DOI:
10.1039/D3OB00792H
(Review Article)
Org. Biomol. Chem., 2023,
21, 5317-5346
Synthesis, reactions and application of chalcones: a systematic review
Received
21st May 2023
, Accepted 7th June 2023
First published on 8th June 2023
Abstract
Chalcones are a group of naturally occurring compounds that have biological effects that include anti-inflammatory, anti-cancer, and antibacterial properties. Current chalcone research, including their synthesis, structure–activity relationships, and biological activities, is summarized herein. Along with their toxicity and safety profiles, the prospective usage of chalcones in medicinal research and development is discussed. This review emphasizes the need for additional research in order to fully examine the therapeutic potential of chalcones as therapeutic agents for the treatment of a variety of disorders.
1. Introduction
Due to the fact that living organisms produce a vast range of bioactive substances, nature has served as an inspiration for scientists throughout time for the development of new medicinal agents. Also, for the disciplines of pharmaceutical and drug discovery studies, heterocycles are crucial pharmacophores.1–3 Ancient Egyptians, Chinese, and Mesopotamians recorded a variety of plant-based medicines that are still in use today.4,5 Due to several inherent challenges with natural product drug development, the pharmaceutical industry has changed its focus from natural products to synthetic compound libraries and high-throughput screening (HTS) for the purpose of finding pharmacological leads.6–8 The (E)-1,3-diphenyl-2-propene-1-ones, known as chalcones, are a significant chemotype of polyphenolic chemical substances. Since these substances are part of the plentiful class of natural substances known as flavonoids and isoflavonoids found in fruits, vegetables, teas, and soy products, researchers have been researching them for decades.9,10 The chalcone scaffold is made up of two phenyl rings joined by a propenone bridge, an unsaturated carbonyl system with three carbons. Fig. 1 illustrates how aryl rings A and B are distinguished by their respective prime and nonprime numbers. Chalcones come in two different forms: trans (E, 1) and cis (Z, 2) isomers. The most common chalcones are E isomers because they are generally more thermodynamically stable, whereas Z isomers are unstable because of thier steric interactions between the carbonyl group and ring B.11–13
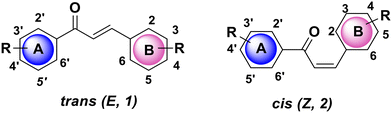 |
| Fig. 1 Structural and numerical representations of chalcone. | |
In higher plants, an enzyme called chalcone synthase converts p-coumaroyl-CoA and malonyl-CoA into chalcones. L-Phenylalanine is changed into p-coumaroyl-CoA via the phenylpropanoid pathway, which produces the chalcone aromatic B-ring and 3C bridge. Chalcone is metabolized through a variety of pathways in plants, leading to the production of aurones, glycosyl conjugates, and naringenin. Chalcones play a crucial ecological role as signaling molecules in plant–microbe symbioses and as biochemical regulators of plant dispersal. Glycosyl conjugated chalcones, which are also common in flowers, are essential for pollination.14–16 The chalcone family has garnered a lot of attention because of its wide spectrum of biological activity, as well as from synthesis and biosynthesis perspectives. For thousands of years, plants and herbs have been used to create chalcones as remedies for a variety of illnesses, such as cancer, diabetes, and inflammation.17–19 Chalcone chemicals are classed based on their molecular structures. The most prevalent substances found in plants are those that have been hydroxylated or methoxylated (see Fig. 2).20 Several hydroxy chalcones have medical and pharmaceutical uses based on anti-cancer,21,22 antioxidant,19 anti-inflammatory,23,24 antidiabetic,25 anti-microbial, and neuroprotective properties.26–28 Particularly, the outcomes of clinical trials for the treatment of trunk or branch varicosity and chronic venous lymphatic insufficiency with hesperidin methylchalcone and hesperidin trimethylchalcone (displayed in Fig. 3), respectively, were encouraging.
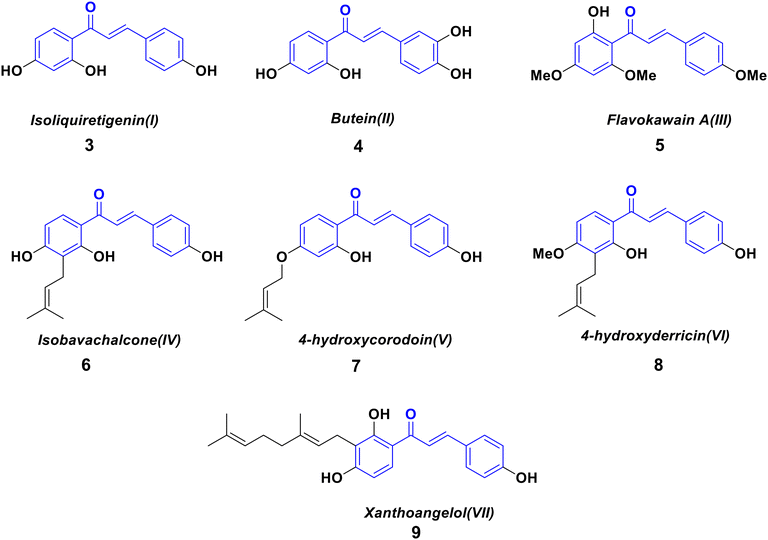 |
| Fig. 2 Analogs of chalcones which have biological applications. | |
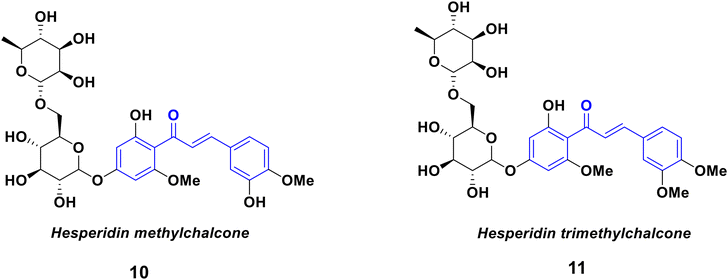 |
| Fig. 3 Chemical structures of naturally occurring chalcones that have been approved for clinical use. | |
2. Fluorescent characteristics of chalcones
When a molecule is excited to a higher state, which causes it to produce electromagnetic radiation spontaneously within a few nanoseconds, it exhibits the photoluminescence phenomenon known as fluorescence.29 Fluorophores, also known as fluorescent dyes, are compounds with fluorescence qualities. These molecules have structural characteristics that contribute to the fluorescence mechanism.30 Quantum yield (ΦF) is used to describe the efficiency of fluorescence and is defined as the ratio of photons emitted to photons absorbed. If the number of photons absorbed equals the number of photons emitted, the fluorescence quantum yield is 1.0 (100%) and achieves the maximum (ΦF) value. Also, excited 2′-hydroxychalcones have a wider range of reactivity than other chalcone derivatives, which exclusively show cis/trans-isomerization and dimerization.31–33 The existence of the hydroxyl group at position 2′ in the chalcone leads to photoinduced cyclization to generate flavanonone11,34 and dark acid–base.35 In addition, phototautomers are produced as a result of intramolecular proton transfer (ESIPT) in the excited state assisted by hydrogen bonding between carbonyl and hydroxyl groups.36,37 The fluorescence and nonlinear optical properties of chalcone and its derivatives, resulting from the π-conjugated planar structure, have gained considerable attention because of the delocalization of the electronic charge distribution and overlapping π orbitals.38 Chalcone and its derivatives are traditional luminogenic materials that emit strong light in the solution state, but lose this luminescence in high-concentration solutions or solids.39 Nevertheless, by introducing electron donors or acceptors into aromatic rings, their optical properties can be altered. As chalcones are very significant and may be modified in structure, it is feasible to develop and manufacture chalcones that emit intense fluorescence in the solid state making them potential chemical probes for mechanistic investigations and imaging/diagnosis. The chalcone in chalcone-based compounds acts as an optically active moiety as well as a recognition unit for selectively detecting the target analytes where their photophysical properties are disturbed after interacting with the analytes, making them suitable for analytical use as selective chemosensors. Several methods, including photoinduced electron transfer (PET), intermolecular charge transfer (ICT), chelation-enhanced fluorescence (CHEF), and aggregation-induced emission (AIE), have been employed to design chalcone-based chemosensors.40,41 A variety of chalcones (12–17) with appropriate substituents (electron-withdrawing functional groups) on both aryl rings have been reported to display intrinsic fluorescence properties,42,43 as indicated in Fig. 4. Prior experimental studies have demonstrated that the chalcone needs specific structural features in order to qualify as a suitable fluorescent material. A structure–fluorescence relationship (SFR) investigation revealed the following: (1) the molecular planarity played an important role in fluorescence; (2) for the A ring, weak electron-donating groups, like a methoxyl group, showed good quantum yields, as opposed to electron-withdrawing substituents, which resulted in a decreased quantum yield; (3) for ring B, properties like a higher extinction coefficient, greater quantum yields, and a lower ionization potential were highly dependent on the presence of disubstituted amino groups; and (4) extension of the conjugation of an α,β-unsaturated system would lead to a decrease in fluorescence and cause a redshift of the maximum emission.14,17,44 Fluorescent chalcones have been studied for their potential activity in the imaging of stem cells and the detection of various diseases.45,46
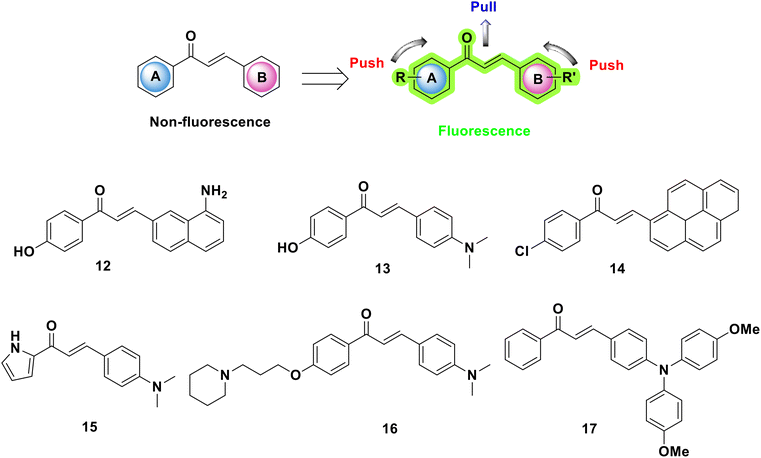 |
| Fig. 4 Chalcones with fluorescent properties. | |
3. Synthesis of chalcones
Several methods are used to synthesize different derivatives of chalcones. Typically, condensation procedures utilizing acid or base catalysis can be used to produce them. The main routes for the synthesis of chalcones include Claisen–Schmidt condensation, the carbonylative Heck coupling reaction, coupling reactions, Sonogashira isomerization coupling, Meyer–Schuster rearrangement, Suzuki–Miyaura coupling reactions, Suzuki coupling reactions, Stille coupling, one-pot synthesis, and solid acid catalyst mediated reactions, as shown in Scheme 1.12,47,48
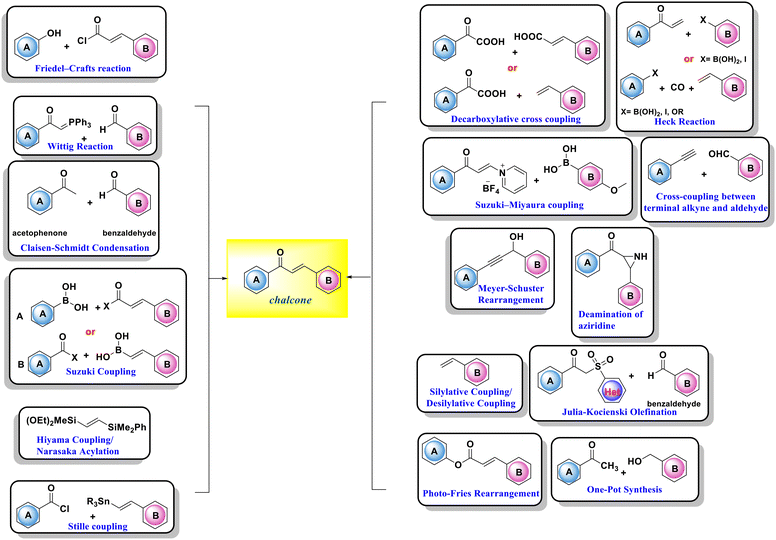 |
| Scheme 1 Common reactions for synthesizing chalcone scaffolds. | |
3.1. Claisen–Schmidt condensation
Claisen–Schmidt condensation is one of the most popular synthesis techniques. In this procedure, chalcones are synthezized through a straightforward condensation reaction between benzaldehyde and acetophenone derivatives in the presence of acid or base catalysts in polar solvents at 50–100 °C for several hours, as indicated in Schemes 2 and 3.26,47,49–51 This reaction is most frequently used because it is straightforward and highly effective at forming the carbon–carbon double bond with little restriction on the complexity of the molecules, despite the fact that the main drawback is the slow reaction rate, which typically takes a few days to complete and also produces a complex mixture containing the desired product, by-products, and sometimes starting materials as well. The synthesis of chalcones is mostly predicated on base condition.17,52
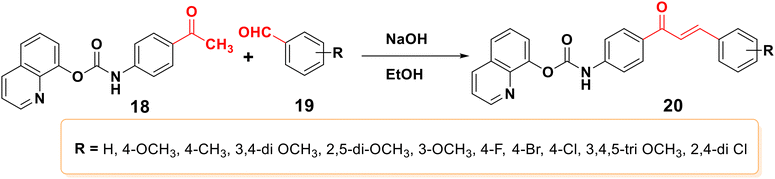 |
| Scheme 2 Synthesis of chalcones using a base as a catalyst. | |
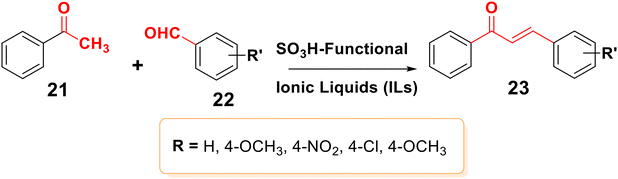 |
| Scheme 3 Synthesis of chalcones using an acid as a catalyst. | |
Moreover, the synthesis of 2′-hydroxychalcones 26 was reported using Claisen–Schmidt condensation,22,53,54 as shown in Scheme 4. Also, boron trifluoride etherate can be used as a high-efficiency catalyst to synthesize distyryl ketones and chalcones bearing phenolic hydroxyl groups in high yield by removing water produced during the reactions with the aid of desiccants such as CaCl2, leading to the synthesis of 1,5-bis(4-hydroxyphenyl) penta-1,4-dien-3-one 29,55 as shown in Scheme 5.
 |
| Scheme 4 Synthesis of 2′-hydroxychalcones. | |
 |
| Scheme 5 Synthesis of distyryl ketone. | |
3.2. Photo-Fries rearrangement of phenyl cinnamates
Since the early 1970s, the photochemistry of chalcones has become more important since 2′-hydroxychalcones are important intermediates in the biosynthesis of flavonoids.56 Hydroxychalcones can be produced via photo-Fries rearrangement, which is an ortho–para selective reaction of phenyl cinnamate 30 to hydroxy aryl ketone 31 in the presence of Lewis acids, as shown in Scheme 6. The relative ratio of products is greatly influenced by the reaction conditions (temperature, concentration, solvent type, and substrates).57–60 For chalcone synthesis, photo-Fries rearrangement is not commonly used due to its low yield, prolonged reaction time, and complex procedure.
