DOI:
10.1039/D3NR02012F
(Paper)
Nanoscale, 2023,
15, 15396-15404
Polymeric architecture as a tool for controlling the reactivity of palladium(II) loaded nanoreactors†
Received
1st May 2023
, Accepted 20th August 2023
First published on 24th August 2023
Abstract
Self-assembled systems, like polymeric micelles, have become great facilitators for conducting organic reactions in aqueous media due to their broad potential applications in green chemistry and biomedical applications. Massive strides have been taken to improve the reaction scope of such systems, enabling them to perform bioorthogonal reactions for prodrug therapy. Considering these significant advancements, we sought to study the relationships between the architecture of the amphiphiles and the reactivity of their PdII loaded micellar nanoreactors in conducting depropargylation reactions. Towards this goal, we designed and synthesized a series of isomeric polyethylene glycol (PEG)-dendron amphiphiles with different dendritic architectures but with an identical degree of hydrophobicity and hydrophilic to lipophilic balance (HLB). We observed that the dendritic architecture, which serves as the main binding site for the PdII ions, has greater influence on the reactivity than the hydrophobicity of the dendron. These trends remained constant for two different propargyl caged substrates, validating the obtained results. Density functional theory (DFT) calculations of simplified models of the dendritic blocks revealed the different binding modes of the various dendritic architectures to PdII ions, which could explain the observed differences in the reactivity of the nanoreactors with different dendritic architectures. Our results demonstrate how tuning the internal architecture of the amphiphiles by changing the orientation of the chelating moieties can be used as a tool for controlling the reactivity of PdII loaded nanoreactors.
1. Introduction
Despite the vital role of water as a solvent in nature in general and especially in living systems, it did not make its way into mainstream organic chemistry. Even today, most organic synthesis is carried out in dry organic solvents since many of the chemicals and reagents used are sparingly soluble in water. Amphiphilic polymers that self-assemble in water, where the hydrophobic portions collapse together because of the hydrophobic effect, can help bridge this gap between organic and aqueous phase chemistry.1 Polymeric assemblies, including micelles and vesicles, have gained increasing attention due to their potential to serve as nanoreactors for conducting organic reactions in aqueous media.2 These self-assembled structures have sizes in the nanometre range. They can host most hydrophobic substrates, effectively reducing the reaction volume and leading to a high local concentration of the substrate molecules. This phenomenon can eventually help in carrying out reactions in relatively milder conditions than conducting the corresponding reaction in an organic environment.3–5 Additional advantages of utilizing these systems include the reduced consumption of volatile organic solvents and reduced chemical load on the environment.
Although the development of nanoreactors is in its emergent state, significant contributions have already been made, especially in facilitating organometallic and organocatalytic reactions using self-assembled polymeric amphiphiles as reported by Meijer,6–8 Lipshutz,5,9–11 Weck,12–14 O'Reilly,15–18 Zimmerman,19–21 and many others.22–26 Several studies have focused on understanding the effect of different ligands,6–8,19,24,27 amphiphile's and substrate's hydrophobicity,7,26 and the type of co-surfactants,9,10 on the range and the type of the reactions that can be conducted in aqueous environment. In addition to these parameters, it is also important to further understand how the architecture of the amphiphiles and the resulting positioning and number of chelating moieties can affect the reactivity of the self-assembled nanoreactors. Hence, we decided to systematically tune the position of the chelating moieties and the number of end-groups in the dendritic part of the amphiphile while maintaining their overall molecular weight and hydrophilic to lipophilic balance (HLB).10 By using this approach, we aimed to isolate the effect of the dendritic architecture on reaction kinetics while minimizing the influence of other parameters. With this in mind, we synthesized a library of PEG-dendron amphiphiles with three, four, and six thioether containing end-groups in the dendritic part of the amphiphile (Fig. 1). The utilization of a dendritic structure as the lipophilic block allowed us to precisely tune the architecture and the lipophilicity of the dendritic block. This high molecular precision enabled us to synthesize amphiphiles with nearly identical molecular weights and HLB. In this study we used palladium-mediated O-propargyl cleavage27–30 as a model reaction due to its potential application as bioorthogonal uncaging approach for the activation of dyes and prodrugs.31–35 The suggested mechanisms of this palladium catalysed uncaging of propargylic substrates such as dyes and cytotoxic drugs has been recently reviewed by Domingos.36
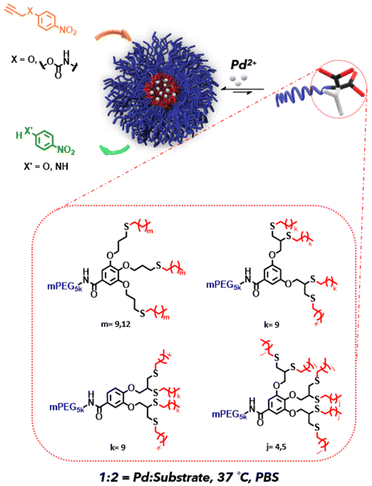 |
| Fig. 1 Schematic illustration of PdII-loaded micellar nanoreactors based on PEG-dendron amphiphiles with varied architectures for the depropargylation of substrates with different propargyl protecting groups. | |
To evaluate the impact of the type of protecting group on the reaction rate, we synthesized two low molecular weight substrates containing different propargyl protecting groups (propargyl ether and propargyl carbamate). These substrates were used as model prodrugs for conducting depropargylation reactions using PdII-loaded micellar nanoreactors with different dendritic architectures. This approach enabled us to investigate how the nano environment inside the micellar core, and the type of propargyl protecting group affect the reaction rates.
