Water-soluble copper pyrithione complexes with cytotoxic and antibacterial activity†
Received
8th July 2022
, Accepted 20th December 2022
First published on 1st March 2023
Abstract
Copper Pyrithione, [Cu(PyS)2] has shown excellent biological activity against cancer cells and bacterial cells, however, it has extremely low aqueous solubility, limiting its applicability. Herein, we report a series of PEG-substituted pyrithione copper(II) complexes with significantly increased aqueous solubility. While long PEG chains lead to a decrease in bioactivity, the addition of short PEG chains leads to improved aqueous solubility with retention of activity. One novel complex, [Cu(PyS1)2], has particularly impressive anticancer activity, surpassing that of the parent complex.
Introduction
Copper plays pivotal roles in cellular physiology, typically by participating as a catalytic cofactor in enzymes that carry out electron transfer such as cytochrome-c-oxidase and Cu, Zn- superoxide dismutase.1,2 However, an excess of copper is toxic to cells, since this metal ion can catalyse side electron transfer reactions, generating reactive oxygen species (ROS) and promoting oxidative damage to DNA, proteins and lipids.3,4 An excess of copper can also bind adventitiously to proteins and biomolecules, and inhibit their functions.5 The amount and availability of copper in cells needs to be tightly regulated, since too little copper will lead to cellular starvation while too much copper will lead to cellular poisioning.6 This concept can be utilised in controlling growth and proliferation of cells to combat diseases. For example, increasing copper levels in the cellular environment by dosing with copper-releasing complexes can lead to destruction of cancer cells and disruption of bacterial cell growth.7
The poor cell-permeability of simple copper salts (e.g., CuCl2)8 has led to the development of small, lipophilic copper carrier molecules that increase the permeability of the copper ion. Disulfiram (DSF) and diethyldithiocarbamate (DDC) are two early examples of ligands that bind copper(II), forming the same complex that exhibits a broad range of activity against various cancer cell lines (Fig. 1A).9,10 Moreover, this complex has also been observed to be a potent antibacterial agent, particularly against an antibiotic-resistant strain of Staphylococcus aureus (S. aureus).11 Various other Schiff-base and phenanthroline based ligands which form complexes with copper(II) have also been developed as antibacterial agents.12,13 In other recent studies, various phenanthroline and bipyridine based copper(II) complexes have been developed by Suntharalingam et al. to treat breast cancer cells with sub-micromolar potency (Fig. 1C and D).14,15
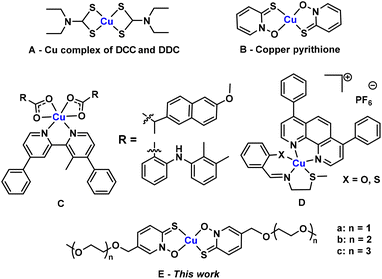 |
| Fig. 1 Structure of copper complexes as potential bioactive drugs. | |
Pyrithione (PyS) is another well-known bidentate chelator, widely used as an active ingredient in personal care products such as shampoo and also as an antifouling agent upon formation of square planar complexes with zinc(II) or copper(II) ions (Fig. 1B).16 Pyrithione is also a known ionophore, shown to aid metal ion transport across cell membranes.17 However, utilisation of this ligand has not yet been popularised in clinical medicine. In addition to its direct antibacterial activity, [Cu(PyS)2] also displays synergistic effects with β-lactam antibiotics.8 Here, it is thought that [Cu(PyS)2] delivers copper(II) ions into the bacterial cells, which inactivate bacterial metallo-β-lactamases, leading to restoration of bacterial susceptibility to β-lactam antibiotics.8 Furthermore, [Cu(PyS)2] has recently been found to show potency towards various cancer cells lines.18 In addition, pyrithione complexes of ruthenium(II) have shown anticancer activity.19 While some organometallic pyrithione complexes have been shown to have reasonable aqueous solubility,20 one limitation to the use of [Cu(PyS)2] in clinical medicine is the poor water solubility (0.001 g l−1), reducing its bioavailability.21 Herein, we address this limitation by synthesising polyethylene glycol (PEG) substituted pyrithione ligands and their copper(II) complexes in order to modulate solubility and lipophilicity, while maintaining or improving the biological activity (Fig. 1E). Specifically, we hypothesised that introduction of short PEG chains on pyrithione will help to achieve balanced physical and chemical properties for biological activity.