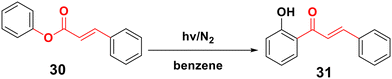 |
| Scheme 6 Synthesis of 2′-hydroxychalcones via photochemistry. | |
3.3. Sequential silylative coupling/desilylative coupling in the synthesis of chalcones
For the synthesis of (E)-α,β-unsaturated ketones 36 from styrenes 32, a very effective stereo-selective one-pot technique based on sequential ruthenium-catalyzed silylative coupling followed by rhodium-catalyzed desilylative acylation processes was reported,61–63 as displayed in Scheme 7. Under ideal conditions, substituted styrenes 32 bearing both electron donating and electron withdrawing functional groups produced (E)-styryl ketones 36 in high yields through (E)-styrylsilane intermediates 34, regardless of the electronic characteristics and position of the substituent on the aromatic ring.64 A variety of anhydrides can be used to perform this process, including those of aliphatic, aromatic, and unsaturated carboxylic acids.
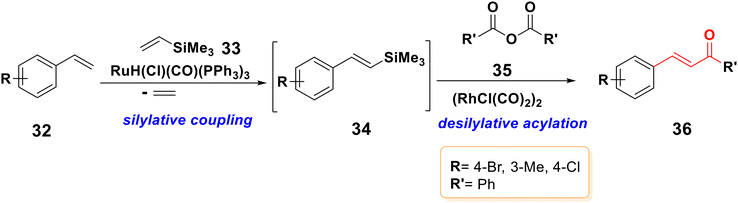 |
| Scheme 7 Synthesis of (E)-α,β-unsaturated ketones from styrenes. | |
3.4. The Heck reaction
By combining unsaturated ketone 38 with an arylboronic acid or aryl iodide 37 in the presence of a palladium catalyst and a base, the typical Heck reaction can be used to create the stilbene chalcone 40,26,65,66 as indicated in Scheme 8. Additionally, carbon monoxide is present to create chalcone 40, and palladium (Pd) serves as a catalyst in the carbonylative vinylation of phenyl halide 37 with styrene 39.67,68 The synthesis of substituted chalcones 43 from aryl triflates 41 with aromatic olefins 42 using the carbonylative Heck reaction, as shown in Scheme 9, was also reported.69
 |
| Scheme 8 Heck coupling and the carbonylative Heck coupling reaction. | |
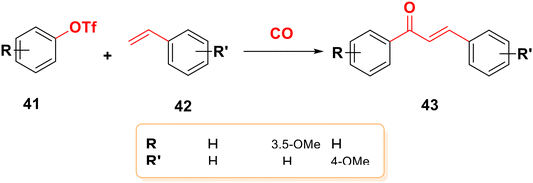 |
| Scheme 9 Synthesis of chalcones via the carbonylative Heck reaction. | |
3.5. Suzuki coupling
Suzuki coupling was used for the first time in 2003 for the synthesis of chalcones by employing carbon–carbon bond formation that was stimulated by palladium.70,71 Two possible methods for the synthesis of chalcones 40 are based on retrosynthetic analysis: coupling benzoyl chloride 44 with phenylvinylboronic acid 45 and coupling cinnamoyl chloride 47 with phenylboronic acid 48, as shown in Scheme 10.
 |
| Scheme 10 Synthesis of chalcone via a Suzuki coupling reaction. | |
Additionally, a highly effective aqueous system of the PdCl2-catalyzed cross-coupling reaction of arylboronic acid 45 with carboxylic anhydride 48 yielding aryl ketone (Scheme 11) without the use of phosphine ligands in short reaction times, unaffected by electron-releasing and electron-withdrawing substituents, was reported.72
 |
| Scheme 11 Synthesis of chalcone via cross-coupling of benzoic anhydride and phenylboronic acid. | |
3.6. Suzuki–Miyaura coupling
Organoborane 50 is cross-coupled with N-vinylpyridinium tetrafluoroborate salts 49 under palladium catalysis producing products in yields between 60 and 81%73 (Scheme 12).
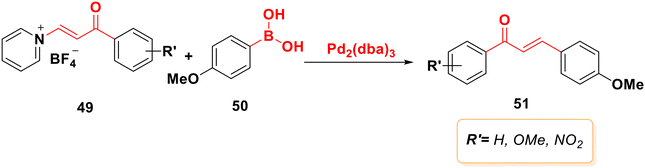 |
| Scheme 12 Synthesis of chalcones via Suzuki–Miyaura coupling. | |
3.7. Julia–Kocienski olefination
When benzaldehyde 53 and heteroaryl-sulfonyl phenylethanone 52 combine at −78 °C, the resulting main diastereomer is (E)-chalcone,74 as shown in Scheme 13, where the Z/E ratio for all the products is >1
:
99%, as determined by 1H NMR/HPLC analysis. The Julia reagent with benzothiazole as the heteroaryl was most efficient, whereas bases like 1,8-diazabicyclo[5.4.0]undec-7-ene (DBU) and nonpolar solvents showed higher yields than those of reactions in polar solvents. However, at −78 °C, the reaction yield significantly decreased.
 |
| Scheme 13 Synthesis of chalcone via the Julia–Kocienski olefination reaction. | |
3.8. Wittig reaction
Triphenylbenzoylmethylene phosphorane 54 interacts with benzaldehyde 55 in a standard heating process by refluxing it in benzene for three days or in trichlorohydrin for thirty hours, to give the product in 70% yield75 (Scheme 14). The reaction rate was accelerated by microwave irradiation, yielding >80% of the product in 5–8 minutes.76
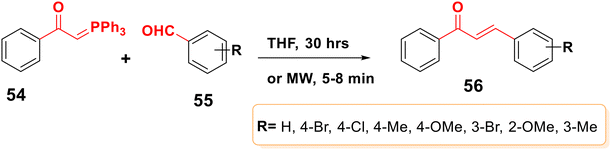 |
| Scheme 14 Synthesis of chalcones via the Wittig reaction. | |
3.9. Sequential Hiyama coupling/Narasaka acylation reaction
The (E)-1,2-bis-silylethene derivative 57 was reacted with aryl halides 58 in the presence of an ionic gel stabilized Pd catalyst and a catalytic quantity of an ammonium fluoride-supported polymer. The resulting mixture subsequently underwent the Narasaka reaction using a rhodium catalyst to produce chalcone 60,77,78 as shown in Scheme 15.
 |
| Scheme 15 Synthesis of 2′-hydroxychalchone via the Hiyama coupling/Narasaka acylation reaction. | |
3.10. Stille coupling reactions
The first example of the C–C bond coupling reaction to produce chalcone was described by Stille in 1983. Pd was used as a catalyst for the reaction between organotin compounds (organostannanes) 61 and alkyl/aryl halide 44,79 as shown in Scheme 16. According to Yamakawa et al., Stille couplings between the tribenzylstryltin derivative and benzoyl chloride in THF as a solvent produced 91% yield of chalcone.80
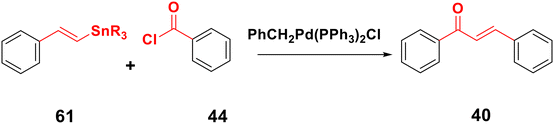 |
| Scheme 16 Synthesis of chalcone via the Stille coupling reaction. | |
Moreover, the selective formation of Z sulfenyl chalcones 64 was reported by using Stille cross-coupling with the Z α-sulfenyl-β-chloroenones 62, while the E α-sulfenyl-β-chloroenones did not react under the same conditions (Scheme 17).81,82
 |
| Scheme 17 Synthesis of chalcones from α-sulfenyl-β-chloroenone. | |
3.11. Decarboxylative cross-coupling
Jiang et al. described the synthesis of different chalcones 67 by the decarboxylation cross-coupling reaction of α-oxo carboxylic acid 65 and cinnamic acid derivatives 66 in an aqueous solution using an iron-facilitated oxidative radical,83 as shown in Scheme 18. Also, Wu et al. claimed that chalcones 70 could be approached via decarboxylative cross-coupling reactions between α-keto acids 68 and alkenes 69 using a silver-catalyzed decarboxylation process,84–86 as shown in Scheme 19.
 |
| Scheme 18 Synthesis of various chalcones using an iron-catalyzed decarboxylative cross-coupling reaction. | |
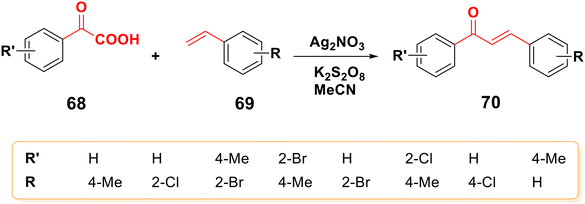 |
| Scheme 19 Synthesis of various chalcones using a silver-catalyzed decarboxylative cross-coupling reaction. | |
3.12. Deamination of aziridines
According to Lee et al., aziridine deamination was accomplished using N2O4 in the presence of triethylamine as a base and THF as a solvent in 10 minutes at −23 °C in an inert environment87 (Scheme 20). Many new deaminating agents have been created as a result of the difficulties associated with that process, including iodine and diethylthiourea,88 Ph3P/I289 and NBS-ceric ammonium nitrate90 to produce chalcones in high yield.
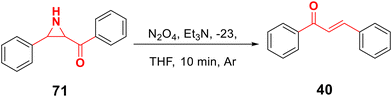 |
| Scheme 20 Synthesis of chalcone via aziridine deamination. | |
3.13. Meyer–Schuster rearrangement
Through a formal 1,3-hydroxyl shift and tautomerization in a Meyer–Schuster rearrangement, propargyl alcohols are transformed into chalcone. For the production of chalcones, scientific researchers have discovered an effective gold-catalyzed process91–93 (Scheme 21). A lower yield was observed in the absence of additives in the case of secondary and tertiary propargylic alcohols, where the reaction progress was particularly effective. Moreover, a number of Lewis acids and transition metal catalysts, such as silver salt/CO2, p-toluene sulfonic acid in dichloroethane, AuCl3, and FeCl3 in THF, have been applied.27,77,94–96
 |
| Scheme 21 Synthesis of chalcone via the Meyer–Schuster rearrangement. | |
3.14. Cross-coupling between terminal alkynes and aldehydes
A cross coupling reaction between terminal alkynes and aryl aldehydes was also reported to be involved in the synthesis of chalcones and their derivatives. Moreover, it was reported that the coupling between terminal alkyne 73 and aldehyde 74 could be accomplished using a Lewis acid (SnCl2) or copper salts (CuSO4) (Scheme 22).97–99 A rhodium-based catalyst system in the presence of 1,1′-bis(diphenylphosphino)ferrocene (dppf) as ligand was reported in the synthesis of 2′-hydroxychalcone 31,5,100 as seen in Scheme 23.
 |
| Scheme 22 Cross coupling reaction of aryl aldehyde with a terminal alkyne. | |
 |
| Scheme 23 Synthesis of 2′-hydroxychalcone. | |
3.15. Friedel–Crafts acylation with cinnamoyl chloride
Chalcone 80 can be made from phenol 79 and cinnamoyl chloride 47 using a strong Lewis acid catalyst, such as aluminium trichloride. In this process, the B-ring and three-carbon bridge are provided by the acylating agent, while the A-ring is constructed using phenol,17,47 as elucidated in Scheme 24.
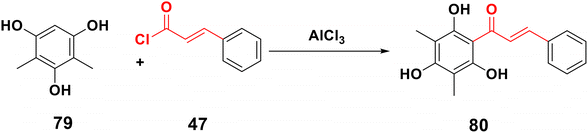 |
| Scheme 24 Synthesis of chalcone from cinnamoyl chloride. | |
3.16. One-pot synthesis of chalcones
By skipping intermediary purification and increasing yields, one-pot synthesis is a technique for increasing reaction efficiency and conserving time. Using CrO3 as an oxidizing agent, chalcone 83 can be produced from alcohol 81 and ketone 82 in a single pot,12 as shown in Scheme 25.
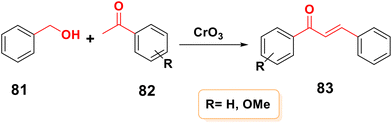 |
| Scheme 25 Synthesis of chalcones via one-pot synthesis. | |
4. Reactions of chalcone derivatives
4.1. Oxidative cyclization of 2′-hydroxychalcones to aurones and flavones
It is well known that Hg2+, Cu2+ and Tl3+ mediated oxidative cyclizations of 2′-hydroxychalcone 31 produce aurone 84,101 while I2, Se4+, In3+, Cu+ and Fe3+ mediated reactions produce flavone 85.102 Moreover, a diphenyl disulfide (PhSSPh)-mediated reaction involving peroxide and oxalic acid was used to convert 2′-hydroxychalcone into flavone 85,102 as displayed in Scheme 26.
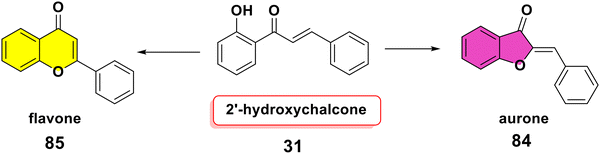 |
| Scheme 26 Oxidation cyclization products from 2′-hydroxychalcone. | |
4.2. Michael acceptor-related chemical reactions
Electrophilic Michael acceptors are essential research tools in chemical biology because thier corrdination with different cellular signaling pathways. It is known that the α,β-unsaturated carbonyl group present in the scaffold of chalcones may function as a Michael acceptor. Chalcones can react with nucleophiles, such as proteins, enzymes, and DNA, in this way act as Michael acceptors17 (Scheme 27).
