRef CAS PubMed.
- Z. Deng, H. Yu, L. Wang, J. Liu and K. J. Shea, Ferrocene-based metal-organic framework nanosheets loaded with palladium as a super-high active hydrogenation catalyst, J. Mater. Chem. A, 2019, 7, 15975–15980 RSC.
- X.-D. Du, W. Zheng, X.-H. Yi, J.-P. Zhao, P. Wang and C.-C. Wang, General strategy for lanthanide coordination polymers constructed from 1,1′-ferrocenedicarboxylic acid under hydrothermal conditions, CrystEngComm, 2018, 20, 2608–2616 RSC.
- R. Rajak, M. Saraf, A. Mohammad and S. M. Mobin, Design and construction of a ferrocene based inclined polycatenated Co-MOF for supercapacitor and dye adsorption applications, J. Mater. Chem. A, 2017, 5, 17998–18011 RSC.
- K. Hirai, H. Uehara, S. Kitagawa and S. Furukawa, Redox reaction in two-dimensional porous coordination polymers based on ferrocenedicarboxylates, Dalton Trans., 2012, 41, 3924–3927 RSC.
- J. Huo, L. Wang, E. Irran, H. Yu, J. Gao, D. Fan, B. Li, J. Wang, W. Ding, A. M. Amin, C. Li and L. Ma, Hollow ferrocenyl coordination polymer microspheres with micropores in shells prepared by Ostwald Ripening, Angew. Chem., Int. Ed., 2010, 49, 9237–9241 CrossRef CAS PubMed.
- X.-R. Meng, G. Li, H.-W. Hou, H.-Y. Han, Y.-T. Fan, Y. Zhu and C.-X. Du, A series of novel metal-ferrocenedicarboxylate coordination polymers: crystal structures, magnetic and luminescence properties, J. Organomet. Chem., 2003, 679, 153–161 CrossRef CAS.
- D. Guo, Y.-T. Li, C.-Y. Duan, H. Mo and Q.-J. Meng, Heterodimetallic compounds assembled from ferrocenedicarboxylato and ferrocenecarboxylato ligands, Inorg. Chem., 2003, 42, 2519–2530 CrossRef.
- G. Li, H.-W. Hou, L.-K. Li, X.-R. Meng, Y.-T. Fan and Y. Zhu, Novel Pb(II), Zn(II), and Cd(II) coordination polymers constructed from ferrocenyl-substituted carboxylate and bipyridine-based ligands, Inorg. Chem., 2003, 42, 4995–5004 CrossRef CAS.
- J. Benecke, E. S. Grape, A. Fuss, S. Wohlbrandt, T. A. Engesser, A. K. Inge, N. Stock and H. Reinsch, Polymorphous Indium metal-organic frameworks based on a ferrocene linker: redox activity, porosity, and structural diversity, Inorg. Chem., 2020, 59, 9969–9978 CrossRef CAS PubMed.
- R. Gao, S.-M. Chen, F. Wang and J. Zhang, Single-crystal syntheses and properties of indium-organic frameworks based on 1,1′-Ferrocenedicarboxylic Acid, Inorg. Chem., 2021, 60, 239–245 CrossRef CAS PubMed.
- Z. Huang, H. Yu, L. Wang, X. Liu, T. Lin, F. Haq, S. Z. Vatsadze and D. A. Lemenovskiy, Ferrocene-contained metal organic frameworks: From synthesis to applications, Coord. Chem. Rev., 2021, 430, 213737 CrossRef CAS.
- J. Benecke, S. Mangelsen, T. A. Engesser, T. Weyrich, J. Junge, N. Stock and H. Reinsch, A porous and redox active ferrocenedicarboxylic acid based aluminium MOF with a MIL-53 architecture, Dalton Trans., 2019, 48, 16737–16743 RSC.
- G. M. Sheldrick, Crystal structure refinement with SHELXL, Acta Crystallogr., Sect. C: Struct. Chem., 2015, 71, 3–8 Search PubMed.
- D.-J. Li, Q.-H. Li, Z.-R. Wang, Z.-Z. Ma, Z.-G. Gu and J. Zhang, Interpenetrated metal-porphyrinic framework for enhanced nonlinear optical limiting, J. Am. Chem. Soc., 2021, 143, 17162–17169 CrossRef CAS.