2. Results and discussion
Our amphiphiles were constructed from a commercial 5 kDa methoxy polyethylene glycol (mPEG5k) as the hydrophilic block, and dendron as the lipophilic block. The dendritic architecture, and the number and length of the end-groups were varied to maintain the overall molecular weight and HLB of the amphiphiles to help us isolate the effect of their architecture on the kinetics.
2.1. Tuning the number of end-groups in dendron
Synthesis and characterization of amphiphiles.
Three amphiphiles were synthesized: mPEG5k-D-(C14)3 (C14×3) (calculated Mn = 6081 Da), mPEG5k-D-(C11)4, (3,5) (C11×4 (3,5)) (calculated Mn = 6084 Da) and mPEG5k-D-(C6)6 (C6×6) (calculated Mn = 6094 Da). The C14×3 amphiphile was synthesized by coupling mPEG5k-NH2 to an activated 4-nitrophenol ester of 3,4,5-tris(allyloxy)benzoic acid37 to get mPEG5k-triene followed by a thiol–ene reaction using 1-tetradecanethiol to obtain the amphiphile. For making the four-armed (3,5) amphiphile, C11×4 (3,5), mPEG5k-NH2 was coupled with an activated 4-nitrophenol ester of 3,5-bis(prop-2-yn-1-yloxy) benzoic acid37 to get mPEG5k-di-yne (3,5), followed by thiol–yne reaction with 1-undecanethiol. To make the C6×6 amphiphile, first the complete C6×6 dendron was synthesized by propargylating gallic acid,37 which was then coupled to 1-hexanethiol using thiol–yne chemistry. Finally, the C6×6 dendron was conjugated to mPEG5k-NH2 to get the final amphiphile (Scheme 1). 1H-NMR, High-Performance Liquid Chromatography (HPLC), and Gel Permeation Chromatography (GPC) were used to verify the conversion, product's purity, molecular weight and polydispersity, respectively. All experimental results showed excellent correlations with the expected values, as can be seen in the ESI.†
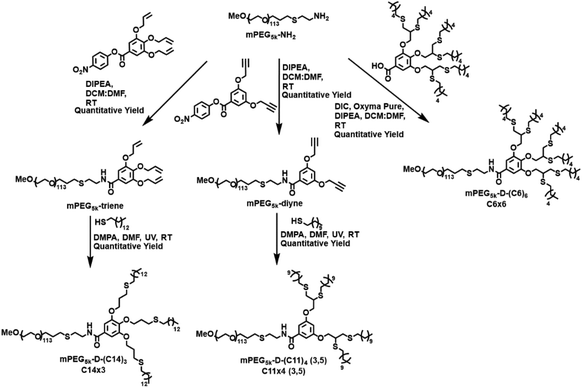 |
| Scheme 1 Synthetic route for C14×3, C11×4 (3,5) and C6×6 amphiphiles. | |
After synthesizing the amphiphiles, their self-assembly into micelles in aqueous media (phosphate buffered saline-PBS, pH 7.4, 37 °C) was examined by measuring their critical micelle concentration (CMC) values using the Nile red method.38 The CMC values of all three amphiphiles were nearly identical (Table 1 and Fig. S20, S21 and S23†). Next, we used dynamic light scattering (DLS) to estimate the size of the micelles in the presence and absence of PdII salt. To prepare the metal loaded micelles, the PdII salt and the different amphiphiles were dissolved separately in acetone, mixed and stirred briefly, followed by the evaporation of acetone to form a thin film, which was rehydrated in PBS to yield aqueous micellar solutions ([amphiphile] = 320 μM). The diameters of the micelles measured by DLS were found to be around 20 nm (for the empty micelles), while slightly smaller diameters were observed for PdII-loaded micelles (Table 1 and Fig. 2). Transmission electron microscopy (TEM) images of empty, and PdII loaded micelles further confirmed the presence of nano-sized spherical particles (Fig. 2 and Fig. S31†), which corelates with the data that was obtained from DLS.
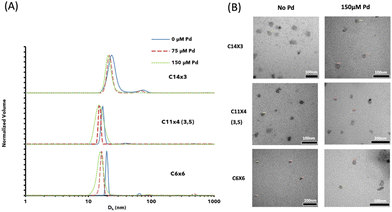 |
| Fig. 2 (A) DLS measurements of micelles with different dendritic architectures ([amphiphile] = 320 μM) without encapsulated Pd(OAc)2 (blue solid lines), micelles with encapsulated PdII ([Pd(OAc)2] = 75 μM) (red large-dashed lines) and micelles with encapsulated PdII ([Pd(OAc)2] = 150 μM) (green dashed lines) in PBS (pH = 7.4) at 37 °C. (B) TEM images of the micelles based on the C14×3, C11×4 (3,5) and C6×6 amphiphiles, with (right column) and without (left column) Pd(OAc)2 salt. | |
Table 1 Molecular properties of the C14×3, C11×4 (3,5) and C6×6 amphiphiles and their micellar assemblies
Hybrid |
Name |
End-group |
M
n a (kDa) |
M
n b (kDa) |
Đ
|
CMCc (μM) |
D
h d (nm) |
D
h e (nm) |
D
h f (nm) |
[Pd] = 0 μM |
[Pd] = 75 μM |
[Pd] = 150 μM |
Calculated based on a 5 kDa mPEG and the expected exact mass of the dendrons.
Measured by GPC using PEG commercial standards.
Determined using the Nile red method.
Hydrodynamic diameter measured by DLS (% volume) of micelles formed from micelles only ([amphiphile] = 320 μM).
Hydrodynamic diameter measured by DLS (% volume) of micelles ([amphiphile] = 320 μM) with the encapsulated Pd(OAc)2 salt [75 μM].