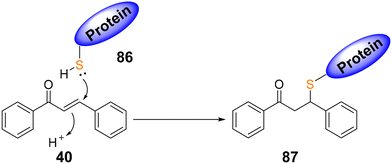 |
| Scheme 27 Michael addition of a chalcone with cysteine. | |
4.2.1. Michael addition.
Michael addition, a nucleophilic addition of β-carbon, is one of the most efficient methods in organic synthesis for producing C–C and C–X (X = O, N, or S) bonds (1,4-addition reaction). It is noted to be an important contender for producing an enantioselective Michael adduct in organocatalytic asymmetric synthesis103–105 (Scheme 28).
 |
| Scheme 28 Reactions of Michael-type addition to chalcone. | |
4.2.1.1. Michael addition of alkanes.
Effective asymmetric conjugate addition of organozinc reagents 89 to chalcones 88 were achieved in the presence of a wide variety of regioisomeric chiral diols 90 and 92 with phosphorus moieties as ligands106 (Scheme 29). This reaction in the presence of Cu(OAc)2 produced the main enantiomer 91 with an enantiomeric excess (ee) of 41%, whereas using Cu(acac)2 produced the product 93 with an ee of 90%.
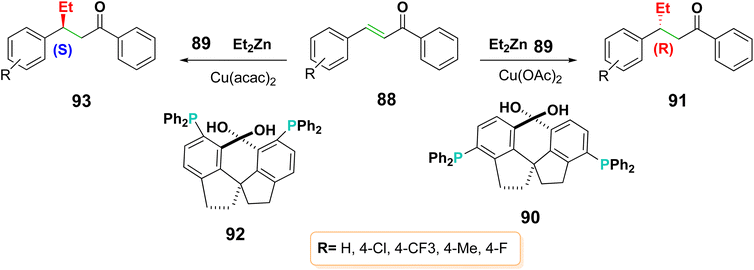 |
| Scheme 29 Asymmetric conjugate addition reaction of chalcones. | |
4.2.1.2. Michael addition of malonitriles or malonates.
It was claimed that an efficient, economical, and environmentally friendly Michael addition of malononitrile 94 to chalcone 31 could be accomplished using baker's yeast, a widely available and reasonably priced natural catalyst to form chalcone malonate 95. In ethanol-based nonaqueous media, yeast whole cells behaved admirably without experiencing a decrease in catalytic activity107 (Scheme 30). Moreover, it was reported that chalcone malonate could be formed with adding malonates to chalcone in the presence of potassium hydroxide.108 Also, there have been reports of the enantioselective Michael addition of diethyl malonate to chalcone utilizing a variety of cinchona-based alkaloids.103,109,110
 |
| Scheme 30 Michael addition of malononitrile to chalcone. | |
4.2.1.3. Michael addition of nitroalkane.
Using DBU as a superior base source, Cardona et al. reported the Michael addition reaction of nitromethane to chalcone.111 Moreover, Chanawanno et al. reported on the interaction of nitromethane with chalcone when Et3N was present as a base.112 Per-6-amino-β-cyclodextrin (per-6-ABCD) was reported to catalyze the enantioselective Michael addition reaction of nitromethane 97 to trans-chalcone 96 in water at room temperature with good yield from nitroketone compound 98 and an enantiomeric excess of 22.4–87.0%,113 as displayed in Scheme 31.
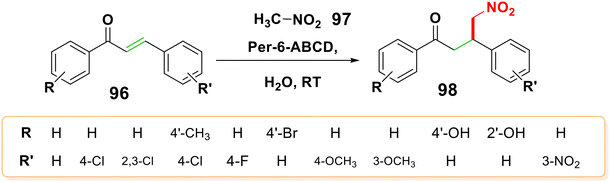 |
| Scheme 31 Michael addition reaction of nitromethane to trans-chalcone. | |
4.2.1.4. Michael addition of ketones.
Unmodified ketones and chalcones undergo highly enantioselective and organocatalytic Michael addition reactions.114 It was reported that derivatives of hydroxyphthalimide linked triazole–pyrrolidines 102 and 1,8-naphthalimides based on pyrrolidines 101 could catalyze the addition of ketones to chalcones to form compound 103 with enantiomeric excesses (ee) of 47–91% and 96%; respectively,115,116 as shown in Scheme 32.
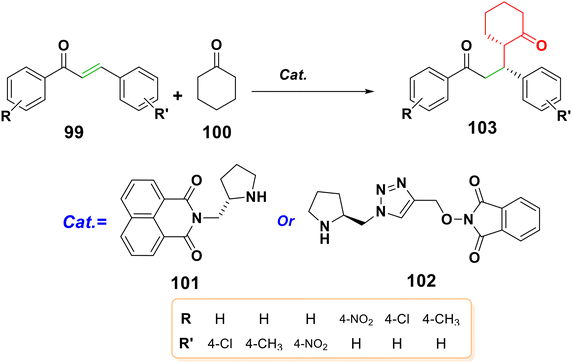 |
| Scheme 32 Structures of pyrrolidine-based 1,8-naphthalimide and hydroxyphthalimide linked triazole–pyrrolidine catalysts. | |
4.2.1.5. Sulfa-Michael addition to chalcones.
In Scheme 33, it is shown that enantioselective sulfa-Michael addition forms carbon–sulfur (C–S) bonds through stimulation reaction of chalcones with cysteines in biological systems.117 The corresponding adducts 104 were produced in up to >95% ee with the Michael addition of thiophenols to chalcones catalyzed via (+)-cinchonine, followed by crystallization.118 Additionally, it was reported that sulfonic acids 105 generated utilizing an appropriate aminothiourea bifunctional catalyst with excellent enantioselectivity (84–99%).119
 |
| Scheme 33 Sulfa Michael addition reactions of chalcone. | |
A method for the conjugate addition of sodium p-toluenesulfinate 106 to enones was disclosed that used TMSCl as an additive and FeCl3 as a catalyst for the β-sulfonation of chalcone compounds.120 In addition, a number of β-mercapto carbonyl compound 109 were produced when methyl thioglycolate 108 was added to chalcone 31 in the presence of a catalytic amount of iodine121 (Scheme 34).
 |
| Scheme 34 Sulfonation reactions of 2′-hydroxychalcone. | |
4.2.1.6. Aza-Michael reactions of chalcones.
The aza-Michael reaction is one of the most important synthesis routes for forming a C–N bond between an accessible NH-nucleophile and an unsaturated electrophile to generate useful intermediates for the synthesis of natural product analogues and active pharmaceutical ingredients.122 The synthesis of biologically active azaheterocycles such pyrroles, isoxazoles, pyrazoles, benzodiazepines, triazoles, and pyrimidines is frequently carried out using chalcones123–126 (Scheme 35).
 |
| Scheme 35 Aza-Michael addition reactions of chalcone with different reagents. | |
4.2.1.6.1. Aza-Michael addition of amines.
It is important that chiral auxiliaries and amino alcohols can all be synthesized from β-amino carbonyl derivatives, which are useful intermediates for a variety of organic compounds. They also form the backbone of numerous bioactive compounds and naturally occurring molecules. Moreover, the pharmaceutical and fine chemical industries use this sort of molecule. The synthesis of β-amino carbonyl derivatives has remained a primary objective for years due to the extensive range of uses. The addition of amines to α,β-unsaturated carbonyl compounds is one of the simplest and most effective techniques to produce β-amino carbonyl compounds. Water, ionic liquids, β-cyclodextrin, and polyethylene glycol are among the environmentally friendly products and procedures that have been developed. Moreover, a number of methods have been developed, including simple grinding, mixing, or microwave-assisted addition.127 For example, highly enantioselective β-amination of chalcones 110 with N-bromosuccinimide were reported to form β-imidoketones 112 in high yields,128 as shown in Scheme 36.
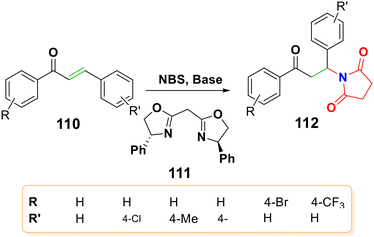 |
| Scheme 36 Aza-Michael addition of amines to chalcones formed β-aminoketones. | |
4.2.1.6.2. Aza-Michael addition of hydrazine derivatives and hydroxylamine to chalcones.
Many biologically active pyrazoles and isoxazoles have been generated by the interaction of chalcones with hydrazine derivatives or hydroxyl amines.129,130 The usage of pyrazoles as antioxidants in fuels, anesthetics, and agricultural uses, as well as in pharmaceuticals and dyes,131 has attracted attention from researchers.132–134 These substances are helpful for materials chemistry, brightening, and electroluminescence.135 They can also be utilized as organic light-emitting diodes,136 liquid crystals,137 and semiconductors.138 Isoxazole, on the other hand, is an azole that contains nitrogen and has an oxygen atom adjacent to it. Ibotenic acid, a natural molecule, and several medications, including the COX-2 inhibitor valdecoxib, contain isoxazole rings. Due to their enormous potential as analgesics, as well as anti-inflammatory,140 anticancer,141 antiviral, anticonvulsant,142 antidepressant, antimicrobial,143 and immunosuppressant properties,144 isoxazole chemistry has long been an interesting area of study.139 Moreover, it was observed that substituted isoxazoles such isoxaben, isouron, and isoxathion were efficient and biodegradable insecticides.145 It was reported that chalcones 113 could be cyclized with hydrazine under mechanochemical ball-milling conditions to produce 3,5-diphenyl-1H-pyrazoles 114,146 as shown in Scheme 37. Also, chalcones 113 could cyclize with hydroxylamine and trichloroisocyanuric acid (TCCA) to produce 3,5-diarylisoxazoles 115.147
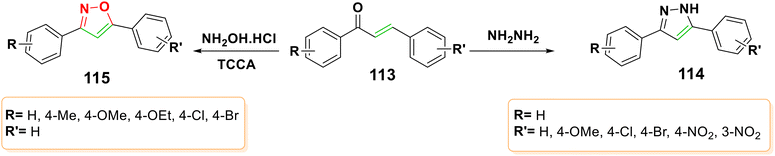 |
| Scheme 37 Synthesis of pyrazoles and isoxazoles via Aza-Michael addition to chalcones. | |
4.2.1.6.3. Aza-Michael addition of urea, guanidine hydrochloride and thiourea to chalcones to form pyrimidines derivatives.
The pyrimidine family is one of the most important and successful groups of chemical molecules. The pyrimidine core is a naturally occurring chemical that can be found in nucleotides, nucleic acids, vitamins, coenzymes, purines, pterins, and uric acids.148 A variety of pyrimidine derivatives have attracted interest due to their intriguing pharmacological properties, such as anticancer, antiviral, antioxidant, antimalarial, antibacterial, antifungal anti-inflammatory, and anti-hypertensive activities.149–159 The reaction of chalcone with guanidine, urea or thiourea forms pyrimidine derivatives.129,160,161 As shown in Scheme 38, chalcone 116 was reported to yield pyrimidine derivative 117 when treated with urea in ethanolic sodium hydroxide.129 Moreover, aryl chalcone 40 reacted with urea and thiourea to yield pyrimidine derivatives 118 and 119 (Scheme 39).
 |
| Scheme 38 Synthesis of pyrimidine from a chalcone using guanidine. | |
 |
| Scheme 39 Synthesis of pyrimidine from chalcone with urea and thiourea. | |
4.2.1.6.4. Aza-Michael addition of o-phenylenediamine to chalcones to form diazepines.
There are numerous medical uses for benzodiazepines. Members of the diazepine family are extensively used for their anticonvulsant, anxiolytic, analgesic, sedative, antidepressant, and hypnotic effects.162,163 Chalcone 120 and o-phenylenediamine 121 were reported to produce a range of benzodiazepines 122
164,165 (Scheme 40).
 |
| Scheme 40 Synthesis of benzodiazepine derivatives. | |
4.2.1.6.5. Aza-Michael addition of azides to chalcones to form triazoles.
The biological uses of triazole derivatives include as anticonvulsants, antimicrobials, antivirals, antituberculars, antidiabetics, anti-inflammatories, antiproliferatives, antioxidants, anti-ureases, and antimalarials.129,166 Triazoles are produced when the chalcone undergoes a [3 + 2] cycloaddition reaction with azide molecules.167,168 Moreover, it was reported that the [3 + 2] cycloaddition reaction of chalcone 123 with 5-azido-5-deoxy-1,2-O-isopropylidene-α-D-xylofuranose 124 using tetrabutylammonium hydrogen sulfate (TBAHS) as a catalyst yielded triazole 125, as shown in Scheme 41.
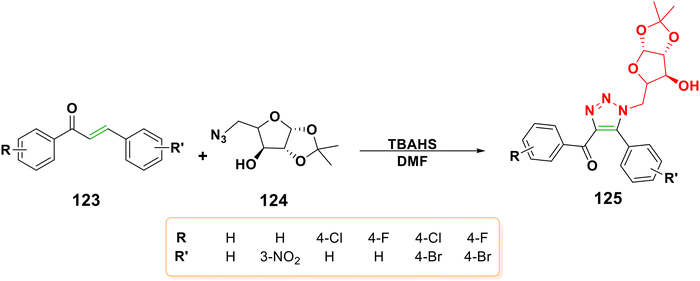 |
| Scheme 41 Synthesis of triazoles derivatives. | |
4.2.1.6.6. Aza-Michael addition of aminoazoles to chalcone to form nitrogen-bridgehead heterocyclic compounds.
5,7-Disubstituted-1,2,4-triazolo[1,5-a]pyrimidine has shown superior in vitro cytotoxicity to that of the anti-cancer drug doxorubicin, acted as an inhibitor of the casein kinase 1δ as a potential treatment for neurological disorders, and shown promising inhibitory activity for the cellular secretion of the hepatitis B virus surface antigen as a potential treatment for HBV infection.169 The cyclocondensation of aminoazoles 127 with chalcones 126 is a typical technique for generating nitrogen-bridgehead pyrimidine derivatives 128,170–172 as shown in Scheme 42.