- V. A. Blatov, A. P. Shevchenko and D. M. Proserpio, Applied topological analysis of crystal structures with the program package topospro, Cryst. Growth Des., 2014, 14, 3576–3586 CrossRef CAS.
- N. J. Long, Organometallic Compounds for nonlinear optics-the search for en-light-enment!, Angew. Chem., Int. Ed. Engl., 1995, 34, 21–38 CrossRef CAS.
- K. Senthilkumar, K. Thirumoorthy, G. Vinitha, K. Soni, N. S. P. Bhuvanesh and N. Palanisami, Synthesis and characterization of d10 metal complexes of 3-Me-5-FcPz: Structural, theoretical and third order nonlinear optical properties, J. Mol. Struct., 2017, 1128, 36–43 CrossRef CAS.
- C. Gao, J. Zhou, M. Cui, D. Chen, L. Zhou, F. Li and X.-L. Li, Distinct nonlinear optical responses in three pairs of 2D homochiral Ag(i) enantiomers modulated by dicarboxylic acid ligands, Inorg. Chem. Front., 2022, 9, 284–293 RSC.
- X.-L. Li, A. Wang, M. Cui, C. Gao, X. Yu, B. Su, L. Zhou, C.-M. Liu, H.-P. Xiao and Y.-Q. Zhang, Modulating two pairs of chiral Dy(III) enantiomers by distinct beta-diketone ligands to show giant differences in single-ion magnet performance and nonlinear optical response, Inorg. Chem., 2022, 61, 9283–9294 CrossRef CAS PubMed.
- M. Cui, L. Yang, F. Li, L. Zhou, Y. Song, S.-M. Fang, C.-M. Liu and X.-L. Li, Multifunctional Dy(III) enantiomeric pairs showing enhanced photoluminescences and third-harmonic generation responses through the coordination role of homochiral tridentate N,N,N-pincer ligands, Inorg. Chem., 2021, 60, 13366–13375 CrossRef CAS PubMed.
- G. Li, Y. Song, H. Hou, L. Li, Y. Fan, Y. Zhu, X. Meng and L. Mi, Synthesis, Crystal structures, and third-order nonlinear optical properties of a series of ferrocenyl organometallics, Inorg. Chem., 2003, 42, 913–920 CrossRef CAS PubMed.
- M. Sheik-Bahae, A. A. Said, T.-H. Wei, D. J. Hagan and E. W. Van-Stryland, Sensitive measurement of optical nonlinearities using a single beam, IEEE J. Quantum Electron., 1990, 26, 760–769 CrossRef CAS.
- X. Liu, M. Kozlowska, T. Okkali, D. Wagner, T. Higashino, G. Brenner-Weiss, S. M. Marschner, Z. Fu, Q. Zhang, H. Imahori, S. Brase, W. Wenzel, C. Woll and L. Heinke, Photoconductivity in metal-organic framework (MOF) thin films, Angew. Chem., Int. Ed., 2019, 58, 9590–9595 CrossRef CAS PubMed.
- C. Wang, S. Wang, F. Kong and N. Chen, Ferrocene-sensitized titanium-oxo clusters with effective visible light absorption and excellent photoelectrochemical activity, Inorg. Chem. Front., 2022, 9, 959–967 RSC.
- Q.-F. Chen, X. Zhao, Q. Liu, J.-D. Jia, X.-T. Qiu, Y.-L. Song, D.-J. Young, W.-H. Zhang and J.-P. Lang, Tungsten(VI)-copper(I)-sulfur cluster-supported metal-organic frameworks bridged by in situ click-formed tetrazolate ligands, Inorg. Chem., 2017, 56, 5669–5679 CrossRef CAS PubMed.
- J. Zhang, Q. Xiang, Y. Zhu, J. Yang, Y. Song and C. Zhang, Two W/S/Cu-cluster-containing metal–organic frameworks fabricated by multidentate organic ligands: New topologies, strong NLO properties, and efficient luminescent detection, Cryst. Growth Des., 2021, 21, 3225–3233 CrossRef CAS.
Footnote |
† Electronic supplementary information (ESI) available: Additional experimental details, crystallographic studies, additional figures, general characterization, fluorescence spectra, photocurrent, cyclic voltammetric, and adsorption characterization. CCDC 2204897–2204903. For ESI and crystallographic data in CIF or other electronic format see DOI: https://doi.org/10.1039/d2qi01949c |
|
This journal is © the Partner Organisations 2023 |