Results and discussion
Synthesis and characterisation of polyethylene glycol substituted pyrithione copper(II) complexes
In order to increase the solubility of copper pyrithione complexes, we introduced amphiphilic substituents onto the pyrithione ligand. Three water-soluble pyrithione ligands with different lengths of polyethylene glycol (PEG) chains were synthesised using adapted literature procedures (Scheme 1).22,23 The general procedure started with bromination of commercially available 2-bromo-5-bromomethylpyridine using N-bromosuccinimide and dibenzoyl peroxide. The brominated product (1) was then reacted with the respective alcohol-terminated PEG chains and further oxidation and nucleophilic aromatic sulfur substitution were performed to achieve substituted pyrithione ligands (4a–c). Formation of intermediates and the final ligands were confirmed and analysed using high field multinuclear NMR spectroscopy and ESI mass spectrometry. Complexation of these ligands with copper was carried out by reacting the respective bidentate ligand with copper(II) chloride in a 2
:
1 ratio using water as the solvent, leading to precipitation of the desired complexes. Mass spectrometry confirmed the formation of the metal–ligand complexes and elemental analysis (% C, H and N) and HPLC data confirmed purity of the products.
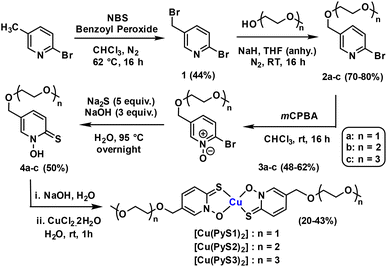 |
| Scheme 1 Synthetic route for novel copper complexes. | |
Single crystals for the copper complex with the shortest PEG-chain [Cu(PyS1)2] were grown from slow-evaporation of dichloromethane-methanol solvent-system. The X-ray crystallography data reveals a P
space group and formation of the square planar complex in both cis and trans isomer forms together as co-crystals in a unit cell (Fig. 2). This behaviour contrasts the parent [Cu(PyS)2] complex, where only the trans isomer is observed in the published X-ray structures.24 The crystal packing of [Cu(PyS1)2] (see ESI†) shows stacking of alike isomers, with adjacent cis isomer displaying intermolecular Cu–O and Cu–S interactions (bond distance = 3.5 Å and 3.6 Å, respectively) and adjacent trans isomers showing intermolecular Cu–S interactions of 3.5 Å. Compared to the parent complex, which forms highly ordered stacked layers of complexes, the crystal packing of [Cu(PyS1)2] shows fewer intermolecular interactions, which may contribute to the higher aqueous solubility of the complex (vide infra).
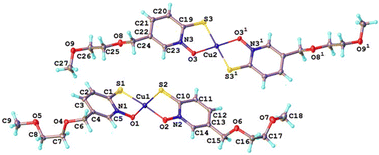 |
| Fig. 2 A 1 : 1 mixture of cis and trans isomers in the unit cell of [Cu(PyS1)2] as determined by single crystal X-ray diffraction. | |
Solubility and lipophilicity of copper(II) complexes
Water-solubility of the PEG-substituted complexes was calculated by measuring absorbance of saturated solutions in 1% DMSO/water using UV-vis spectroscopy (Table 1). As expected, solubility increases with increasing PEG chain length. [Cu(PyS1)2] was found to be the least soluble of the new complexes (limiting solubility = 0.32 mM) but still showed more than 100 times greater solubility than the parent complex [Cu(PyS)2], which has solubility of around 0.003 mM (0.001 g l−1). As the PEG chain length increased further, solubility increased with values of 1.2 mM for [Cu(PyS2)2] and >40 mM for the longest PEG chain complex [Cu(PyS3)2]. In addition, extinction coefficients (ε) were calculated in octanol and water for each PEGylated copper complex (Table 1) by measuring absorption spectra at varying complex concentration (Fig. 3 and ESI†).