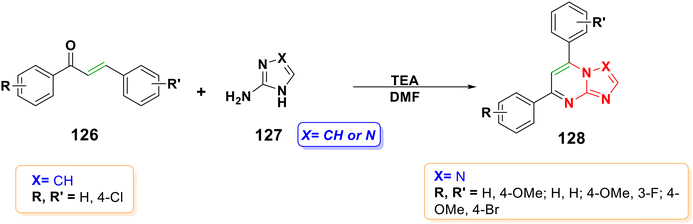 |
| Scheme 42 Synthesis of nitrogen-bridgehead heterocyclic derivatives. | |
4.3. Epoxidation of chalcones
Epoxy chalcone is an interesting heterocyclic intermediate molecule that is an essential building block in the ring opening reaction, which produces compounds with biological activity173 and it is known as ethylene oxide or oxirane.174 The epoxidation of chalcones has received a lot of attention in order to increase yield and enantiomeric excess (ee) values utilizing various types of catalysts175–179 (Scheme 43). Several oxidants have been used in the epoxidation of chalcones, including hydrogen peroxide, dimethyl dioxirane, sodium hypochlorite, sodium peroxide, m-chloroperbenzoic acid, sodium chlorite, sodium perborate tetrahydrate, oxone, cyclohexylidene bishydro peroxide, trichloroisocyanuric acid, and hydrogen peroxide.180–189 According to reports, urea-hydrogen peroxide (UHP) can be used to epoxidize chalcone 129 under sonication to produce products 130 in yields of 78–93%. The use of safer oxidizing agents, non-toxic solvents, lower temperatures, and shorter reaction times are some of the advantages of this approach over the standard one.190 The manufacture of 2′-hydroxychalcone epoxides by direct peracid oxidation of 2′-hydroxychalcone is ineffective. Aqueous acidic dioxane was reported to be used in the synthesis of 2′-hydroxychalcone epoxides, which are intermediates in the production of aurones and flavanones.191
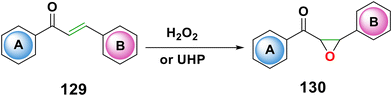 |
| Scheme 43 Epoxidation of chalcone. | |
4.4. Bromination of chalcones
One of the most appealing precursors, α,β-chalcone dibromide, is widely acknowledged as the primary synthon for the production of numerous sets of heterocyclic scaffolds with biological interest. The ability of α,β-chalcone dibromide to undergo a regioselective transformation in a variety of heterocycles is made possible via taking advantage of its accessibility from an inexpensive starting material, its adaptable reactivity due to the carbonyl group, and its readily replaceable bromine atoms under mild conditions.192 Dibromochalcones 131 are produced using a variety of brominating agents, including bromine in various organic solvents, bromine vapour, pyridinium hydrobromide perbromide, phenyl trimethylammonium bromide, (bromodimethyl)-sulfonium bromide, tetrabutylammonium tribromide, and boric acid in potassium bromide solution,192–197 as seen in Scheme 44.
 |
| Scheme 44 Bromination of chalcone. | |
4.5. Diels–Alder cycloaddition reactions of chalcones
Mulberry trees (Morus plants of the family Moraceae) are known for their naturally occurring phenolic compounds known as Mulberry Diels–Alder type adducts (MDAAs) 133.198 They are created biosynthetically through the intermolecular [4 + 2] cycloaddition of dienophiles (mostly chalcones) 129 and dehydroprenylphenol dienes 132, as depicted in Scheme 45. Various Moraceae plant family mulberry Diels–Alder type adducts have been discovered. Based on their structural differences, these can be categorized as four types of adducts: (I) chalcone and prenylated flavonoid (Kuwanon G) adducts; (II) chalcone and prenylstilbene (Kuwanol E) adducts; (III) chalcone and prenylated 2-arylbenzofuran (chalcomoracin) adducts; and (IV) other forms of adducts (bromosin A, mulberrofuran G, or sanggenon S).123 Chalcone can function as a heterodiene in a [4 + 2] cycloaddition as well as a dienophile in a Diels–Alder cycloaddition. This duality is recorded.123 Quantitative yields were obtained for the Diels–Alder annulation between 2′-hydroxychalcone 31 and diene 134 utilizing reusable catalysts that supported AgNPs in silica gel under solvent-free conditions, producing endo/exo product 135
199 (Scheme 46).
 |
| Scheme 45 MDAA biosynthesis pathway. | |
 |
| Scheme 46 Diels–Alder reaction of chalcone initiated with single-electron transfer. | |
It was reported that chalcones 137 and allenoates 136 experienced a hetero-Diels–Alder and isomerization process that was catalyzed by N-heterocyclic carbenes (NHCs), yielding highly functionalized multisubstituted pyranyl carboxylates 138
200 (Scheme 47).
 |
| Scheme 47 Hetero-Diels–Alder reaction between chalcone and allenoates with N-heterocyclic carbene catalysis. | |
5. Applications of chalcones
5.1. Chalcones as innovative and extraordinarily adaptable photoinitiators for polymerization under visible light
Chalcones have come into their own as creative and flexible photoinitiators for visible light polymerization techniques. Photoinitiators are substances that, when exposed to light, produce reactive species that both start and speed up the polymerization reaction. Photoinitiators have traditionally been developed for use with ultraviolet (UV) light, which restricts their use in several polymerization procedures. But new opportunities and benefits have emerged with the introduction of chalcones as photoinitiators for visible light polymerization. Main benefit of employing chalcones as photoinitiators for visible light polymerization is the availability of safer and more energy-efficient visible light sources, such as LED lamps, as opposed to UV lamps. Chalcone-based photoinitiators permit polymerization procedures to be carried out under more controlled settings through utilizing visible light, preventing any potential harm or degradation that could result from exposure to UV radiation.201 These structures are remarkably adaptable and capable of reaching photoinitiating systems for a variety of photoprocesses, such as the synthesis of interpenetrated polymer networks (IPNs), cationic polymerization (CP) of epoxides and vinyl ethers, free radical polymerization (FRP) of acrylates, and the thiol–ene reactions (TERs).202 These photoprocesses were investigated for five hydroxyl chalcones, as indicated in Fig. 5. Compared with other chalcones, chalcone with a dimethyl amino group, which is a strong electron-donating group, exhibits the greatest red-shifted absorption. Only 141 and 142 would be appropriate for visible light photopolymerization, according to their absorptions. Due to the weak electron donating groups connected to the cinnamoyl group, chalcones 31 and 139–142 were unable to produce a sufficient push–pull force that would move the intramolecular charge transfer (ICT) band into the visible range (H, Me, OC8H17) (Fig. 5).
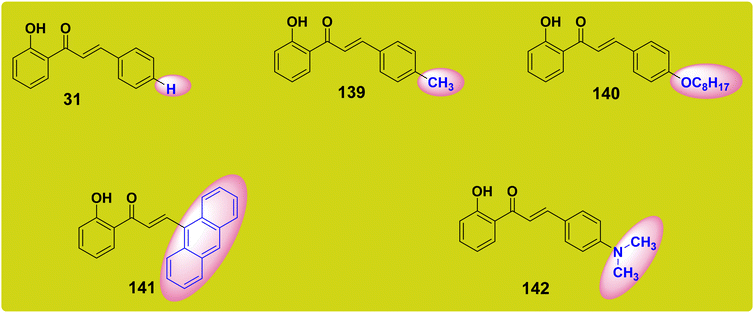 |
| Fig. 5 Structures of some photosensitizers. | |
5.2. Chalcone derivatives as fluorescence turn-on chemosensors
The visual simplicity, rapid reaction, selectivity, and sensitivity of fluorescent chemosensors make them distinctive in that they function as a molecular stopwatch that begins when the light is turned on and ends when it is turned off; this is usually employed to explore the molecular process.203 Thus, it is a fascinating objective to create such molecular systems that can permit biomolecules and metal ions to be detected by the human eye. Chalcone derivatives are well-known tiny molecular sensors noted for their affordability, flexibility in structural alteration, and capacity to vary their emission properties in response to binding ions or changes in the immediate environment. Fluorescent chalcones were found to be good research tools for cellular targets.204,205 The structural variety of chalcone derivatives may provide additional benefits for recognizing specific analytes. For rapid anion detection, o-hydroxyl and carbonyl groups can show effective intramolecular hydrogen bonding. Moreover, rings A and B can employ a large number of heteroatoms to selectively interact with metal ions. Additionally, the selectivity and sensitivity of both aromatic rings can be altered by adding other substituents.204 It is observed that o-hydroxy chalcone 143, which has the terminal electron acceptor group 4-nitrobenzene, works well as a chemosensor for cyanide anions in CH3CN–H2O (1
:
1, v/v) solution.206 Nitro-substituted chalcone derivative 144 is also reported to be a quick-acting chemosensor for cyanide anions, where the chalcone with the diethylamino terminal group shows blatant changes in fluorescence from orange to green when cyanide anions are introduced.207 Additionally, two indole chalcone compounds with bromine substitutions, 145 and 146, were discovered to operate as cyanide anion chemosensors. 4-(Dimethylamino)-2′-hydroxychalcone 142 was reported251 as a colorimetric naked-eye detection chemosensor for Al3+ and Cu2+ ions. This receptor functioned as a highly selective colorimetric turn-on sensor when in contact with Al3+ ions via changing color from yellow to red, and as a turn-off sensor when in contact with Cu2+ ions. In many domains of biotechnology, such as medical diagnostics, food safety, and anti-bioterrorism, the recognition of biomolecules is vital; nevertheless, water solubility is the main requirement for bio probes. The creation of sensitive bio probes that can perform effectively in an aqueous medium has been hampered by the fact that many traditional fluorescent probes exhibit fluorescence quenching on aggregation in aqueous conditions.204,208 In light of this, a chalcone containing a phosphate unit was used to detect alkaline phosphatase (ALP) activity and aid in its visualization in living cells. The probe emits a greenish-yellow hue in aqueous buffers and is water soluble. In the presence of ALP, the phosphate group on this molecule is broken down, resulting in the insoluble enzyme product (2′-hydroxy chalcone derivative 142), which emits red fluorescence.208 According to reports, hydroxychalcone dyes 148 act as pH and fluoride ion color indicators209 (Fig. 6).
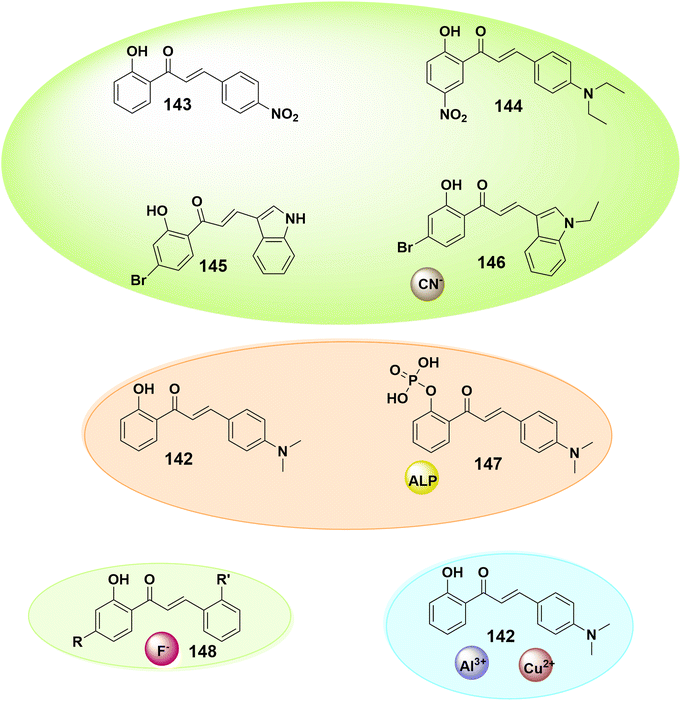 |
| Fig. 6 Derivatives of chalcone used for sensing applications. | |
5.3. Potential antioxidant additives in biofuels
Due to depletion and the pollution caused from petroleum-based fuels, renewable fuel sources have been sought and improved during the past two decades.210 Biofuels, such as ethanol, biodiesel, and biogas, are examples of a fuel that can be created from a renewable source using diverse plant, animal, and biomass sources. Moreover, biofuels in their solid, liquid, or gaseous physical forms can represent a number of known fuels.210–212 Biodiesel's drawbacks (poor oxidation stability, low storage stability, and poorer energy availability) can be reduced by adding additives that increase fuel stability through the development of technologies that increase fuel stability and produce greater energy efficiency.213–215 Antioxidants suppress chain-breaking and the hydroperoxide decomposer, which is crucial for preventing the oxidation-based break down of biodiesel.216 Chalcones work as antioxidants to improve the oxidative stability of biodiesel, according to recent studies.217,218 Hydroxychalcones were already found to be extremely powerful antioxidants in biodiesel, and chalcone 149 in Fig. 7 exhibited the highest antioxidant activity.217
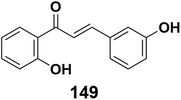 |
| Fig. 7 Structure of 2′-hydroxychalchone. | |
5.4. Chalcones as useful nonlinear optical (NLO) chromophores
Due to recent developments in the field of nonlinear optics (NLO), second- and third-order nonlinear optical materials have found their way into practical applications and devices such as photodynamic therapy, optical limiting, optical data storage, 3D microfabrication, and two-photon excited fluorescence microscopy. The chalcone core has a useful NLO chromophore, with design strategy identified through an examination of past investigations. It is found that an alkene-bridge can bridge moieties more effectively than an alkyne-bridge. It is determined that the higher degree of conjugation is the cause of their efficiency. Dialkyl and diaryl-based donors are more efficient than oxygen-based donors, with phenoxide being the most efficient.219 Chalcone derivatives are promising possibilities due to their high damage threshold, large NLO coefficients, good crystallizability, and superior blue light transmission.220 Thiophene chalcone derivative 150 under a continuous wave laser (CW = 532 nm) is interesting block for NLO applications, according to Z-scan investigations.221 Also, Pyrene-based chalcone 151 was also created in consideration of the molecular arrangement, role of donor–acceptor substituents, ICT factors, and low energy gap, all of which are significant requirements in the hunt for a good NLO response for NLO applications222 (Fig. 8).
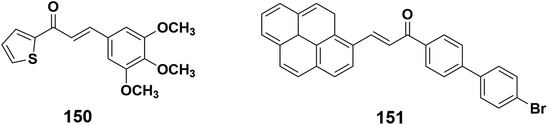 |
| Fig. 8 Chalcones which are promising for NLO applications. | |
5.5. Stimuli-responsive host–guest systems
Host–guest chemistry is a special for supramolecular chemistry that studies the molecular recognition (noncovalent binding) of small molecules or ions (guests) with larger receptors (hosts) to form a host–guest complex.223 A simple host–guest complex is generated when a cation is attached to a synthetic macrocycle, like a crown ether. Enzymes and their substrates are examples of host–guest complexes. Low molecular weight synthetic compounds having well-defined and structurally preorganized binding sites, such as macrocycles, molecular clips, and tweezers, can attract small guest molecules or ions.224trans-Chalcone (Ct), one of many synthetic flavylium multistates, is thought to be the most stable species under acidic conditions. The fact that Ct has a far higher affinity for β-cyclodextrin (β-CD) than it does for the flavylium cation (AH+) and quinoidal base (A), which are often the last species formed following photoirradiation of Ct, makes it possible to create robust photoresponsive host–guest complexes. It was found that when Ct 152 was exposed to radiation in the presence of 8 mM β-CD (K = 9 × 103 M−1, 88% of the complex), AH+153 was generated. Because AH+ has a low affinity for cyclodextrin (K = 10 M−1, 8% of the complex), radiation causes it to be (photo)ejected from the host cavity225 (Scheme 48).