Hydrodynamic diameter measured by DLS (% volume) of micelles ([amphiphile] = 320 μM) with the encapsulated Pd(OAc)2 salt [150 μM].
|
mPEG5k-D-(C14)3 |
C14×3
|
n-C14H29 |
6.1 |
6.3 |
1.03 |
4 ± 1 |
24 ± 4 |
22 ± 2 |
20 ± 7 |
mPEG5k-D-(C11)4 (3,5) |
C11×4 (3,5)
|
n-C11H23 |
6.1 |
6.3 |
1.04 |
4 ± 1 |
17 ± 1 |
15 ± 1 |
15 ± 2 |
mPEG5k-D-(C6)6 |
C6×6
|
n-C6H13 |
6.1 |
6.0 |
1.04 |
3 ± 1 |
20 ± 1 |
16 ± 1 |
16 ± 2 |
The two substrates para-nitrophenyl propargyl ether (PNPPE)26 and N-para-nitrophenyl O-propargyl carbamate (PNACAPE)39 with propargyl ether and propargyl carbamate protecting groups, respectively, were synthesized as previously reported. The depropargylation reactions were conducted by adding the substrate, PNPPE (300 μM) and PNACAPE (150 μM) to micellar solutions containing 150 μM and 75 μM PdII respectively. The ratio of PdII to substrate was 1
:
2 in both cases. The reaction progress at 37 °C was monitored by HPLC (Fig. 3, and Fig. S34–S37, S40 and S41†).
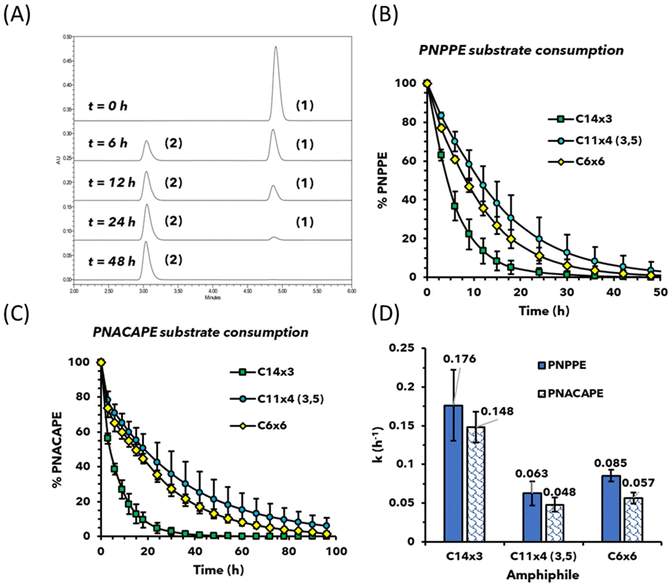 |
| Fig. 3 (A) Representative HPLC chromatogram overlay (taken at 307 nm), showing the transformation of PNPPE (1) to para-nitrophenol (PNP) (2) using PdII loaded C14×3 amphiphilic micelles ([Amphiphile] = 320 μM, [PNPPE] = 300 μM, [Pd(OAc)2] = 150 μM, [Pd(OAc)2 : Substrate] = 1 : 2). Normalized substrate consumption of (B) PNPPE and (C) PNACAPE over time in the presence of C14×3 (square, green), C11×4 (3,5) (circle, deep sky blue) and C6×6 (diamond, yellow) metallic micelles. (D) Rate constants of PNPPE (blue, solid fill) and PNACAPE (blue, shingle pattern) depropargylation in presence of C14×3, C11×4 (3,5) and C6×6 metal loaded micelles. | |
To evaluate the rate of depropargylation, the area under the curve (AUC) of the substrate's peak in each chromatogram was measured (Fig. 3A) and the decrease in AUC (in %) was plotted as a function of time (Fig. 3B and C). To assess the reaction kinetics, a natural log of the experimental data was plotted against time which provided a linear equation correlating with a pseudo first-order reaction ln[A] = −kt + ln[A]0. The rate constant (k) values were calculated from the slope of the kinetic data, and the theoretical half-life (t1/2) values were calculated using the equation t1/2 = ln(2)/k. The rate constant k and calculated values of t1/2 are presented alongside the experimental value of t1/2 in Table S1.†
The kinetic results showed that micelles made with C14×3 amphiphile were two and a half times faster in performing depropargylation than micelles made from C6×6 and C11×4 (3,5) amphiphiles, while the C11×4 (3,5) being the slowest of the three (Fig. 3B and C). The difference in depropargylation rates between C6×6 and C11×4 (3,5) amphiphiles was small compared to C14×3 (Fig. 3D).
Importantly, the kinetic trends among the three amphiphiles remained the same for both PNPPE and PNACAPE substrates, although the depropargylation of the latter was slower for all three micellar nanoreactors.
ICP-MS measurements were conducted to ensure that all the micellar nanoreactors had similar PdII concentrations for a particular substrate and that the obtained trends resulted solely from the architectural changes in the amphiphiles (Fig. S45 and S46†).
2.2. The effect of the length of the end-group on the kinetics of depropargylation
Next, we aimed to investigate if the number of carbon atoms in the end-groups affected the kinetic results. Towards this, mPEG5k-D-(C7)6, i.e., C7×6, was synthesized and compared with C14×3 to assess their ability to conduct the depropargylation reactions, since both C7×6 and C14×3 amphiphiles have a total of forty-two carbon atoms in their end-groups. The C7×6 amphiphile was synthesized using the same method employed for the C6×6 amphiphile by preparing the dendron followed by coupling it to mPEG5k-NH2 (Fig. S10†). As seen in Tables 1 and 2, C7×6 and C14×3 based micelles had nearly identical CMCs and diameters (4 μM and ∼20 nm respectively).