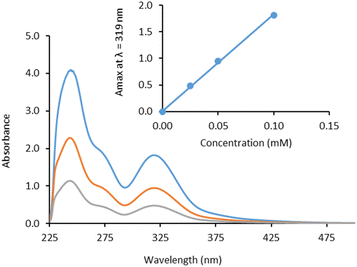 |
| Fig. 3 UV absorbance spectra of [Cu(PyS1)2] in water at 25 °C at 0.03 (grey) 0.05 (orange) and 0.10 (blue) mM and (insert) absorbance at 319 nm at varying concentration allowing calculation of extinction coefficient. | |
Table 1 Limiting solubility, extinction coefficient (ε) and log
P values for PEG-chain substituted copper pyrithione complexes
Complex |
Solubility (mM) |
ε
octanol (M−1 cm−1) |
ε
water (M−1 cm−1) |
Log P |
Log P values measured by ICP-OES.
Log P values measured by UV/Vis.
|
[Cu(PyS)2] |
0.003 |
— |
— |
2.337a (–)b |
[Cu(PyS1)2] |
0.32 |
20 000 |
19 800 |
1.812a (1.484)b |
[Cu(PyS2)2] |
1.2 |
18 900 |
18 200 |
1.744a (1.423)b |
[Cu(PyS3)2] |
>40 |
18 600 |
17 100 |
1.391a (1.217)b |
Lipophilicity affects the ability of a drug molecule to permeate through the lipid bilayer of cell membranes. Regulating lipophilicity of a potential drug molecule is of great importance to the pharmaceutical industry to improve bioavailability and cell uptake. Lipophilicity is usually represented as partition coefficient log
P, where P describes the equilibrium concentrations of the compound dissolved into a two-phase system of n-octanol and water (eqn (1)).
| 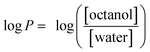 | (1) |
Conventionally, lipophilicity is measured using a shake-flask method where UV/vis-spectroscopy is used to quantify compound in the organic and aqueous solvent layers. Initially, we used this method to measure log
P values for the three new complexes (Table 1). Due to the low solubility of the parent complex [Cu(PyS)2] in water, we were unable to measure an extinction coefficient, which is required to calculate [Cu(PyS)2] concentration in the lipophilicity study. Instead, we resorted to ICP-OES as a technique to quantify the Cu levels present in both octanol and aqueous layers. From ICP-OES analysis, the order of lipophilicity from highest to lowest is [Cu(PyS)2] > [Cu(PyS1)2] > [Cu(PyS2)2] > [Cu(PyS3)2]. This trend is echoed using analysis by UV-vis and reveals that adding PEG-chains to the copper(II) pyrithione complex leads to a reduction in lipophilicity.
In vitro human cell line cytotoxicity data
Metal complexes have been long been explored for their potential anticancer activity.25–27 For the novel copper complexes, antiproliferation studies were carried out against MIA PaCa-2 pancreatic carcinoma cells, 143B bone osteosarcoma cells and normal ARPE-19 retinal epithelial cells, using MTT assay over the course of 24 h. [Cu(PyS)2] and the three novel copper(II) complexes were screened alongside the known anticancer drug cisplatin and CuCl2 as a reference. The inhibitory concentration (IC50) values of the three PEG-substituted complexes are in the sub-micromolar range and are comparable with that of the parent [Cu(PyS)2] complex (Table 2). This result indicates that chemical modification of the pyrithione ligand in order to improve solubility does not have a detrimental effect on the activity of the complex. However, the shortest PEG-chain complex [Cu(PyS1)2] displayed slightly higher activity than [Cu(PyS)2] against MIA PaCa-2 (Table 2). As the PEG-chain length increases, activity decreases against both pancreatic and bone cancer cell lines, indicating a trend that lowering lipophilicity can decrease activity. By comparing activity against the normal ARPE-19 cell line, it can be seen that copper complexes show little selectivity against cancer cells, although the most selective complex [Cu(PyS1)2] shows a slight selectivity, with a selectivity index of 1.6 between pancreatic and normal cells. The proliferation data against the MIA PaCa-2 and 143B cancer cell lines show that the IC50 values of the copper(II) pyrithione complexes are more than 50 times lower than that of cisplatin. This impressive activity augurs well for further study into these complexes as anticancer agents.