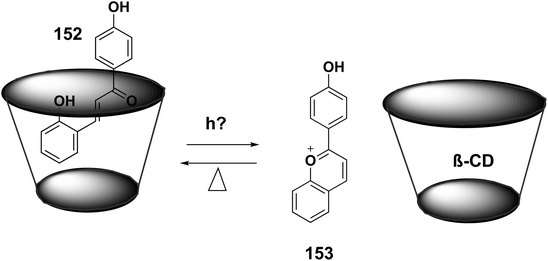 |
| Scheme 48 Host–guest complex of chalcone with β-cyclodextrin. | |
5.6. Biological applications
Studies on the potential therapeutic benefits of chalcones have covered a wide range of topics, including the treatment of cancer, diabetes, inflammation, and microbial infections. However, its poor absorption and metabolic stability have reduced its therapeutic utility. Pharmacokinetic enhancement techniques such prodrug synthesis, nanoparticle encapsulation, and chemical modification have been proposed to work around these limitations.226,227,252
5.6.1. Chalcones as anti-gout agents.
Due to their anti-inflammatory and uric acid-lowering qualities, chalcones have been researched for their potential as anti-gout medications. A key factor in the onset and progression of gout is inflammation. By blocking inflammatory mediators and enzymes like cyclooxygenase (COX) and lipoxygenase (LOX), which are involved in the generation of inflammatory chemicals, chalcones have shown anti-inflammatory efficacy. Chalcones may lessen gout-related pain and edema by lowering inflammation. Fig. 9 displays reported chalcone scaffold compounds 3, 4, 10, and 154–157 with potent anti-gout activity.66 In fact, the chalcone hydroxyl group (–OH) is crucial for how well they interact with biological targets. In order to bind to these targets and inhibit or modulate them, chalcones need to create hydrogen bonds as well as other van der Waals forces. Between certain amino acid residues or functional groups in the biological target and the hydroxyl group of chalcones, hydrogen bonding is a crucial intermolecular interaction that takes place. The attraction between a hydrogen atom bound to an oxygen or nitrogen atom and the electronegative oxygen atom of the hydroxyl group is known as a hydrogen bond. The chalcone target complex may become more stable due to this interaction, which may also make binding easier.
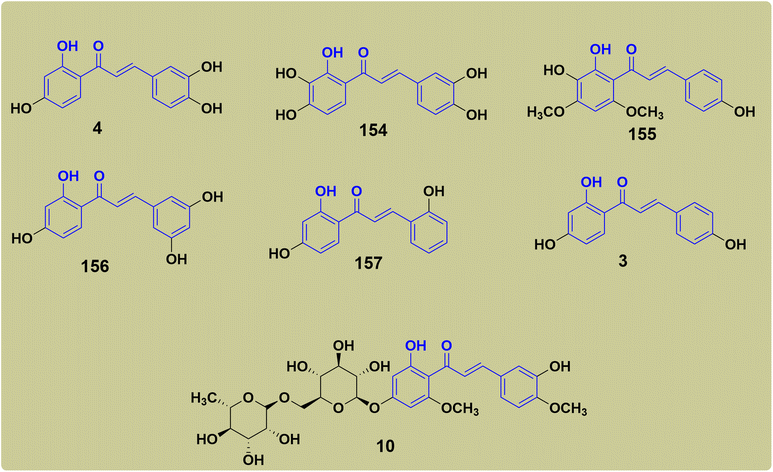 |
| Fig. 9 Structures of some chalcone scaffold compounds. | |
5.6.2. Chalcones with antiviral activities.
Indeed, the potential of chalcones as pharmacological agents against a range of human viruses, including the coronavirus linked to severe acute respiratory syndrome (SARS-CoV), has been studied. Research into the antiviral capabilities of chalcones has been sparked by the introduction of novel viral infections and the demand for efficient antiviral therapeutics. The antiviral activity of chalcones against several viruses, including SARS-CoV and other coronaviruses, has been investigated in a number of investigations. By concentrating on several viral life cycle stages, such as viral entry, viral RNA synthesis, and viral protein production, chalcones have demonstrated inhibitory effects on viral replication.228,229 Chalcones have also shown antiviral efficacy against various human viruses, including the herpes simplex virus (HSV), hepatitis viruses, HIV, influenza viruses, and human immunodeficiency virus (HIV). They have been demonstrated to suppress viral gene expression, lower viral load, and prevent viral replication such as MERS-CoV,230 HIV,10,231–233 influenza virus,234–236 herpes simplex virus (HSV),237 dengue virus (DEN),238 human rhinovirus (HRV)239,240 and hepatitis C virus (HCV).241–243 Several human viruses have been found to react with hydroxyl chalcone derivatives 3, 4, 6, 60, and 158–168, as shown in Fig. 10.
 |
| Fig. 10 Some chalcones anticipated to have antiviral activities. | |
5.6.3. Chalcones in dermatology.
Chalcones also showed potential for treating several dermatitis and skin rash diseases. Inflammation, high sebum production, or aberrant skin cell proliferation are characteristics of acne vulgaris, seborrheic dermatitis, dandruff, and psoriasis. Chalcones are prospective options for treating these disorders because of their ability to reduce inflammation, regulate sebum production, and limit the growth of harmful bacteria. They are promising for treating various skin conditions, but more investigations and clinical studies are required to determine their effectiveness and safety for particular uses. Another area of active study is the creation of chalcone-based formulations and delivery systems that are optimised for skin penetration and stability. For example, the novel sunscreen molecule called (E)-3-(5-bromofuran-2-yl)-1-(2-hydroxyphenyl)prop-2-en-1-one (169) is reported to address the concerns of traditional sunscreen molecules that have only one energy relaxation pathway and therefore do not provide UVA light protection.244 Moreover, several chalcone analogues 170 were reported to have enhanced selectivity in vitro against promastigotes and intracellular amastigotes of Leishmania amazonensis245 (Fig. 11). So chalcones offer a wide range of possible advantages for maintaining healthy skin and treating a number of skin conditions. They are excellent candidates for further research in the area of dermatology and skin care due to their distinctive bioactivities, which include anti-inflammatory, tyrosinase inhibition, antileishmanial, and antibacterial characteristics.
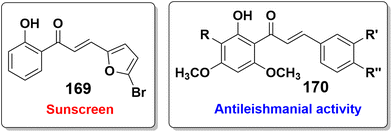 |
| Fig. 11 Some chalcones suitable for dermatology applications. | |
5.6.4. Chalcones as antibacterial agents.
The creation of alternative compounds to tackle bacterial resistance to several drugs is now necessary due to the rising prevalence of antibiotic resistance. Due to their diverse structural and wide variety of pharmacological activities, chalcone-based compounds have become attractive candidates in the search for new therapeutic agents. Chalcones’ structural diversity enable tweaks and changes to their chemical composition, which might improve their antibacterial effects. To find molecules with strong antibacterial action against multidrug-resistant pathogens, researchers have investigated the production and screening of different chalcone derivatives. Both Gram-positive and Gram-negative bacteria have been significantly reduced by chalcones’ antibacterial properties. They have shown effectiveness against a variety of pathogenic strains, including ESBL-producing bacteria and drug-resistant bacteria like methicillin-resistant Staphylococcus aureus (MRSA) and vancomycin-resistant enterococci (VRE).48,232 Chalcones have also been studied for their potential synergistic interactions with other antibiotics. By lowering the minimum inhibitory concentration (MIC) of the antibiotics or by making them effective again against resistant strains, they have demonstrated the ability to improve the efficacy of antibiotics against drug-resistant bacteria. This strategy, known as antibiotic adjuvant therapy, may be able to circumvent resistance processes and increase the potency of already available antibiotics. An MIC range of 2 g mL−1 was used to assess the antibacterial activity of chalcones from Angelica keiskei that include prenyl or geranyl groups 6, 8, and 171–174 (Fig. 12).246
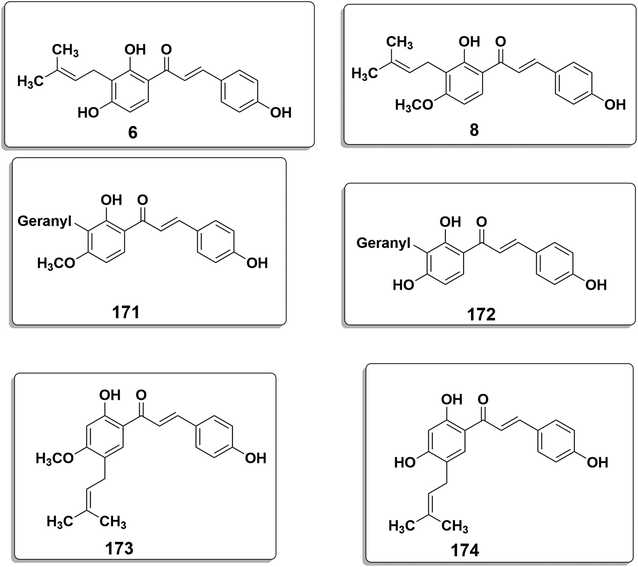 |
| Fig. 12 Chalcones bearing phenyl or geranyl groups with antibacterial activity. | |
5.6.5. Chalcones as tubulin polymerization inhibitors.
It was discovered that chalcone derivatives bound with microtubule proteins, particularly tubulin, and obstructed the dynamic assembly and disassembly of these proteins. Chalcones can impair microtubule development by attaching to tubulin, which results in aberrant spindle formation and cell division arrest, ultimately preventing the proliferation of tumor cells.
By targeting the vasculature of solid tumors, a number of chalcone derivatives, including combretastatin A-4, have demonstrated strong anti-tumor action. These substances have the ability to damage the microtubule network in the tumor's blood vessels, reducing blood flow and ultimately causing tumor necrosis.247 For the human neuroepithelioma cell lines SK-N-MC and K562, Aryapour and associates examined the cytotoxic effects of several chloro-substituted 2′-hydroxychalcones as well as their function as tubulin polymerization inhibitors. One of these chemicals, 3,5′-dichloro-derivative (175), inhibited 89% protofilament production. Additionally, it was discovered that the chemical had altered the far-UV circular dichroism spectra of tubulin and had a dissociation constant of 3.7 M for tubulin.248 Fuurthermore, new chalcones 176 and 177 containing fluoro atoms were tested for their capacity to stop cell proliferation using a panel of human cancer cell lines. Two hydroxy chalcones demonstrated negligible cytotoxicity for healthy HEK 293 cells but had potent anti-proliferative effects on several human cancer cells.249 Shin et al. found that 2-hydroxy-4-methoxy-2′,3′-benzochalcone 178 was the most effective treatment against the clonogenicity of Capan-1 human pancreatic cancer cells. It also prevented tumor growth in nude mice250 (Fig. 13).
 |
| Fig. 13 Chalcones as tubulin polymerization inhibitors. | |
6. Conclusion
This review provides a brief overview of chalcone chemistry and discusses different methods for synthesizing and describing chalcone derivatives, which have a wide range of thier biological activities, potential medical uses and industrial applications. Also, chalcones are involved in different Michael addition reactions and act as precursors for other substances like flavonoids and coumarins through these processes; thus, they have attracted attention of scientists due to their distinct structures and pharmacological characteristics. Additionally, chalcones are adaptable and can be used for synthesis of several products for food, medicine, cosmetic, and skincare applications. Chalcone derivatives are fascinating compounds with numerous applications in the medicinal, pharmacological, and optical fields.
Conflicts of interest
There are no conflicts to declare.
References
- Y. Guo, L. Ding, S. Ghidinelli, C. H. Gotfredsen, M. de la Cruz, T. A. Mackenzie, M. C. Ramos, P. Sánchez, F. Vicente and O. Genilloud, J. Nat. Prod., 2021, 84, 979–985 CrossRef CAS PubMed
.
- A. G. Atanasov, B. Waltenberger, E.-M. Pferschy-Wenzig, T. Linder, C. Wawrosch, P. Uhrin, V. Temml, L. Wang, S. Schwaiger and E. H. Heiss, Biotechnol. Adv., 2015, 33, 1582–1614 CrossRef CAS PubMed
.
- M. S. Butler, J. Nat. Prod., 2004, 67, 2141–2153 CrossRef CAS PubMed
.
- G. M. Cragg, P. G. Grothaus and D. J. Newman, Chem. Rev., 2009, 109, 3012–3043 CrossRef CAS PubMed
.
- W. S. Tay, Y. Li, Y. Lu, S. A. Pullarkat and P.-H. Leung, Organometallics, 2020, 39, 271–278 CrossRef CAS
.
- S. Bernardini, A. Tiezzi, V. Laghezza Masci and E. Ovidi, Nat. Prod. Res., 2018, 32, 1926–1950 CrossRef CAS PubMed
.
- B. David, J.-L. Wolfender and D. A. Dias, Phytochem. Rev., 2015, 14, 299–315 CrossRef CAS
.
- M. Zheng, C. Huang, J.-Z. Yan, S.-L. Xie, S.-J. Ke, H.-D. Xia and Y.-N. Duan, J. Org. Chem., 2023, 88, 1504–1514 CrossRef CAS PubMed
.
- X. Qiu, L. Zhu, H. Wang, Y. Tan, Z. Yang, L. Yang and L. Wan, Biorg. Med. Chem., 2021, 52, 116510 CrossRef CAS PubMed
.
- Z. Nowakowska, Eur. J. Med. Chem., 2007, 42, 125–137 CrossRef CAS PubMed
.
- B. E. Aksöz and R. Ertan, Fabad J. Pharm. Sci., 2011, 36, 223–242 Search PubMed
.
- M. N. Gomes, E. N. Muratov, M. Pereira, J. C. Peixoto, L. P. Rosseto, P. V. Cravo, C. H. Andrade and B. J. Neves, Molecules, 2017, 22, 1210 CrossRef PubMed
.