Table 2 Molecular properties of the C7×6, C11×3, and C11×4 (3,4) amphiphiles and their micellar assemblies
Hybrid |
Name |
End-group |
M
n a (kDa) |
M
n b (kDa) |
Đ
|
CMCc (μM) |
D
h d (nm) |
D
h e (nm) |
D
h f (nm) |
[Pd] = 0 μM |
[Pd] = 75 μM |
[Pd] = 150 μM |
Molecular weight calculated based on a 5 kDa mPEG and the expected exact mass of the dendrons.
Molecular weight measured by GPC using PEG commercial standards.
Determined using the Nile red method.
Hydrodynamic diameter measured by DLS (% volume) of micelles formed from micelles only ([amphiphile] = 320 μM).
Hydrodynamic diameter measured by DLS (% volume) of micelles ([amphiphile] = 320 μM) with the encapsulated Pd(OAc)2 salt [75 μM].
Hydrodynamic diameter measured by DLS (% volume) of micelles ([amphiphile] = 320 μM) with the encapsulated Pd(OAc)2 salt [150 μM].
|
mPEG5k-D-(C7)6 |
C7×6
|
n-C7H15 |
6.2 |
5.7 |
1.07 |
4 ± 1 |
21 ± 2 |
20 ± 1 |
19 ± 3 |
mPEG5k-D-(C11)3 |
C11×3
|
n-C11H23 |
6.0 |
5.7 |
1.04 |
5 ± 1 |
15 ± 1 |
15 ± 1 |
15 ± 1 |
mPEG5k-D-(C11)4 (3,4) |
C11×4 (3,4)
|
n-C11H23 |
6.1 |
6.6 |
1.12 |
6 ± 1 |
20 ± 1 |
20 ± 1 |
18 ± 2 |
Despite having the same number of carbon atoms in the end-groups, C14×3 based micelles were almost twice as fast as compared to C7×6 based micelles at depropargylating both the substrates (Fig. 4). Additionally, the C7×6 based micelles were approximately 10–15% faster than micelles of C6×6. This result is in good correlation with our previous work, which showed that increase in the length of the end-group for a particular architecture leads to faster depropargylation.26 This highlighted that the architecture had greater influence on the kinetics than the length of the end-groups and the hydrophobicity of the dendron.
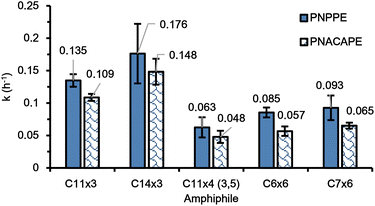 |
| Fig. 4 Rate constants of PNPPE (blue, solid fill) and PNACAPE (blue, shingle pattern) depropargylation in presence of C11×3, C14×3, C11×4 (3,5), C6×6 and C7×6 metallic micelles. | |
Following this comparison, we synthesized an additional amphiphile, mPEG5k-D-(C11)3 (C11×3), and conducted depropargylation experiments using its micelles, which were then compared with the C11×4 (3,5) based micelles. The C11×3 amphiphile was synthesized using the same method employed for making the C14×3 amphiphile, using a thiol–ene coupling reaction of mPEG5k-triene with 1-undecanethiol to obtain the final amphiphile (Fig. S1†). As seen Fig. 4, it was observed that C11×3 based micelles were twice as fast as C11×4 (3,5) in depropargylating both the substrates. The overall rates obtained for C11×3 were closer to C14×3 than to C11×4 (3,5), reinforcing the hypothesis that the architecture had a greater influence on the observed kinetics than the length of the end-groups. In this case as well, the depropargylation rates of C11×3 based micelles were approximately 25% slower than those obtained for C14×3 based micelles, which aligns well with our current and previous findings on the contribution of end-group hydrophobicity for the same polymeric architecture.26
2.3. Changing the position of the end-groups
To understand the influence of the position of the branches of the dendron on the kinetics, a different isomer of the four-armed amphiphile was synthesized with the 1,2 dimercaptoether containing end-groups in the third and the fourth position, yielding the mPEG5k-D-(C11)4 (3,4) amphiphile, i.e., C11×4 (3,4). The synthetic procedure was similar to the C6×6 amphiphile where the C11×4 (3,4) dendron was first synthesized and then subsequently coupled to mPEG5k-NH2 to obtain the final C11×4 (3,4) amphiphile (Fig. S6†). As observed in Fig. 5, the C11×4 (3,4) based micelles exhibited the fastest kinetics among all the polymers studied. This result was unexpected as the C6×6 amphiphile, has two of its three 1,2 dimercaptoether moieties located at the third and the fourth position of the aromatic branching unit, similar in their substitution pattern to the two 1,2 dimercaptoether groups of the C11×4 (3,4).