Table 2 IC50 data (after 24 h incubation, MTT assay) for non-substituted and PEG- chain substituted copper pyrithione complexes along with copper chloride and cisplatin (CDDP)
Complex |
IC50 values (μM ± SD) |
Cell uptakea (ng of Cu/106 cells) |
MIA PaCa-2 |
143B |
ARPE-19 |
Measured cell uptake with the 143B cell line using ICP-OES (see ESI for details†).
|
[Cu(PyS)2] |
0.15 ± 0.04 |
0.20 ± 0.02 |
0.22 ± 0.02 |
16 ± 4 |
[Cu(PyS1)2] |
0.13 ± 0.01 |
0.42 ± 0.04 |
0.20 ± 0.01 |
63 ± 7 |
[Cu(PyS2)2] |
0.22 ± 0.01 |
0.50 ± 0.03 |
0.16 ± 0.01 |
43 ± 5 |
[Cu(PyS3)2] |
0.26 ± 0.01 |
0.86 ± 0.07 |
0.27 ± 0.05 |
38 ± 3 |
CuCl2 |
>100 |
75 ± 3 |
83 ± 2 |
— |
CDDP |
5.7 ± 0.1 |
14 ± 2 |
13.01 ± 0.1 |
— |
Cell uptake of the copper complexes (1 μM for 4 h) into the 143B cells was measured using ICP-OES (ESI for details†). The results (Table 2) show a correlation within the PEGylated complexes between lipophilicity, cell uptake and cytotoxicity, with the more lipophilic [Cu(PyS1)2] showing the highest cell uptake of 63 ± 7 ng of Cu/106 cells. The data show that, within the PEGylated complexes, increased lipophilicity leads to increased cell uptake and a corresponding increase in cytotoxicity. The parent complex [Cu(PyS)2] does not follow this trend, however, and shows the lowest cell uptake of 16 ± 4 ng of Cu/106 cells, despite being more lipophilic than the PEGylated complexes. While the reason for this lower cell uptake is not clear, it is possible that the highly lipophilic [Cu(PyS)2] associates strongly with the cell membrane, reducing the overall cell uptake or potentially the lower solubility of [Cu(PyS)2] leads to aggregation in the cell media hindering cell uptake.
In vitro activity against Gram-positive bacteria
The three novel PEG chain copper(II) complexes, along with non-substituted copper(II) pyrithione, CuCl2, and levofloxacin as controls, were tested against ESKAPE pathogen panel. The lowest MIC values were found against Gram-positive bacterium S. aureus (Table 3), with all copper(II) pyrithione complexes showing low micromolar (μM) activity. By comparison, CuCl2 shows no activity up to 100 μM, highlighting the importance of the ligand for activity. The copper(II) pyrithione complexes follow the same trend as that for anticancer activity; namely, that the increase in PEG chain length leads to lower complex activity.