- M. A. Shalaby, A. M. Fahim and S. A. Rizk, Sci. Rep., 2023, 13, 4999 CrossRef CAS PubMed
.
- A. Rammohan, J. S. Reddy, G. Sravya, C. N. Rao and G. V. Zyryanov, Environ. Chem. Lett., 2020, 18, 433–458 CrossRef CAS
.
- C. Díaz-Tielas, E. Graña, M. Reigosa and A. Sánchez-Moreiras, J. Planta Daninha, 2016, 34, 607–616 CrossRef
.
- R. Mata, J. Nat. Prod., 2007, 70, 140–140 CrossRef CAS
.
- C. Zhuang, W. Zhang, C. Sheng, W. Zhang, C. Xing and Z. Miao, Chem. Rev., 2017, 117, 7762–7810 CrossRef CAS PubMed
.
- M. Rudrapal, J. Khan, A. A. B. Dukhyil, R. M. I. I. Alarousy, E. I. Attah, T. Sharma, S. J. Khairnar and A. R. Bendale, Molecules, 2021, 26, 7177 CrossRef CAS PubMed
.
- S. Ammaji, S. Masthanamma, R. R. Bhandare, S. Annadurai and A. B. Shaik, Arabian J. Chem., 2022, 15, 103581 CrossRef CAS
.
- G. Rajendran, D. Bhanu, B. Aruchamy, P. Ramani, N. Pandurangan, K. N. Bobba, E. J. Oh, H. Y. Chung, P. Gangadaran and B.-C. Ahn, Pharmaceuticals, 2022, 15, 1250 CrossRef CAS PubMed
.
-
R. Watson, V. R. Preedy and S. Zibadi, Polyphenols: prevention and treatment of human disease, Academic press, 2018 Search PubMed
.
- C. X. Qin, X. Chen, R. A. Hughes, S. J. Williams and O. L. Woodman, J. Med. Chem., 2008, 51, 1874–1884 CrossRef CAS PubMed
.
- N. Abu, W. Y. Ho, S. K. Yeap, M. N. Akhtar, M. P. Abdullah, A. R. Omar and N. B. Alitheen, Cancer Cell Int., 2013, 13, 1–7 CrossRef PubMed
.
- M. Feldman, S. Tanabe, F. Epifano, S. Genovese, M. Curini and D. Grenier, J. Nat. Prod., 2011, 74, 26–31 CrossRef CAS PubMed
.
- G. Padmavathi, N. K. Roy, D. Bordoloi, F. Arfuso, S. Mishra, G. Sethi, A. Bishayee and A. B. Kunnumakkara, Phytomedicine, 2017, 25, 118–127 CrossRef CAS PubMed
.
-
H. Wang, Comprehensive Organic Name Reactions, Wiley, Germany, 2010 Search PubMed
.
- J. Park, J. Yun, J. Kim, D.-J. Jang, C. H. Park and K. Lee, Synth. Commun., 2014, 44, 1924–1929 CrossRef CAS
.
- Y.-S. Kil, S. T. Pham, E. K. Seo and M. Jafari, Arch. Pharmacal Res., 2017, 40, 655–675 CrossRef CAS PubMed
.
-
J. R. Lakowicz, Principles of fluorescence spectroscopy, Springer, 2006 Search PubMed
.
- K. E. Sapsford, L. Berti and I. L. Medintz, Angew. Chem., Int. Ed., 2006, 45, 4562–4589 CrossRef CAS PubMed
.
- I. E. Serdiuk, M. Wera and A. D. Roshal, J. Phys. Chem. A, 2018, 122, 2030–2038 CrossRef CAS PubMed
.
- Y. Leydet, P. Batat, G. Jonusauskas, S. Denisov, J. C. Lima, A. J. Parola, N. D. McClenaghan and F. Pina, J. Phys. Chem. A, 2013, 117, 4167–4173 CrossRef CAS PubMed
.
- N. Yaylı, Y. Gök, O. Üçüncü, A. Y. Ç. Atasoy, E. Şahinbaş and M. Küçük, J. Chem. Res., 2005, 2005, 155–159 CrossRef
.
- A. M. Fahim, H. E. M. Tolan, H. Awad and E. H. I. Ismael, J. Iran. Chem. Soc., 2021, 18, 2965–2981 CrossRef CAS
.
- J. B. Harborne and T. J. Mabry, J. Chem. Educ., 1995, 72, A73 CAS
.
- K. Tokumura, K. Nagaosa, Y. Ohta and R. Matsushima, Chem. Phys. Lett., 1998, 295, 516–524 CrossRef CAS
.
- T. Teshima, M. Takeishi and T. Arai, New J. Chem., 2009, 33, 1393–1401 RSC
.
- L. Zhang, J. Liu, J. Gao, R. Lu and F. Liu, RSC Adv., 2017, 7, 46354–46357 RSC
.
- K. Komarova, S. Sakipov, V. Plotnikov and M. Alfimov, J. Lumin., 2015, 164, 57–63 CrossRef CAS
.
- A. D'Aléo, A. Karapetyan, V. Heresanu, M. Giorgi and F. Fages, Tetrahedron, 2015, 71, 2255–2259 CrossRef
.
- A. Karuppusamy, T. Vandana and P. Kannan, J. Photochem. Photobiol., A, 2017, 345, 11–20 CrossRef CAS
.
- E. Colucci-Guyon, A. S. Batista, S. D. Oliveira, M. Blaud, I. C. Bellettini, B. S. Marteyn, K. Leblanc, P. Herbomel and R. Duval, Chem. Sci., 2019, 10, 3654–3670 RSC
.
- F. Yu, M. Wang, H. Sun, Y. Shan, M. Du, A. Khan, R. Usman, W. Zhang, H. Shan and C. Xu, RSC Adv., 2017, 7, 8491–8503 RSC
.
- H. E. M. Tolan, A. M. Fahim and E. H. I. Ismael, J. Mol. Struct., 2023, 1283, 135238 CrossRef CAS
.
- W. Wu, H. Ye, L. Wan, X. Han, G. Wang, J. Hu, M. Tang, X. Duan, Y. Fan and S. He, Carcinogenesis, 2013, 34, 1636–1643 CrossRef CAS PubMed
.
- S.-W. Yun, N.-Y. Kang, S.-J. Park, H.-H. Ha, Y. K. Kim, J.-S. Lee and Y.-T. Chang, Acc. Chem. Res., 2014, 47, 1277–1286 CrossRef CAS PubMed
.
- D. K. Mahapatra, S. K. Bharti and V. Asati, Eur. J. Med. Chem., 2015, 101, 496–524 CrossRef CAS PubMed
.
- W. Dan and J. Dai, Eur. J. Med. Chem., 2020, 187, 111980 CrossRef CAS PubMed
.
- S. Nasir Abbas Bukhari, M. Jasamai and I. Jantan, Mini-Rev. Med. Chem., 2012, 12, 1394–1403 Search PubMed
.
- M. M. Amin, G. E.-D. A. Abuo-Rahma, M. S. A. Shaykoon, A. A. Marzouk, M. A. S. Abourehab, R. E. Saraya, M. Badr, A. M. Sayed and E. A. M. Beshr, Bioorg. Chem., 2023, 134, 106444 CrossRef CAS PubMed
.
- H. Qian, D. Liu and C. Lv, Ind. Eng. Chem. Res., 2011, 50, 1146–1149 CrossRef CAS
.
- T. Szell and I. Sohar, Can. J. Chem., 1969, 47, 1254–1258 CrossRef CAS
.
- B. M. Ivković, K. Nikolic, B. B. Ilić, Ž. S. Žižak, R. B. Novaković, O. A. Čudina and S. M. Vladimirov, Eur. J. Med. Chem., 2013, 63, 239–255 CrossRef PubMed
.
- M. A. Shalaby, H. M. Al-Matar, A. M. Fahim and S. A. Rizk, J. Phys. Chem. Solids, 2022, 170, 110933 CrossRef CAS
.
- R. Zong and T. Wang, Ind. Eng. Chem. Res., 2022, 61, 1294–1300 CrossRef CAS
.
- M. Sisa, S. L. Bonnet, D. Ferreira and J. H. Van der Westhuizen, Molecules, 2010, 15, 5196–5245 CrossRef CAS PubMed
.
- H. Obara, H. Takahashi and H. Hirano, Bull. Chem. Soc. Jpn., 1969, 42, 560–561 CrossRef CAS
.
- H. Obara and H. Takahashi, Bull. Chem. Soc. Jpn., 1967, 40, 1012–1012 CrossRef CAS
.
- V. K. Bhatia and J. Kagan, Chem. Ind., 1970, 1203 CAS
.
- V. Ramakrishnan and J. Kagan, J. Org. Chem., 1970, 35, 2901–2904 CrossRef CAS
.
- P. Pawluc, J. Szudkowska, G. Hreczycho and B. Marciniec, J. Org. Chem., 2011, 76, 6438–6441 CrossRef CAS PubMed
.
- P. Pawluć, Catal. Commun., 2012, 23, 10–13 CrossRef
.
- X.-Z. Deng, Z.-Y. Chen, Y. Song, F. Xue, M. Yamane and Y.-N. Yue, J. Org. Chem., 2021, 86, 12693–12704 CrossRef CAS PubMed
.
- J. Szudkowska-Frątczak, G. Hreczycho and P. Pawluć, Org. Chem. Front., 2015, 2, 730–738 RSC
.
- M. N. Gomes, E. N. Muratov, M. Pereira, J. C. Peixoto, L. P. Rosseto, P. V. Cravo, C. H. Andrade and B. J. Neves, Molecules, 2017, 22, 1210 CrossRef PubMed
.
- D. K. Mahapatra, V. Asati and S. K. Bharti, J. Crit. Rev., 2019, 6, 1–5 CrossRef
.
- X.-F. Wu, H. Neumann, A. Spannenberg, T. Schulz, H. Jiao and M. Beller, J. Am. Chem. Soc., 2010, 132, 14596–14602 CrossRef CAS PubMed
.
- N. A. Elkanzi, H. Hrichi, R. A. Alolayan, W. Derafa, F. M. Zahou and R. B. Bakr, ACS Omega, 2022, 7, 27769–27786 CrossRef CAS PubMed
.
- X. F. Wu, H. Neumann and M. Beller, Synfacts, 2010, 2010, 1180–1180 CrossRef
.
- S. Eddarir, N. Cotelle, Y. Bakkour and C. Rolando, Tetrahedron Lett., 2003, 44, 5359–5363 CrossRef CAS
.
- T. Ramar, M. A. Subbaiah and A. Ilangovan, J. Org. Chem., 2022, 87, 4508–4523 CrossRef CAS PubMed
.
- B.-W. Xin, Synth. Commun., 2008, 38, 2826–2837 CrossRef CAS
.
- K. R. Buszek and N. Brown, Org. Lett., 2007, 9, 707–710 CrossRef CAS PubMed
.
- A. Kumar, S. Sharma, V. D. Tripathi and S. Srivastava, Tetrahedron, 2010, 66, 9445–9449 CrossRef CAS
.
- F. Ramirez and S. Dershowitz, J. Org. Chem., 1957, 22, 41–45 CrossRef CAS
.
- C. Xu, G. Chen and X. Huang, Org. Prep. Proced. Int., 1995, 27, 559–561 CrossRef CAS
.
- A. Sharma and A. Saraswat, J. Indian Chem. Soc., 2021, 98, 100028 CrossRef CAS
.
- C. Thiot, C. Mioskowski and A. Wagner, Eur. J. Org. Chem., 2009, 2009, 3219–3227 CrossRef
.
- J. W. Labadie and J. Stille, J. Am. Chem. Soc., 1983, 105, 6129–6137 CrossRef CAS
.
- T. Yamakawa, H. Kinoshita and K. Miura, J. Organomet. Chem., 2013, 724, 129–134 CrossRef CAS
.
- A. M. Kearney, L. Murphy, C. C. Murphy, K. S. Eccles, S. E. Lawrence, S. G. Collins and A. R. Maguire, Tetrahedron, 2021, 88, 132091 CrossRef CAS
.
- S. Seo, M. Gao, E. Paffenholz and M. C. Willis, ACS Catal., 2021, 11, 6091–6098 CrossRef CAS PubMed
.
- Q. Jiang, J. Jia, B. Xu, A. Zhao and C.-C. Guo, J. Org. Chem., 2015, 80, 3586–3596 CrossRef CAS PubMed
.
- S. Wu, H. Yu, Q. Hu, Q. Yang, S. Xu and T. Liu, Tetrahedron Lett., 2017, 58, 4763–4765 CrossRef CAS
.
- S. Tanaka, T. Kunisawa, Y. Yoshii and T. Hattori, Org. Lett., 2019, 21, 8509–8513 CrossRef CAS PubMed
.
- X.-W. Zhang, G.-Q. Jiang, S.-H. Lei, X.-H. Shan, J.-P. Qu and Y.-B. Kang, Org. Lett., 2021, 23, 1611–1615 CrossRef PubMed
.
- K. Lee and Y. Hae Kim, Synth. Commun., 1999, 29, 1241–1248 CrossRef CAS
.
- H. A. Samimi, Z. Shams and A. R. Momeni, J. Iran. Chem. Soc., 2012, 9, 705–708 CrossRef CAS
.
- H. A. Samimi, H. Kiyani and Z. Shams, J. Chem. Res., 2013, 37, 282–284 CrossRef CAS
.
- H. A. Samimi, E. Salehi and F. Dadvar, J. Chem. Res., 2014, 38, 731–733 CrossRef CAS
.
- M. N. Pennell, M. G. Unthank, P. Turner and T. D. Sheppard, J. Org. Chem., 2011, 76, 1479–1482 CrossRef CAS PubMed
.
- M. Yu, G. Li, S. Wang and L. Zhang, Adv. Synth. Catal., 2007, 349, 871–875 CrossRef CAS
.
- D. A. Engel and G. B. Dudley, Org. Lett., 2006, 8, 4027–4029 CrossRef CAS PubMed
.
- Y. Sugawara, W. Yamada, S. Yoshida, T. Ikeno and T. Yamada, J. Am. Chem. Soc., 2007, 129, 12902–12903 CrossRef CAS PubMed
.
- F. Justaud, A. Hachem and R. Grée, Eur. J. Org. Chem., 2021, 2021, 513–513 CrossRef CAS
.
- A. S. El Douhaibi, Z. M. A. Judeh, H. Basri, Z. Moussa, M. Messali and G. Qi, Synth. Commun., 2011, 41, 533–540 CrossRef CAS
.