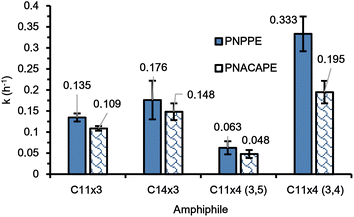 |
| Fig. 5 Rate constants of PNPPE (blue, solid fill) and PNACAPE (blue, shingle pattern) depropargylation in presence of C11×3, C14×3, C11×4 (3,5) and C11×4 (3,4) metallic micelles. | |
2.4. Anisotropy measurements
To understand the reason for the observed trends in kinetic rates, we aimed to investigate whether the difference in mobility of guest molecules inside the micelles can be correlated with the difference in depropargylation rates for amphiphiles with different architectures. The mobility of a fluorescent molecule inside the micelles could provide a proxy for the freedom of movement of a substrate molecule inside the core thus ultimately affecting the rates of depropargylation. To calculate the anisotropy factors, we encapsulated Nile Red dye ([Dye] = 21 nM, [amphiphile] = 320 μM), along with various concentrations of Pd(OAc)2 ([Pd] = 0, 75, 150 μM). The measurements were conducted by placing light polarizers at the source and the detector in different combinations of vertical and horizontal orientations (section 4.3 in ESI†).40
The results showed an increase in the anisotropy factor with increasing concentration of Pd(OAc)2 but the value remained the same for all micelles at a given concentration of Pd(OAc)2 with a slight variation of around 5% (Fig. 6). Thus, it was concluded that the Nile Red had approximately the same mobility in the core of the micelles irrespective of their amphiphile's architecture, suggesting that mobility inside the core is not the influence behind the observed trends in the depropargylation kinetics.
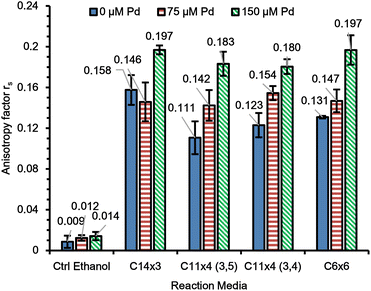 |
| Fig. 6 Anisotropy factor of Nile red dye in amphiphilic micellar environment ([Amphiphile] = 320 μM) at different concentrations of encapsulated palladium acetate. [PdII] = 0 μM (blue, solid fill), 75 μM (orange, horizontal stripes), 150 μM (green, downward stripes). | |
2.5. DFT computed binding modes of thioethers/PdII complexes in different dendrons
To better understand the observed kinetic trends, DFT calculations were carried out using the BP86-D3 method,41,42 with Ahlrichs’ def2-SVP basis set,43 and with the relativistic effect of palladium that was accounted for by the Stuttgart–Dresden (SDD) effective core potential (ECP).44,45 Before DFT measurements, we simplified our ligand/PdII structures based on the results from our previous NMR titration experiments with PEG-dendron amphiphile (3,5-based architecture) and Pd(OAC)2, which showed that only protons adjacent to the sulphur, oxygen, and the aromatic ring, got affected when coordinating with the PdII salt.26 Therefore, for these model systems, the structure of the amphiphile was simplified by replacing the PEG chain and dendritic aliphatic end-groups with methyl groups, while preserving the exact architecture of the dendritic cores (Fig. 7). Subsequently, we optimized the geometries of the four model systems and the resulting geometry around PdII was analysed for each system.
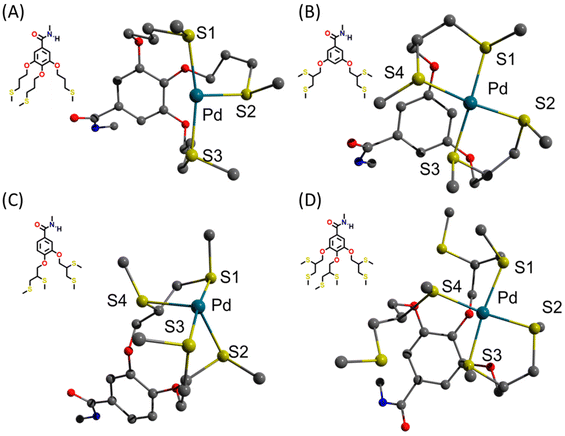 |
| Fig. 7 Simulated results of the predicted orientation of thioethers around the palladium atom (green) in the dendritic structures of (A) three-armed architecture, (B) four-armed (3,5) architecture, (C) four-armed (3,4) architecture and (D) six-armed architecture. | |
The optimized structure of the PdII-complex with the three-armed dendron (Fig. 7A) exhibits a T-shaped geometry of the PdII-center with vacant fourth position that could potentially serve to bind the substrate to the palladium center. The optimized structure of the PdII-complex with the four-armed (3,4) dendron has a seesaw geometry around PdII-center, which is atypical and unfavoured in the case of PdII ion (Fig. 7C). The optimized structures of the PdII-complex with the four-armed (3,5) and six-armed dendrons both have a square planar geometry, which typical for PdII-complexes (Fig. 7B and D).46,47
The calculated results (Fig. 7) provided important insights into the different reactivities that were observed in the kinetic experiments (Fig. 4 and Fig. 5). While nanoreactors made from four-armed (3,5) and the six-armed dendritic amphiphiles were slowest among the four studied systems, both architectures were shown to have square planar geometry architectures around the PdII-center, which is a more stable geometry for the PdII-complex.
On the other hand, the dendritic cores of the other two amphiphiles, which were significantly more reactive, showed atypical seesaw geometry (for the four-armed (3,4) architecture) and a T-shaped geometry (for the three-arm architecture). These unique geometries can be expected to be less stable and hence allows for a better substrate binding, which enables increased reactivity. Overall, the calculated geometries could provide an explanation for the different reactivates observed for the four systems, indicating that the more stable the chelating of the PdII ions, the less reactive the nanoreactors become. Nevertheless, it is important to note that despite the different geometries, all amphiphiles were shown to maintain the same amount of PdII ions, demonstrating the critical role of the dendritic core's architecture on the reactivity of the micellar nanoreactors.