Table 3 Antibacterial data for PEG chain substituted copper pyrithione complexes against ESKAPE pathogen panel
Complex |
MIC (μM) |
E. coli ATCC 25922 |
S. aureus ATCC 29213 |
K. pneumoniae BAA 1705 |
A. baumannii BAA 1605 |
P. aeruginosa ATCC 27853 |
[Cu(PyS)2] |
6.4 |
0.4 |
12.8 |
12.8 |
>100 |
[Cu(PyS1)2] |
32.5 |
2 |
>100 |
>100 |
>100 |
[Cu(PyS2)2] |
>100 |
3.4 |
>100 |
>100 |
>100 |
[Cu(PyS3)2] |
>100 |
6 |
>100 |
>100 |
>100 |
CuCl2 |
>100 |
>100 |
>100 |
>100 |
>100 |
Levofloxacin |
0.022 |
0.35 |
>100 |
22 |
2.8 |
Among the panel of Gram-negative bacteria, activity of Escherichia coli (E. coli) is most affected by the introduction of PEG substituents. Unsubstituted [Cu(PyS)2] shows some activity with a minimum inhibitory concentration (MIC) value of 6.4 μM. The shortest PEG-chain complex, [Cu(PyS1)2], shows lower activity at 32.5 μM, while the longer chain complexes show almost no activity. The universal lower activity of the copper(II) complexes against Gram-negative bacteria likely relates to their poorer ability to permeate through the cell membranes, as Gram-negative species have an extra layer of lipopolysaccharide membrane which is absent in Gram-positive species.
In vitro synergy study with β-lactam antibiotics
The three novel PEG chain copper complexes, along with non-substituted copper(II) pyrithione were tested against a β-lactam resistant strain of E. coli that produces the New Delhi metallo-β-lactamase 1 (NDM-1). All the copper complexes tested in this study did not display strong direct activity against this strain. The MIC values of the copper complexes alone were 100 μM for [Cu(PyS1)2] and >100 μM for [Cu(PyS2)2] and [Cu(PyS3)2]. However, the copper complexes appeared to synergise with the β-lactam antibiotics, meropenem and ertapenem. In the absence of copper complexes, meropenem and ertapenem display MIC values of 1 μM and 6 μM, respectively (Fig. 4). These values are unaffected by the addition of CuCl2 (up to 100 μM). By contrast, addition of the novel copper pyrithione complexes leads to strong reduction in MIC values for both antibiotics. Addition of the longer chain PEG complexes [Cu(PyS3)2] and [Cu(PyS2)2] reduced the meropenem and ertapenem MIC values to around half of their original value (at 50 μM copper complex). The shorter chain complex [Cu(PyS1)2] leads to the same halving of antibiotic MIC at only 6 μM copper concentration and at 50 μM copper concentration, further reduced the antibiotic's MIC values by 10-fold compared to the value without copper. [Cu(PyS)2] slightly outperforms [Cu(PyS1)2], with a 10-fold reduction in antibiotic MIC at around 25 μM copper concentration. Fractional inhibitory concentration (FIC) index values could not be calculated since the MIC values for most of the copper complexes were beyond the solubility limit >100 μM. Nevertheless, the results clearly indicate that these complexes enhanced the potency of meropenem and ertapenem antibiotics. As hypothesised previously, the copper complexes likely inhibit the NDM-1 metallo-β-lactamase via transmetallation of the zinc(II) enzyme cofactor with copper(II).8
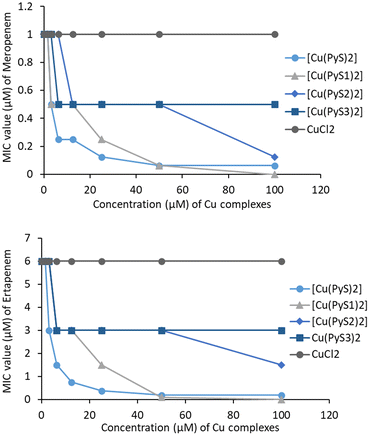 |
| Fig. 4 Data for antibiotic synergy study of non-substituted and PEG chain substituted complexes with meropenem (upper) and ertapenem (lower). | |
Conclusions
In summary, we report the synthesis and characterisation of three novel water soluble pyrithione-based ionophores bearing PEG chain substitutions of varying chain length and their copper(II) complexes. Addition of the PEG chains vastly increases aqueous solubility when compared to the parent complex [Cu(PyS)2]. The longest PEG-chain complex shows >25