- Y. Masuyama, W. Takamura and N. Suzuki, Eur. J. Org. Chem., 2013, 2013, 8033–8038 CrossRef CAS
.
- Y. Zhao and Q. Song, Org. Chem. Front., 2016, 3, 294–297 RSC
.
- V. Murugesan, A. Muralidharan, G. V. Anantharaj, T. Chinnusamy and R. Rasappan, Org. Lett., 2022, 24, 8435–8440 CrossRef CAS PubMed
.
- K. Kokubo, K. Matsumasa, M. Miura and M. Nomura, J. Org. Chem., 1997, 62, 4564–4565 CrossRef CAS
.
- M.-A. Karadendrou, I. Kostopoulou, V. Kakokefalou, A. Tzani and A. Detsi, Catalysts, 2022, 12, 249 CrossRef CAS
.
- I. B. Masesane, Int. J. Chem. Stud., 2015, 3, 53–59 Search PubMed
.
- S. Nayak, S. Chakroborty, S. Bhakta, P. Panda and S. Mohapatra, Res. Chem. Intermed., 2016, 42, 2731–2747 CrossRef CAS
.
- K. X. Nguyen, P. H. Pham, T. T. Nguyen, C.-H. Yang, H. T. B. Pham, T. T. Nguyen, H. Wang and N. T. S. Phan, Org. Lett., 2020, 22, 9751–9756 CrossRef CAS PubMed
.
- S. G. Scrivener, Y. Wang and Y.-M. Wang, Org. Lett., 2023, 25, 1420–1424 CrossRef CAS PubMed
.
- K. Endo, D. Hamada, S. Yakeishi, M. Ogawa and T. Shibata, Org. Lett., 2012, 14, 2342–2345 CrossRef CAS PubMed
.
- N. D. Punyapreddiwar, S. P. Zodape, A. V. Wankhade and U. R. Pratap, J. Mol. Catal. B: Enzym., 2016, 133, 124–126 CrossRef CAS
.
- T. Guo, R. Xia, T. Liu, F. Peng, X. Tang, Q. Zhou, H. Luo and W. Xue, Chem. Biodiversity, 2020, 17, e2000025 CAS
.
- Y. Liu, X. Wang, X. Wang and W. He, Org. Biomol. Chem., 2014, 12, 3163–3166 RSC
.
- M. A. Shalaby, A. M. Fahim and S. A. Rizk, RSC Adv., 2023, 13, 14580–14593 RSC
.
- F. Cardona, J. Rocha, A. M. Silva and S. Guieu, Dyes Pigm., 2014, 111, 16–20 CrossRef CAS
.
- K. Chanawanno, A. Hasheminasab, J. T. Engle and C. Ziegler, Polyhedron, 2015, 101, 276–281 CrossRef CAS
.
- P. Suresh and K. Pitchumani, Tetrahedron: Asymmetry, 2008, 19, 2037–2044 CrossRef CAS
.
- J. Wang, H. Li, L. Zu and W. Wang, Adv. Synth. Catal., 2006, 348, 425–428 CrossRef CAS
.
- H.-Y. Xie, S.-R. Ban, J.-N. Liu and Q.-S. Li, Tetrahedron Lett., 2012, 53, 3865–3868 CrossRef CAS
.
- T. P. Kumar, M. A. Sattar and V. U. M. Sarma, Tetrahedron: Asymmetry, 2013, 24, 1615–1619 CrossRef
.
- Y. A. Malik, T. A. Awad, M. Abdalla, S. Yagi, H. A. Alhazmi, W. Ahsan, M. Albratty, A. Najmi, S. Muhammad and A. Khalid, Molecules, 2022, 27, 3181 CrossRef CAS PubMed
.
- J. Skarżewski, M. Zielińska-Błajet and I. Turowska-Tyrk, Tetrahedron: Asymmetry, 2001, 12, 1923–1928 CrossRef
.
- M. Moccia, F. Fini, M. Scagnetti and M. F. Adamo, Angew. Chem., 2011, 123, 7025–7027 CrossRef
.
- B. Sreedhar, M. A. Reddy and P. S. Reddy, Synlett, 2008, 1949–1952 CrossRef CAS
.
- M. B. Gürdere, H. Gezegen, Y. Budak and M. Ceylan, Phosphorus, Sulfur Silicon Relat. Elem., 2012, 187, 889–898 CrossRef
.
- M. G. Vinogradov, O. V. Turova and S. G. Zlotin, Org. Biomol. Chem., 2019, 17, 3670–3708 RSC
.
- S. Mastachi-Loza, T. I. Ramírez-Candelero, L. J. Benítez-Puebla, A. Fuentes-Benítes, C. González-Romero and M. A. Vázquez, Chem. – Asian J., 2022, 17, e202200706 CrossRef CAS PubMed
.
- S. A. Abdel-Latef, A. S. Darwish, S. A. Rizk, S. K. Atya and M. H. E. Helal, J. Mol. Liq., 2019, 288, 111006 CrossRef CAS
.
- M. M. Kaddah, A. A. Fahmi, M. M. Kamel, S. K. Ramadan and S. A. Rizk, Synth. Commun., 2021, 51, 1798–1813 CrossRef CAS
.
- Z.-B. Zhao, X. Li, M.-W. Chen, B. Wu and Y.-G. Zhou, Chin. J. Chem., 2020, 38, 1691–1695 CrossRef CAS
.
-
W.-Q. Chen and J.-A. Ma, Comprehensive Organic Synthesis II, 2014, pp. 1–85 Search PubMed
.
- T. Deng, H. Wang and C. Cai, New J. Chem., 2015, 39, 102–105 RSC
.
- Y. N. Nayak, S. L. Gaonkar and M. Sabu, J. Heterocycl. Chem., 2022 DOI:10.1002/jhet.4617
.
- S. A. Rizk and S. Shaban, J. Heterocycl. Chem., 2019, 56, 2379–2388 CrossRef CAS
.
- F. El-Taib Heakal, S. K. Attia, S. A. Rizk, M. A. Abou Essa and A. E. Elkholy, J. Mol. Struct., 2017, 1147, 714–724 CrossRef CAS
.
- A. Ansari, A. Ali and M. Asif, New J. Chem., 2017, 41, 16–41 RSC
.
- A. Shaik, R. R. Bhandare, K. Palleapati, S. Nissankararao, V. Kancharlapalli and S. Shaik, Molecules, 2020, 25, 1047 CrossRef CAS PubMed
.
- S. A. Rizk and S. R. Atta-Allah, J. Iran. Chem. Soc., 2021, 18, 2407–2423 CrossRef CAS
.
- X.-C. Gao, H. Cao, L.-Q. Zhang, B.-W. Zhang, Y. Cao and C.-H. Huang, J. Mater. Chem., 1999, 9, 1077–1080 RSC
.
- I. V. Taydakov, A. A. Akkuzina, R. I. Avetisov, A. V. Khomyakov, R. R. Saifutyarov and I. C. Avetissov, J. Lumin., 2016, 177, 31–39 CrossRef CAS
.
- M. J. Mayoral, P. Ovejero, J. A. Campo, J. V. Heras, M. R. Torres, C. Lodeiro and M. Cano, New J. Chem., 2010, 34, 2766–2776 RSC
.
- A. Cetin, B. Gündüz, N. Menges and I. Bildirici, Polym. Bull., 2017, 74, 2593–2604 CrossRef CAS
.
- K. Karthikeyan, T. V. Seelan, K. G. Lalitha and P. T. Perumal, Bioorg. Med. Chem. Lett., 2009, 19, 3370–3373 CrossRef CAS PubMed
.
- E. Rajanarendar, S. R. Krishna, D. Nagaraju, K. G. Reddy, B. Kishore and Y. N. Reddy, Bioorg. Med. Chem. Lett., 2015, 25, 1630–1634 CrossRef CAS PubMed
.
- R. M. Kumbhare, U. B. Kosurkar, M. J. Ramaiah, T. L. Dadmal, S. N. C. V. L. Pushpavalli and M. Pal-Bhadra, Bioorg. Med. Chem. Lett., 2012, 22, 5424–5427 CrossRef CAS PubMed
.
- G. Kunig, B. Niedermeyer, J. Deckert, W. Gsell, G. Ransmayr and P. Riederer, Epilepsy Res., 1998, 31, 153–157 CrossRef CAS PubMed
.
- S. S. Basha, K. Divya, A. Padmaja and V. Padmavathi, Res. Chem. Intermed., 2015, 41, 10067–10083 CrossRef CAS
.
- N. Ye, Y. Zhu, H. Chen, Z. Liu, F. C. Mei, C. Wild, H. Chen, X. Cheng and J. Zhou, J. Med. Chem., 2015, 58, 6033–6047 CrossRef CAS PubMed
.
- A. Mamouni, P. Schmitt, M. Mansour and M. Schiavon, Pestic. Sci., 1992, 35, 13–20 CrossRef CAS
.
- Z. Zhang, Y.-J. Tan, C.-S. Wang and H.-H. Wu, Heterocycles, 2014, 89, 1–10 CrossRef
.
- A. Bhatt, R. K. Singh and R. Kant, Synth. Commun., 2019, 49, 1083–1091 CrossRef CAS
.
- N. Nair, J. Majeed, R. Sweety and R. Thakur, Indian J. Pharm. Sci., 2022, 84, 14–26 CAS
.
- M. N. Yousif, W. A. El-Sayed, H.-A. S. Abbas, H. M. Awad and N. M. Yousif, J. Appl. Pharm. Sci., 2017, 7, 021–032 CAS
.
- H. N. Hafez, H. A. Hussein and A.-R. B. El-Gazzar, Eur. J. Med. Chem., 2010, 45, 4026–4034 CrossRef CAS PubMed
.
- H. A. Stefani, C. B. Oliveira, R. B. Almeida, C. M. Pereira, R. C. Braga, R. Cella, V. C. Borges, L. Savegnago and C. W. Nogueira, Eur. J. Med. Chem., 2006, 41, 513–518 CrossRef CAS PubMed
.
- H. Kikuchi, K. Yamamoto, S. Horoiwa, S. Hirai, R. Kasahara, N. Hariguchi, M. Matsumoto and Y. Oshima, J. Med. Chem., 2006, 49, 4698–4706 CrossRef CAS PubMed
.
- B. Singh, A. Maheshwari, G. Dak, K. Sharma and G. Talesara, Indian J. Pharm. Sci., 2010, 72, 607 CrossRef CAS PubMed
.
- S. El-Bahaie, A. Kadry, M. Assy and Y. Ibrahim, Die Pharmazie, 1988, 43, 537–538 CAS
.
- M. R. Prasad, J. Prashanth, K. Shilpa and D. P. Kishore, Chem. Pharm. Bull., 2007, 55, 557–560 CrossRef CAS PubMed
.
- H. M. Aly, N. M. Saleh and H. A. Elhady, Eur. J. Med. Chem., 2011, 46, 4566–4572 CrossRef CAS PubMed
.
- S. Maddila, S. Gorle, N. Seshadri, P. Lavanya and S. B. Jonnalagadda, Arabian J. Chem., 2016, 9, 681–687 CrossRef CAS
.
- V. Alagarsamy, S. Meena, K. Ramseshu, V. Solomon, K. Thirumurugan, K. Dhanabal and M. Murugan, Eur. J. Med. Chem., 2006, 41, 1293–1300 CrossRef CAS PubMed
.
- R. K. Russell, J. B. Press, R. A. Rampulla, J. J. McNally, R. Falotico, J. A. Keiser, D. A. Bright and A. Tobia, J. Med. Chem., 1988, 31, 1786–1793 CrossRef CAS PubMed
.
- M. Murwih Alidmat, M. Khairuddean, N. Mohammad Norman, A. N. Mohamed Asri, M. H. Mohd Suhaimi and G. Sharma, Arabian J. Chem., 2021, 14, 103304 CrossRef CAS
.
- A. Ahmad, M. Y. Wani, M. Patel, A. J. F. N. Sobral, A. G. Duse, F. M. Aqlan and A. S. Al-Bogami, MedChemComm, 2017, 8, 2195–2207 RSC
.
- M. Jann, W. K. Kennedy and G. Lopez, J. Pharm. Pract., 2014, 27, 5–16 CrossRef PubMed
.
- N. Hartley and C. S. McLachlan, Molecules, 2022, 27, 2414 CrossRef CAS PubMed
.
- A. S. Bhathiwal, A. Bendi and A. Tiwari, J. Mol. Struct., 2022, 132649 CrossRef CAS
.
- K. N. M. Halim, S. K. Ramadan, S. A. Rizk and M. A. El-Hashash, Synth. Commun., 2020, 50, 1159–1175 CrossRef CAS
.
- S. Kumar, S. L. Khokra and A. Yadav, Future J. Pharm. Sci., 2021, 7, 106 CrossRef PubMed
.
- N. Singh, S. Pandey and R. P. Tripathi, Carbohydr. Res., 2010, 345, 1641–1648 CrossRef CAS PubMed
.
- Y. Zhang, X. Li, J. Li, J. Chen, X. Meng, M. Zhao and B. Chen, Org. Lett., 2012, 14, 26–29 CrossRef CAS PubMed
.
- C. Zhang and W. Li, Bioorg. Med. Chem. Lett., 2013, 23, 5002–5005 CrossRef PubMed
.
- H. M. T. Albuquerque, C. M. M. Santos, J. A. S. Cavaleiro and A. M. S. Silva, Curr. Org. Chem., 2014, 18, 2750–2775 CrossRef CAS
.
- X. He, S. E. Kassab, G. Heinzl and F. Xue, Tetrahedron Lett., 2015, 56, 1034–1037 CrossRef CAS
.
- S. A. Rizk, A. M. El-Naggar and A. A. El-Badawy, J. Mol. Struct., 2018, 1155, 720–733 CrossRef CAS
.
- S. Farooq and Z. Ngaini, Chem. Afr., 2020, 3, 291–302 CrossRef CAS
.
- R. Umeda, M. Muraki, Y. Nakamura, T. Tanaka, K. Kamiguchi and Y. Nishiyama, Tetrahedron Lett., 2017, 58, 2393–2395 CrossRef CAS
.
- Y. Zhu, Q. Wang, R. G. Cornwall and Y. Shi, Chem. Rev., 2014, 114, 8199–8256 CrossRef CAS PubMed
.
- S. Arai, H. Tsuge and T. Shioiri, Tetrahedron Lett., 1998, 39, 7563–7566 CrossRef CAS
.
- S. Tanaka and K. Nagasawa, Synlett, 2009, 667–670 CAS
.