3. Conclusions
This work was aimed at understanding the influence of the hydrophobic dendritic core's architecture on PdII-mediated depropargylation reaction in micellar nanoreactors. Our micellar nanoreactors were based on self-assembled PEG-dendron amphiphiles with palladium acetate as the catalytic species. The ability to synthesize the dendritic block with a high precision enabled us to construct PEG dendron amphiphiles with different dendritic architectures while controlling their HLB and overall molecular weight by changing the number, position and length of the end-groups. These nanoreactors were used to perform depropargylation reactions in PBS with two different propargyl caged substrates, which could potentially be replaced by a propargyl caged prodrug in the future.
The obtained results highlighted how changing the dendritic architecture of the amphiphiles has a greater influence on the depropargylation kinetics than the number and length of the end-groups. In our observations, the micelles based on C11×4 (3,4) exhibited the fastest kinetics, surpassing the speed of C11×4 (3,5)-based micelles by four to five times. Remarkably, this significantly greater efficiency was observed despite both amphiphiles being isomers with identical atomic composition. The reason for such a drastic difference in the reactivity of micelles with different architectures was elucidated with the help of DFT calculations of the dendritic core of the amphiphile around the PdII ion. These calculations suggested that CX×4 (3,4) architecture should have a highly unlikely seesaw geometry, while the three-armed architecture showed less stable T-shape geometry for their PdII complexes. These unusual geometries, in contrast to the square planar geometry obtained for the CX×4 (3,5) and six-arm amphiphiles, are predicted to be more labile. As a result, they allow PdII to participate more actively in the depropargylation process compared to the other architectures.
This study highlights how subtle changes in the polymeric architecture can potentially lead to enormous changes in the reaction kinetics for conducting abiotic reactions in aqueous media. Furthermore, when designing micellar nanoreactors for biorthogonally activating prodrugs, we believe these results can serve as a valuable tool for controlling the prodrug activation rate, allowing it to be customized according to the medical requirements of patients.
Author contributions
SW and RJA conceived and designed the project. SW and PR synthesized the amphiphiles and performed the depropargylation experiments. KV helped with performing depropargylation experiments. ST synthesized the PNPPE substrate. RD performed the DFT calculations. AG performed the ICP-MS characterizations. All authors contributed to the interpretation of results and experimental design. SW wrote the manuscript, and all authors revised the final version.
Conflicts of interest
There are no conflicts to declare.
Acknowledgements
This project has received funding from the European Union's Horizon 2020 research and innovation programme under the Marie Sklodowska-Curie grant agreement no. 765497 (THERACAT). S. T. thanks the ADAMA Center for Novel Delivery Systems in Crop Protection, Tel-Aviv University, for the financial support. ICP-MS analysis was conducted at the ADAMA Center for Novel Delivery Systems in Crop Protection, Tel-Aviv University. The background image for the graphical abstract by Daniel Sinoca on Unsplash.
References
- M. Cortes-Clerget, J. Yu, J. R. A. Kincaid, P. Walde, F. Gallou and B. H. Lipshutz, Water as the reaction medium in organic chemistry: from our worst enemy to our best friend, Chem. Sci., 2021, 12, 4237–4266 RSC
.
- B. S. Takale, R. R. Thakore, G. Casotti, X. Li, F. Gallou and B. H. Lipshutz, Mild and Robust Stille Reactions in Water using Parts Per Million Levels of a Triphenylphosphine-Based Palladacycle, Angew. Chem., Int. Ed., 2021, 60, 4158–4163 CrossRef CAS PubMed
.
- J. Epstein, J. J. Kaminski, N. Bodor, R. Enever, J. Sowa and T. Higuchi, Micellar acceleration of organophosphate hydrolysis by hydroximinomethylpyridinium type surfactants, J. Org. Chem., 1978, 43, 2816–2821 CrossRef CAS
.
- F. Gallou, N. A. Isley, A. Ganic, U. Onken and M. Parmentier, Surfactant technology applied toward an active pharmaceutical ingredient: more than a simple green chemistry advance, Green Chem., 2016, 18, 14–19 RSC
.
- B. H. Lipshutz, The Nano-to-Nano Effect Applied to Organic Synthesis in Water, Johnson Matthey Technol. Rev., 2017, 61, 196–202 CrossRef CAS
.
- Y. Liu, P. Turunen, B. F. M. De Waal, K. G. Blank, A. E. Rowan, A. R. A. Palmans and E. W. Meijer, Catalytic single-chain polymeric nanoparticles at work: From ensemble towards single-particle kinetics, Mol. Syst. Des. Eng., 2018, 3, 609–618 RSC
.
- M. Artar, E. R. J. Souren, T. Terashima, E. W. Meijer and A. R. A. Palmans, Single Chain Polymeric Nanoparticles as Selective Hydrophobic Reaction Spaces in Water, ACS Macro Lett., 2015, 4, 1099–1103 CrossRef CAS
.
- Y. Liu, S. Pujals, P. J. M. Stals, T. Paulöhrl, S. I. Presolski, E. W. Meijer, L. Albertazzi and A. R. A. Palmans, Catalytically Active Single-Chain Polymeric Nanoparticles: Exploring Their Functions in Complex Biological Media, J. Am. Chem. Soc., 2018, 140, 3423–3433 CrossRef CAS PubMed
.
- B. H. Lipshutz, S. Ghorai, A. R. Abela, R. Moser, T. Nishikata, C. Duplais, A. Krasovskiy, R. D. Gaston and R. C. Gadwood, TPGS-750-M: A second-generation amphiphile for metal-catalyzed cross-couplings in water at room temperature, J. Org. Chem., 2011, 76, 4379–4391 CrossRef CAS PubMed
.