000 fold increase in solubility, while even the shortest PEG chain length shows 200 fold improvement over [Cu(PyS)2]. Lipophilicity decreased with increasing PEG-chain length, consistent with the introduction of polar substituents. [Cu(PyS)2] is well known to have interesting biological activity against cancer cells and bacteria. The novel copper(II) complexes exhibit sub-micromolar potency towards pancreatic carcinoma (MIA PaCa-2) and bone osteosarcoma (143B) cell lines, with a correlation between lipophilicity, cell uptake and cytotoxicity, and [Cu(PyS1)2] showing improved activity compared to the parent complex. All copper(II) pyrithione complexes showed far greater anticancer activity than either cisplatin or the inactive CuCl2. We also report the antimicrobial activity of the new complexes against S. aureus class of pathogen. In both anticancer and antimicrobial assays, a trend is observed of lower activity with increasing length of PEG chain. As longer PEG-chain lead to a decrease in lipophilicity, we conclude that activity is correlated with cell uptake, which is directly associated with lipophilicity of the complexes. The most lipophilic complex is the parent [Cu(PyS)2], however, this species has exceptionally low aqueous solubility making it challenging to process in biological assays. The shortest PEG chain complex [Cu(PyS1)2] retains activity, but with vastly increased aqueous solubility. In short, the novel complex, [Cu(PyS1)2], shows the most promise by balancing the increase in solubility with only a small decrease in lipophilicity, ultimately leading to an easily manipulated complex with exceptional biological activity.
Author contributions
Project design by J. W. W., K. Y. D. and A. M. Synthesis and characterisation by A. M. Anticancer assays and cell uptake work by R. M. L. and Y.-H. L. Antimicrobial assays by SC, A. A., G. K. and D. S. Antibiotic synergy studies by K. Y. D. and A. M. Manuscript written by A. M., with edits from all authors.
Conflicts of interest
There are no conflicts to declare.
Acknowledgements
We acknowledge Durham University GCRF CDT for funding. We acknowledge Dmitry S. Yufit for crystallography and Emily R. Unsworth for ICP-OES. We also acknowledge the UKRI Future Leader Fellow scheme which fund the employment of Yi-Hsuan Lee and Rianne M. Lord at the University of East Anglia.
References
- T. Attar, Chem. Rev. Lett., 2020, 3, 117–130 CAS.
- C. Marzano, M. Pellei, F. Tisato and C. Santini, Anticancer Agents Med. Chem., 2012, 9, 185–211 CrossRef PubMed.
- N. Husain and R. Mahmood, Environ. Sci. Pollut. Res., 2019, 26, 20654–20668 CrossRef CAS PubMed.
- Y. Yoshida, S. Furuta and E. Niki, Biochim. Biophys. Acta, 1993, 1210, 81–88 CrossRef CAS PubMed.
- C. Pfeiffer and R. Mailloux, J. Orthomol. Med., 1987, 2, 171–182 Search PubMed.
- D. L. de Romaña, M. Olivares, R. Uauy and M. Araya, J. Trace Elem. Med. Biol., 2011, 25, 3–13 CrossRef PubMed.
- B. E. Kim, T. Nevitt and D. J. Thiele, Nat. Chem. Biol., 2008, 4, 176–185 CrossRef CAS PubMed.
- K. Y. Djoko, M. E. S. Achard, M.-D. Phan, A. W. Lo, M. Miraula, S. Prombhul, S. J. Hancock, K. M. Peters, H. E. Sidjabat, P. N. Harris, N. Mitić, T. R. Walsh, G. J. Anderson, W. M. Shafer, D. L. Paterson, G. Schenk, A. G. Mcewan and A. Schembri, Antimicrob. Agents Chemother., 2018, 62, 1–10 CrossRef CAS PubMed.
- S. Wadhwa and R. J. Mumper, Cancer Lett., 2013, 337, 8–21 CrossRef CAS PubMed.
- Z. Skrott and B. Cvek, Mini-Rev. Med. Chem., 2012, 12, 1184–1192 CrossRef CAS PubMed.
- M. Haeili, C. Moore, C. J. C. Davis, J. B. Cochran, S. Shah, T. B. Shrestha, Y. Zhang, S. H. Bossmann, W. H. Benjamin, O. Kutsch and F. Wolschendorf, Antimicrob. Agents Chemother., 2014, 58, 3727–3736 CrossRef PubMed.