- N. Ji, Q. Tian, Q. Yang, M. Li and W. He, Tetrahedron Lett., 2021, 68, 152909 CrossRef CAS
.
- E. Miller, P. J. Moon and F. D. Toste, Catal. Asymmetric Synth., 2022, 117–156 Search PubMed
.
- G. Fioroni, F. Fringuelli, F. Pizzo and L. Vaccaro, Green Chem., 2003, 5, 425–428 RSC
.
- D. Y. Kim, Y. J. Choi, H. Y. Park, C. U. Joung, K. O. Koh, J. Y. Mang and K.-Y. Jung, Synth. Commun., 2003, 33, 435–443 CrossRef CAS
.
- V. K. Reddy, B. Haritha and M. Yamashita, Lett. Org. Chem., 2005, 2, 128–131 CrossRef CAS
.
- M. B. Andrus and B. W. Poehlein, Tetrahedron Lett., 2000, 41, 1013–1014 CrossRef CAS
.
- X.-L. Geng, Z. Wang, X.-Q. Li and C. Zhang, J. Org. Chem., 2005, 70, 9610–9613 CrossRef CAS PubMed
.
- J. V. Allen, K.-H. Drauz, R. W. Flood, S. M. Roberts and J. Skidmore, Tetrahedron Lett., 1999, 40, 5417–5420 CrossRef CAS
.
- N. Hashimoto and A. Kanda, Org. Process Res. Dev., 2002, 6, 405–406 CrossRef CAS
.
- K. Jakka, J. Liu and C.-G. Zhao, Tetrahedron Lett., 2007, 48, 1395–1398 CrossRef CAS PubMed
.
- J. Ye, Y. Wang, J. Chen and X. Liang, Adv. Synth. Catal., 2004, 346, 691–696 CrossRef CAS
.
- A. L. Baumstark and D. B. Harden Jr., J. Org. Chem., 1993, 58, 7615–7618 CrossRef CAS
.
- H. Jin, H. Zhao, F. Zhao, S. Li, W. Liu, G. Zhou, K. Tao and T. Hou, Ultrason. Sonochem., 2009, 16, 304–307 CrossRef CAS PubMed
.
- C. J. Adams and L. Main, Tetrahedron, 1991, 47, 4959–4978 CrossRef CAS
.
- R. Kamal, R. Kumar, V. Kumar and V. Bhardwaj, ChemistrySelect, 2019, 4, 11578–11603 CrossRef CAS
.
- K. Jakhar and J. Makrandi, Indian J. Chem., 2013, 52B, 141–145 CAS
.
- J. Nath and M. K. Chaudhuri, Green Chem. Lett. Rev., 2008, 1, 223–230 CrossRef CAS
.
- A. Jitareanu, A.-M. Zbancioc, C. Tuchilus, M. Balan, U. Stanescu and G. Tataringa, Med. Surg. J., 2015, 119, 1180–1188 Search PubMed
.
- E. Hadjoudis, Mol. Cryst. Liq. Cryst., 1986, 134, 237–244 CrossRef CAS
.
- K. Tanaka, R. Shiraishi and F. Toda, J. Chem. Soc., Perkin Trans. 1, 1999, 3069–3070 RSC
.
- S.-Y. Luo, J.-Y. Zhu, M.-F. Zou, S. Yin and G.-H. Tang, Nat. Prod. Bioprospect., 2022, 12, 31 CrossRef CAS PubMed
.
- H. Cong, C. F. Becker, S. J. Elliott, M. W. Grinstaff and J. A. Porco Jr., J. Am. Chem. Soc., 2010, 132, 7514–7518 CrossRef CAS PubMed
.
- Y. Hu, S. Li, Z. Wang, Y. Yao, T. Li, C. Yu and C. Yao, J. Org. Chem., 2018, 83, 3361–3366 CrossRef CAS PubMed
.
- M. Ibrahim-Ouali and F. Dumur, Eur. Polym. J., 2021, 158, 110688 CrossRef CAS
.
- M.-A. Tehfe, F. Dumur, P. Xiao, M. Delgove, B. Graff, J.-P. Fouassier, D. Gigmes and J. Lalevée, Polym. Chem., 2014, 5, 382–390 RSC
.
- P. Mahesha, N. S. Shetty and S. D. Kulkarni, J. Fluoresc., 2022, 32, 835–862 CrossRef CAS PubMed
.
- A. Gupta, S. Garg and H. Singh, Anal. Methods, 2020, 12, 5022–5045 RSC
.
- B. Zhou, P. Jiang, J. Lu and C. Xing, Arch. Pharm., 2016, 349, 539–552 CrossRef CAS PubMed
.
- Y. Sun, H. Chen, D. Cao, Z. Liu, H. Chen, Y. Deng and Q. Fang, J. Photochem. Photobiol., A, 2012, 244, 65–70 CrossRef CAS
.
- Y. Shan, Z. Liu, D. Cao, Y. Sun, K. Wang and H. Chen, Sens. Actuators, B, 2014, 198, 15–19 CrossRef CAS
.
- Z. Song, R. T. Kwok, E. Zhao, Z. He, Y. Hong, J. W. Lam, B. Liu and B. Z. Tang, ACS Appl. Mater. Interfaces, 2014, 6, 17245–17254 CrossRef CAS PubMed
.
- Y. Du, F. Liang, M. Hu, R. Bu, M. Wang, A. Tsuda and C. Eerdun, RSC Adv., 2020, 10, 37463–37472 RSC
.
- A. Demirbas, Appl. Energy, 2009, 86, S108–S117 CrossRef CAS
.
-
P. Grammelis, Solid biofuels for energy, Springer, 2010 Search PubMed
.
-
A. Dufey, Biofuels production, trade and sustainable development: emerging issues, Iied, 2006 Search PubMed
.
- Z. Yaakob, B. N. Narayanan, S. Padikkaparambil, S. Unni K. and M. Akbar P., Renewable Sustainable Energy Rev., 2014, 35, 136–153 CrossRef CAS
.
- V. Chandrasekaran, M. Arthanarisamy, P. Nachiappan, S. Dhanakotti and B. Moorthy, J. Transp. Res. D: Transp. Environ., 2016, 46, 145–156 CrossRef
.
- K. Fangsuwannarak, P. Wanriko and T. Fangsuwannarak, Energy Procedia, 2016, 100, 227–236 CrossRef CAS
.
- R. Bharti and B. Singh, Fuel, 2020, 262, 116658 CrossRef CAS
.
- C. A. Moreira, E. C. Faria, J. E. Queiroz, V. S. Duarte, M. D. N. Gomes, A. M. da Silva, R. L. G. de Paula, C. H. Franco, E. H. D. S. Cavalcanti and G. L. de Aquino, Fuel Process. Technol., 2022, 227, 107122 CrossRef CAS
.
-
C. C. Da Silva, B. S. Pacheco, S. C. de Freitas, L. M. Berneira, M. A. Z. dos Santos, L. Pizzuti and C. M. P. de Pereira, in Increased Biodiesel Efficiency: Alternatives for Production, Stabilization, Characterization and Use of Coproduct, ed. M. Trindade, Springer International Publishing, Cham, 2018, pp. 81–110 Search PubMed
.
- A. Saha, Mater. Today: Proc., 2022, 64, 605–610 CAS
.
- A. J. Kiran, H. Kim, K. Kim, F. Rotermund, H. Ravindra, S. Dharmaprakash and H. Lim, Appl. Phys. Lett., 2008, 92, 103 CrossRef
.
- D. Haleshappa, A. Jayarama, R. Bairy, S. Acharya and P. S. Patil, Phys. B, 2019, 555, 125–132 CrossRef CAS
.
- S. K. Alsaee, M. A. A. Bakar, D. A. Zainuri, A. H. Anizaim, M. F. Zaini, M. M. Rosli, M. Abdullah, S. Arshad and I. A. Razak, Opt. Mater., 2022, 128, 112314 CrossRef CAS
.
- H. Kumari, S. E. Armitage, S. R. Kline, K. K. Damodaran, S. R. Kennedy, J. L. Atwood and J. W. Steed, Soft Matter, 2015, 11, 8471–8478 RSC
.
- L. Cruz, N. Basílio, N. Mateus, V. De Freitas and F. Pina, Chem. Rev., 2021, 122, 1416–1481 CrossRef PubMed
.
- V. Petrov, S. Slavcheva, S. Stanimirov and F. Pina, J. Phys. Chem. A, 2015, 119, 2908–2918 CrossRef CAS PubMed
.
- A. M. Fahim, J. Mol. Struct., 2023, 1277, 134871 CrossRef CAS
.
- G. H. Elsayed, S. Dacrory and A. M. Fahim, Int. J. Biol. Macromol., 2022, 222, 3077–3099 CrossRef CAS PubMed
.
- M. N. Uddin, S. S. Ahmed, M. Uzzaman, M. N. H. Knock, W. Shumi, A. F. M. Sanaullah and M. M. H. Bhuyain, Results Chem., 2022, 4, 100329 CrossRef PubMed
.
- J.-Y. Park, J.-A. Ko, D. W. Kim, Y. M. Kim, H.-J. Kwon, H. J. Jeong, C. Y. Kim, K. H. Park, W. S. Lee and Y. B. Ryu, J. Enzyme Inhib. Med. Chem., 2016, 31, 23–30 CrossRef CAS PubMed
.
- S. Jo, H. Kim, S. Kim, D. H. Shin and M. S. Kim, Chem. Biol. Drug Des., 2019, 94, 2023–2030 CrossRef CAS PubMed
.
- J.-H. Wu, X.-H. Wang, Y.-H. Yi and K.-H. Lee, Bioorg. Med. Chem. Lett., 2003, 13, 1813–1815 CrossRef CAS PubMed
.
- M. Xu, P. Wu, F. Shen, J. Ji and K. Rakesh, Bioorg. Chem., 2019, 91, 103133 CrossRef CAS PubMed
.
- A. L. Cole, S. Hossain, A. M. Cole and O. Phanstiel IV, Bioorg. Med. Chem., 2016, 24, 2768–2776 CrossRef CAS PubMed
.
- A. S. Chintakrindi, E. A.F Martis, D. J. Gohil, S. T. Kothari, A. S. Chowdhary, E. C. Coutinho and M. A. Kanyalkar, Curr. Comput.–Aided Drug Des., 2016, 12, 272–281 CrossRef CAS PubMed
.
- K. Malbari, H. Gonsalves, A. Chintakrindi, D. Gohil, M. Joshi, S. Kothari, S. Srivastava, A. Chowdhary and M. Kanyalkar, Acta Virol., 2018, 62, 179–190 CrossRef CAS PubMed
.
- P. H. Nguyen, M. Na, T. T. Dao, D. T. Ndinteh, J. T. Mbafor, J. Park, H. Cheong and W. K. Oh, Bioorg. Med. Chem. Lett., 2010, 20, 6430–6434 CrossRef CAS PubMed
.
- G. C. Brandão, E. G. Kroon, M. G. R. Duarte, F. C. Braga, J. D. de Souza Filho and A. B. de Oliveira, J. Phytomed., 2010, 17, 926–929 CrossRef PubMed
.
- C. H. Heh, R. Othman, M. J. Buckle, Y. Sharifuddin, R. Yusof and N. A. Rahman, Chem. Biol. Drug Des., 2013, 82, 1–11 CrossRef CAS PubMed
.
- H. Ishitsuka, Y. Ninomiya, C. Ohsawa, M. Fujiu and Y. Suhara, Antimicrob. Agents Chemother., 1982, 22, 617–621 CrossRef CAS PubMed
.
- Y. Ninomiya, N. Shimma and H. Ishitsuka, Antiviral Res., 1990, 13, 61–74 CrossRef CAS PubMed
.
- D. Elkhalifa, I. Al-Hashimi, A.-E. Al Moustafa and A. Khalil, J. Drug Targeting, 2021, 29, 403–419 CrossRef CAS PubMed
.
- M. Adianti, C. Aoki, M. Komoto, L. Deng, I. Shoji, T. S. Wahyuni, M. I. Lusida, H. Fuchino, N. Kawahara and H. Hotta, Microbiol. Immunol., 2014, 58, 180–187 CrossRef CAS PubMed
.
- M. Yang, N. Li, F. Li, Q. Zhu, X. Liu, Q. Han, Y. Wang, Y. Chen, X. Zeng and Y. Lv, Int. Immunopharmacol., 2013, 16, 466–474 CrossRef CAS PubMed
.
-
J. Harizal and Y. S. Kurniawan, Beauty-Cosmetic science, cultural issues and creative developments [Internet].London: IntechOpen, 2020 Search PubMed
.
- P. Boeck, C. A. B. Falcão, P. C. Leal, R. A. Yunes, V. Cechinel Filho, E. C. Torres-Santos and B. Rossi-Bergmann, Bioorg. Med. Chem., 2006, 14, 1538–1545 CrossRef CAS PubMed
.
- K. Sugamoto, Y.-I. Matsusita, K. Matsui, C. Kurogi and T. Matsui, Tetrahedron, 2011, 67, 5346–5359 CrossRef CAS
.
- W. Liu, M. He, Y. Li, Z. Peng and G. Wang, J. Enzyme Inhib. Med. Chem., 2022, 37, 9–38 CrossRef CAS PubMed
.
- H. Aryapour, G. H. Riazi, A. Foroumadi, S. Ahmadian, A. Shafiee, O. Karima, M. Mahdavi, S. Emami, M. Sorkhi and S. Khodadady, Med. Chem. Res., 2011, 20, 503–510 CrossRef CAS
.
- S. Burmaoglu, D. Aktas Anil, A. Gobek, D. Kilic, D. Yetkin, N. Duran and O. Algul, J. Biomol. Struct. Dyn., 2022, 40, 3525–3550 CrossRef CAS PubMed
.
- S. Y. Shin, J.-H. Kim, H. Yoon, Y.-K. Choi, D. Koh, Y. Lim and Y. H. Lee, J. Agric. Food Chem., 2013, 61, 12588–12597 CrossRef CAS PubMed
.
- V. Ramkumar, P. Kamachiyappan, R. Manjunath, S. Balamurugan, A. Ashokkumar, M. K. Ashraf Ali and S. C. Kim, Opt. Mater., 2022, 123, 111881 CrossRef CAS
.
- A. M. Fahim and M. A. Shalaby, J. Mol. Struct., 2019, 1176, 408–421 CrossRef CAS
.
|
This journal is © The Royal Society of Chemistry 2023 |
Click here to see how this site uses Cookies. View our privacy policy here.