- B. H. Lipshutz, G. T. Aguinaldo, S. Ghorai and K. Voigtritter, Olefin Cross-Metathesis Reactions at Room Temperature Using the Nonionic Amphiphile “PTS”: Just Add Water, Org. Lett., 2008, 10, 1325–1328 CrossRef CAS PubMed
.
- M. Cortes-Clerget, N. Akporji, J. Zhou, F. Gao, P. Guo, M. Parmentier, F. Gallou, J. Y. Berthon and B. H. Lipshutz, Bridging the gap between transition metal- and bio-catalysis via aqueous micellar catalysis, Nat. Commun., 2019, 10, 1–10 CrossRef CAS
.
- P. Qu, M. Kuepfert, E. Ahmed, F. Liu and M. Weck, Cross-Linked Polymeric Micelles as Catalytic Nanoreactors, Eur. J. Inorg. Chem., 2021, 2021, 1420–1427 CrossRef CAS
.
- J. Lu, J. Dimroth and M. Weck, Compartmentalization of Incompatible Catalytic Transformations for Tandem Catalysis, J. Am. Chem. Soc., 2015, 137, 12984–12989 CrossRef CAS PubMed
.
- M. Kuepfert, E. Ahmed and M. Weck, Self-Assembled Thermoresponsive Molecular Brushes as Nanoreactors for Asymmetric Aldol Addition in Water, Macromolecules, 2021, 54, 3845–3853 CrossRef CAS
.
- P. Cotanda, A. Lu, J. P. Patterson, N. Petzetakis and R. K. O'Reilly, Functionalized Organocatalytic Nanoreactors: Hydrophobic Pockets for Acylation Reactions in Water, Macromolecules, 2012, 45, 2377–2384 CrossRef CAS
.
- A. Lu, T. P. Smart, I. Thomas, H. Epps, D. A. Longbottom and R. K. O'Reilly, l-Proline Functionalized Polymers Prepared by RAFT Polymerization and Their Assemblies as Supported Organocatalysts, Macromolecules, 2011, 44, 7233–7241 CrossRef CAS PubMed
.
- E. Lestini, L. D. Blackman, C. M. Zammit, T. Chen, R. J. Williams, M. Inam, B. Couturaud and R. K. O'Reilly, Palladium-polymer nanoreactors for the aqueous asymmetric synthesis of therapeutic flavonoids, Polym. Chem., 2018, 9, 820–823 RSC
.
- P. Cotanda, N. Petzetakis and R. K. O'Reilly, Catalytic polymeric nanoreactors: More than a solid supported catalyst, MRS Commun., 2012, 2, 119–126 CrossRef CAS
.
- J. Chen, J. Wang, K. Li, Y. Wang, M. Gruebele, A. L. Ferguson and S. C. Zimmerman, Polymeric ‘clickase’ Accelerates the Copper Click Reaction of Small Molecules, Proteins, and Cells, J. Am. Chem. Soc., 2019, 141, 9693–9700 CrossRef CAS PubMed
.
- J. Chen, J. Wang, Y. Bai, K. Li, E. S. Garcia, A. L. Ferguson and S. C. Zimmerman, Enzyme-like Click Catalysis by a Copper-Containing Single-Chain Nanoparticle, J. Am. Chem. Soc., 2018, 140, 13695–13702 CrossRef CAS PubMed
.
- Y. Bai, J. Chen and S. C. Zimmerman, Designed transition metal catalysts for intracellular organic synthesis, Chem. Soc. Rev., 2018, 47, 1811–1821 RSC
.
- T. N. Ansari, A. Taussat, A. H. Clark, M. Nachtegaal, S. Plummer, F. Gallou and S. Handa, Insights on Bimetallic Micellar Nanocatalysis for Buchwald–Hartwig Aminations, ACS Catal., 2019, 9, 10389–10397 CrossRef CAS
.
- M. Bihani, T. N. Ansari, L. Finck, P. P. Bora, J. B. Jasinski, B. Pavuluri, D. K. Leahy and S. Handa, Scalable α-Arylation of Nitriles in Aqueous Micelles using Ultrasmall Pd Nanoparticles: Surprising Formation of Carbanions in Water, ACS Catal., 2020, 10, 6816–6821 CrossRef CAS
.
- C. Vidal, M. Tomás-Gamasa, P. Destito, F. López and J. L. Mascareñas, Concurrent and orthogonal gold(I) and ruthenium(II) catalysis inside living cells, Nat. Commun., 2018, 9, 1913 CrossRef PubMed
.
- P. Destito, A. Sousa-Castillo, J. R. Couceiro, F. López, M. A. Correa-Duarte and J. L. Mascareñas, Hollow nanoreactors for Pd-catalyzed Suzuki-Miyaura coupling and O-propargyl cleavage reactions in bio-relevant aqueous media, Chem. Sci., 2019, 10, 2598–2603 RSC
.
- S. Tevet, S. S. Wagle, G. Slor and R. J. Amir, Tuning the Reactivity of Micellar Nanoreactors by Precise Adjustments of the Amphiphile and Substrate Hydrophobicity, Macromolecules, 2021, 54, 11419–11426 CrossRef CAS PubMed
.
- A. Sathyan, S. Croke, A. M. Pérez-López, B. F. M. de Waal, A. Unciti-Broceta and A. R. A. Palmans, Developing Pd(II) based amphiphilic polymeric nanoparticles for pro-drug activation in complex media, Mol. Syst. Des. Eng., 2022, 7, 1736–1748 RSC
.
- M. Pal, K. Parasuraman and K. R. Yeleswarapu, Palladium-catalyzed cleavage of O/N-propargyl protecting groups in aqueous media under a copper-free condition, Org. Lett., 2003, 5, 349–352 CrossRef CAS PubMed
.