- A. Speer, T. B. Shrestha, S. H. Bossmann, R. J. Basaraba, G. J. Harber, S. M. Michalek, M. Niederweis, O. Kutsch and F. Wolschendorf, Antimicrob. Agents Chemother., 2013, 57, 1089–1091 CrossRef CAS PubMed.
- K. Y. Djoko, M. M. Goytia, P. S. Donnelly, M. A. Schembri, W. M. Shafer and A. G. McEwan, Antimicrob. Agents Chemother., 2015, 59, 6444–6453 CrossRef CAS PubMed.
- J. Northcote-Smith, P. Kaur and K. Suntharalingam, Eur. J. Inorg. Chem., 2021, 2021, 1770–1775 CrossRef CAS.
- A. Eskandari, J. N. Boodram, P. B. Cressey, C. Lu, P. M. Bruno, M. T. Hemann and K. Suntharalingam, Dalton Trans., 2016, 45, 17867–17873 RSC.
- P. A. Turley, R. J. Fenn and J. C. Ritter, Biofouling, 2000, 15, 175–182 CrossRef CAS PubMed.
- N. L. Reeder, J. Kaplan, J. Xu, R. S. Youngquist, J. Wallace, P. Hu, K. D. Juhlin, J. R. Schwartz, R. A. Grant, A. Fieno, S. Nemeth, T. Reichling, J. P. Tiesman, T. Mills, M. Steinke, S. L. Wang and C. W. Saunders, Antimicrob. Agents Chemother., 2011, 55, 5753–5760 CrossRef CAS PubMed.
- M. Wehbe, C. Lo, A. W. Y. Leung, W. H. Dragowska, G. M. Ryan and M. B. Bally, Invest. New Drugs, 2017, 35, 682–690 CrossRef CAS PubMed.
- J. Kljun, M. Anko, K. Traven, M. Sinreih, R. Pavlič, Š. Peršič, Ž. Ude, E. E. Codina, J. Stojan, T. L. Rižner and I. Turel, Dalton Trans., 2016, 45, 11791–11800 RSC.
- J. Kladnik, J. Kljun, H. Burmeister, I. Ott, I. Romero-Canelón and I. Turel, Chem. – Eur. J., 2019, 25, 14169–14182 CrossRef CAS PubMed.
-
D. A. Farmer, US Pat., 5238490, 1993 Search PubMed.
- D. Magda, P. Lecane, Z. Wang, W. Hu, P. Thiemann, X. Ma, P. K. Dranchak, X. Wang, V. Lynch, W. Wei, V. Csokai, J. G. Hacia and J. L. Sessler, Cancer Res., 2008, 68, 5318–5325 CrossRef CAS PubMed.
- J. M. Cross, N. Gallagher, J. H. Gill, M. Jain, A. W. McNeillis, K. L. Rockley, F. H. Tscherny, N. J. Wirszycz, D. S. Yufit and J. W. Walton, Dalton Trans., 2016, 45, 12807–12813 RSC.
- A. D. Bond, N. Feeder, S. J. Teat and W. Jones, Acta Crystallogr., Sect. C: Cryst. Struct. Commun., 2001, 57, 1157–1158 CrossRef CAS PubMed.
- J. M. Cross, T. R. Blower, A. D. H. Kingdon, R. Pal, D. M. Picton and J. W. Walton, Molecules, 2020, 25, 1–8 CrossRef PubMed.
- R. Paprocka, M. Wiese-Szadkowska, S. Janciauskiene, T. Kosmalski, M. Kulik and A. Helmin-Basa, Coord. Chem. Rev., 2022, 452, 214307 CrossRef CAS.
- B. Sergi, I. Bulut, Y. Xia, Z. A. E. Waller, Y. Yildizhan, C. Acilan and R. M. Lord, ChemMedChem, 2021, 16, 2402–2410 CrossRef CAS PubMed.
|
This journal is © The Royal Society of Chemistry 2023 |