- D. Rambabu, S. Bhavani, N. K. Swamy, M. V. Basaveswara Rao and M. Pal, Pd/C-mediated depropargylation of propargyl ethers/amines in water, Tetrahedron Lett., 2013, 54, 1169–1173 CrossRef CAS
.
- S. E. Coelho, F. S. S. Schneider, D. C. De Oliveira, G. L. Tripodi, M. N. Eberlin, G. F. Caramori, B. De Souza and J. B. Domingos, Mechanism of Palladium(II)-Mediated Uncaging Reactions of Propargylic Substrates, ACS Catal., 2019, 9, 3792–3799 CrossRef CAS
.
- R. Huang, C. H. Li, R. Cao-Milán, L. D. He, J. M. Makabenta, X. Zhang, E. Yu and V. M. Rotello, Polymer-Based Bioorthogonal Nanocatalysts for the Treatment of Bacterial Biofilms, J. Am. Chem. Soc., 2020, 142, 10723–10729 CrossRef CAS PubMed
.
- J. T. Weiss, J. C. Dawson, K. G. Macleod, W. Rybski, C. Fraser, C. Torres-Sánchez, E. E. Patton, M. Bradley, N. O. Carragher and A. Unciti-Broceta, Extracellular palladium-catalysed dealkylation of 5-fluoro-1-propargyl-uracil as a bioorthogonally activated prodrug approach, Nat. Commun., 2014, 5, 3277 CrossRef PubMed
.
- R. M. Yusop, A. Unciti-Broceta, E. M. V. Johansson, R. M. Sánchez-Martín and M. Bradley, Palladium-mediated intracellular chemistry, Nat. Chem., 2011, 3, 239–243 CrossRef CAS PubMed
.
- A. Unciti-Broceta, E. M. V. Johansson, R. M. Yusop, R. M. Sánchez-Martín and M. Bradley, Synthesis of polystyrene microspheres and functionalization with pd0 nanoparticles to perform bioorthogonal organometallic chemistry in living cells, Nat. Protoc., 2012, 7, 1207–1218 CrossRef CAS PubMed
.
- T.-C. Chang, K. Vong, T. Yamamoto and K. Tanaka, Prodrug Activation by Gold Artificial Metalloenzyme-Catalyzed Synthesis of Phenanthridinium Derivatives via Hydroamination, Angew. Chem., Int. Ed., 2021, 60, 12446–12454 CrossRef CAS PubMed
.
- E. Latocheski, G. M. Dal Forno, T. M. Ferreira, B. L. Oliveira, G. J. L. Bernardes and J. B. Domingos, Mechanistic insights into transition metal-mediated bioorthogonal uncaging reactions, Chem. Soc. Rev., 2020, 49, 7710–7729 RSC
.
- A. J. Harnoy, M. Buzhor, E. Tirosh, R. Shaharabani, R. Beck and R. J. Amir, Modular Synthetic Approach for Adjusting the Disassembly Rates of Enzyme-Responsive Polymeric Micelles, Biomacromolecules, 2017, 18, 1218–1228 CrossRef CAS PubMed
.
- E. R. Gillies, T. B. Jonsson and J. M. J. Fréchet, Stimuli-Responsive Supramolecular Assemblies of Linear-dendritic Copolymers, J. Am. Chem. Soc., 2004, 126, 11936–11943 CrossRef CAS PubMed
.
- R. Ramesh, Y. Chandrasekaran, R. Megha and S. Chandrasekaran, Base catalyzed cyclization of N-aryl and N-alkyl-O-propargyl carbamates to 4-alkylidene-2-oxazolidinones, Tetrahedron, 2007, 63, 9153–9162 CrossRef CAS
.
- J. Feng, C. Lin, H. Wang and S. Liu, Gemini dodecyl O-glucoside-based vesicles as nanocarriers for catechin laurate, J. Funct. Foods, 2017, 32, 256–265 CrossRef CAS
.
- Q. S. Li, J. Zhang and S. Zhang, A DFT and ab initio direct dynamics study on the hydrogen abstract reaction of H3BNH3→H2+H2BNH2, Chem. Phys. Lett., 2005, 404, 100–106 CrossRef CAS
.
- S. Grimme, J. Antony, S. Ehrlich and H. Krieg, A consistent and accurate ab initio parametrization of density functional dispersion correction (DFT-D) for the 94 elements H-Pu, J. Chem. Phys., 2010, 132, 154104 CrossRef PubMed
.
- D. A. Pantazis and F. Neese, All-electron scalar relativistic basis sets for the 6p elements, Theor. Chem. Acc., 2012, 131, 1292 Search PubMed
.
- D. Andrae, U. Häußermann, M. Dolg, H. Stoll and H. Preuß, Energy-adjustedab initio pseudopotentials for the second and third row transition elements, Theor. Chim. Acta, 1990, 77, 123–141 CrossRef CAS
.
- M. Dolg, U. Wedig, H. Stoll and H. Preuss, Energy–adjusted ab initio pseudopotentials for the first row transition elements, J. Chem. Phys., 1987, 86, 866–872 CrossRef CAS
.
- M. C. MacInnis, R. McDonald and L. Turculet, Synthesis and Characterization of Palladium Complexes Supported by an NPN-Phosphido Ancillary Ligand, Organometallics, 2011, 30, 6408–6415 CrossRef CAS
.
- Q. Yan, L. Zheng, M. Li and Y. Chen, N,S-chelating triazole-thioether ligand for highly efficient palladium-catalyzed Suzuki reaction, J. Catal., 2019, 376, 101–105 CrossRef CAS
.
|
This journal is © The Royal Society of Chemistry 2023 |